ABSTRACT
A specific type of material, activated carbon fiber (ACF), was modified by SiO2, and the final products ACF-x were obtained as ACF-12.5, ACF-20, ACF-40, and ACF-80 according to different dosages of tetraethoxysilane (TEOS). The modified material on the ACF surface had a significant and smooth cover layer with low content of silica from scanning electron microscope (SEM) image. The modified ACF-x showed the stronger hydrophobicity, thermal stability, and adsorption capacity, which had almost no effect in the presence of water vapor and no destruction in multiple cycles. ACF-20 was proven as the most efficient adsorbent in humid conditions. The dual-function system composed of the regenerative adsorbents and the combustion catalyst would be efficient in consecutive toluene adsorption/oxidation cycles, in which the combustion catalyst was prepared by the displacement reaction of H2PtCl6 with foam Ni. Therefore, the adsorption/catalytic oxidation could be a promising technique in the indoor air purification, especially in the case of very low volatile organic compound (VOC; toluene) concentration levels.
Implications: Exploring highly effective adsorptive materials with less expensive costs becomes an urgent issue in the indoor air protection. ACF-20 modified by SiO2 with Pt/Ni catalysts shows stronger hydrophobicity, thermal stability, and adsorption capacity. This dual-function system composed of the regenerative materials and the combustion catalyst would be a promising technique in the indoor air purification, especially in the case of removal of very low concentration of toluene.
Introduction
Volatile organic compounds (VOCs) are considered as great contributors to the atmospheric pollution and dangerous for their effects on human health (Kim et al., Citation2006; Alcañiz-Monge et al., Citation2012; Zuo et al., Citation2012). Studies have been reported that hundreds of VOCs can be simultaneously found in indoor air (Thevenet et al., Citation2014; Baur et al., Citation2015a; Sharmin and Ray, Citation2012). Long-time exposure to this condition even at low concentrations could lead to the so-called “sick building syndrome” (SBS), which may have a significant impact on human health and work efficiency. Indoor air quality (IAQ) has become a significant issue since the term SBS was first coined by the World Health Organization (WHO) in 1986 (Thevenet et al., Citation2014; Nigar et al., Citation2015). Therefore, attention has been paid to monitoring indoor pollutants to improve air quality, and extensive research has been conducted to develop efficient methods for dealing with the removal of VOCs over the years (Sharmin et al., Citation2012; Xu et al., Citation2013; Raso et al., Citation2014). Recommended strategies include thermal incineration (Khan and Ghoshal, Citation2000), catalytic oxidation (Gaur et al., Citation2005; Lu et al., Citation2009; Bedia et al., Citation2010), condensation and adsorption (Kim et al., Citation2006; Lillo-Ródenas et al., Citation2006; Jo and Yang, Citation2009), biofiltration (Guieysse et al., Citation2008), and membrane separation (Chintawar and Greene, Citation1997; Baek et al., Citation2004; Liu et al., Citation2009). Among which, adsorption is a reliable chemical engineering method widely used because of flexibility of the system, easy operation, low energy, and cheap costs (Hu et al., Citation2001; Serrano et al., Citation2004; Guillemot et al., Citation2007).
Due to very low concentration of VOCs in the indoor air, the adsorption capacity is also lower for normal sorts of absorptive materials in this condition, so highly effective absorbents with less expensive operation costs become an urgent issue. Catalytic oxidation is also attractive because of low energy cost and material cost for catalyst preparation, and high removal efficiency. But in the case of lean VOC stream, catalytic oxidation alone is no more economically attractive. For this reason, a highly economical technology is proposed as follows (Yamaguchi et al., Citation2013): the polluted air initially passes through the absorptive material with large flow rate for a long time, and the polluted air is purified by means of adsorption; the adsorbed VOCs are desorbed with low flow rate in a short time by heating, then desorbed VOCs are converted into CO2 and H2O by the total catalytic oxidation process. Combined adsorption process with catalytic oxidation can be even more effective for the control of low concentration of VOCs. A number of commercially available adsorbents are used for VOC abatement, including granular activated carbon (GAC), activated carbon fiber (ACF), alumina, silica gel, zeolite, etc. Activated carbon is widely employed in adsorption process to eliminate VOCs due to its large micropore volume and low operating cost (Lee et al., Citation2004; Manjare and Ghoshal, Citation2006). Comparing with other adsorbents, VOCs can be released at a lower temperature of 160 °C over ACF under a small flow rate of the purge gas, whereas other adsorbents need a high temperature (350–400 °C) and high gas flow rate to release the VOCs (Das et al., Citation2004).
Many studies were devoted to the removal of relatively high concentration of VOCs (toluene) by adsorption on different types of activated carbon (Karimnezhad et al., Citation2014; Gupta et al., Citation2015; Zabihi et al., Citation2015). However, the information concerning the adsorption of indoor low concentration of VOCs on activated carbons is still scarce in the open literature. The carbonaceous porous materials with a great flexibility in the regeneration operating conditions, a reduced maintenance, and low operating costs in the desorption procedure have received increasing attention in the recent years (Economy et al., Citation1996; Brasquet and Le Cloirec, Citation1997; Subrenat and Le Cloirec, Citation2006). ACF has been considered to be one of the promising adsorbents for removing VOCs due to its high adsorption capacity and fast adsorption kinetics in gas or liquid phase (Sullivan et al., Citation2001; Huang et al., Citation2003; Sullivan et al., Citation2004). However, ACF application is limited by the sensibility to high temperature, pore blocking and hygroscopicity, and a lack of regenerative ability (Zhao et al., Citation1998; Makowski and Kuśtrowski, Citation2007). Furthermore, the regeneration of the adsorbent at high temperatures can damage the surface structure of activated carbon such as ACF (Ania et al., Citation2005; Yu et al., Citation2015).
It will also be of concern that pressure drop should be kept to the lowest level because of the energy cost when large volumes of polluted gas are treated. Both the regenerative adsorption materials and the combustion catalyst should also be conformed to the lowest pressure drop. The Pt/Ni catalyst was chosen for this present research, which has widely been applied in the field of catalysis (Do et al., Citation2012). Among these VOCs, toluene is considered to be a very common pollutant (Liu et al., Citation2013), so toluene is chosen as a model pollutant to evaluate the VOC treatment process in this paper.
The thermal stability of the carbon materials is related to the oxygenated groups on their surface. Doping into P or B is one of the main ways to improve the stability of carbon materials in high temperature (Zhang et al., Citation2008). Tetraethoxysilane (TEOS) will react with hydroxyl in ACF-x pore to form C-O-Si≅ in the silylation process; Si may also improve the stability of the carbon materials as well as the action of P or B. The study intended to improve the hydrophobicity, thermal stability, and adsorption capacity of the carbon material by means of adding TEOS in ACF-x, and meanwhile, to explore the effect of the desorption or regeneration of the appropriately modified ACF adsorption material and to investigate the effect of the purification combining with the catalyst in condition of very low concentration of toluene. The evaluation results predict that it will be a feasible process, saving energy by combining the regeneration of ACF-x by heating with catalytic oxidation process. Toluene can be completely converted, while ACF-x modified by SiO2 shows the stronger hydrophobicity, thermal stability, and adsorption capacity, which display almost no effect in the presence of water vapor and no destruction in multiple cycles.
Experimental
Materials and methods
The raw ACF used in this study was purchased from Zhongsida Environmental Technology Company in Zhengzhou, China. ACFs modified by SiO2 were prepared as follows: 200 mg ACF was dispersed in 50 mL of ethanol followed by adding 0.05 g of cetyltrimethylammonium bromide (CTAB; 99%; Beijing Chemical Reagent Co., Ltd, Beijing, China) and 10 mL of ammonium hydroxide (NH4OH; Beijing Chemical Reagent) and stirred at room temperature for 30 min, then 12.5, 20, 40, or 80 μL of tetraethylorthosilicate (TEOS; 99.999%; Beijing Chemical Reagent Co., Ltd, Beijing, China) was slowly dropped into the above mixture, then stirred at room temperature for 24 hr. The products were collected by centrifugation, washing with ethanol for three times, and reflux extraction using ethanol of ammonium nitrate solution. The final products were referred to as ACF-12.5, ACF-20, ACF-40, and ACF-80 according to the dosage of TEOS, which correspond to SiO2 contents of 1.67%, 2.67%, 5.35%, and 10.71%, respectively. The best sample among them will be selected for further study.
Pt/Ni catalysts were prepared by displacement reaction of H2PtCl6 with foam Ni (70 mesh); it was purchased from Jiayisheng Electronical Company in Kunshan, China. Firstly, the foam metal was preprocessed, stirring with ultrasound in dilute nitric acid and ethyl alcohol for 0.5 hr, respectively. Then, about 2.44 g the foam metal was taken out and dried in the air for 6 hr at 100 °C. Secondly, the treated foam metal was soaked in 0.03 mol/L H2PtCl6 solution (19.5 mL) and then stirred for 3 hr in room temperature. Finally, the material was washed with alcohol and dried in the air for 6 hr at 100 °C. The sample was obtained and labeled as ~4.5 wt% Pt/Ni catalyst.
Sample characterization
To better understand the surface structure, photomicrographs of the surface of these adsorbents were obtained by scanning electron microscope (SEM) (Hitachi S-4800; Honshu, Japan); an energy-dispersive X-ray spectrometer (EDX) was used for analyzing the component of the adsorbents.
To study the effect of the porosity of the adsorbents on toluene adsorption, the specific surface area (SSA) and pore size distribution were determined from the N2 sorption-desorption isotherms at −196 °C using a vacuum volumetric sorption instrument (ASAP-2010; Quantachrome Ins, USA). The total pore volume and specific surface area were calculated by the Brunauer-Emmett-Teller (BET) method, and the average pore sizes were obtained by the Barrett-Joyner-Halenda (BJH) method (Tsai et al., Citation2008; Baur et al., Citation2015b). Fourier transform infrared (FTIR) transmission spectra of ACF-x samples were obtained employing a VERTEX 70 spectrometer (Bruker, Germany) in the wavenumber range of 400–4000 cm–1 and using KBr as the background to identify surface functional groups.
The samples were prepared for water contact angle test as follows: 40 μL 1-methyl-2-pyrrolidone was added into 10 mg carbon fiber or modified carbon fiber, fully grinding for 10 min and painting the samples onto aluminum foil, vacuum-drying at 120 °C for 30 min, and pressing at 10 MPa. The water contact angles of the samples were determined by a DSA10-Mk2 contact angle meter (Kruss GmbH, Hamburg, Germany).
Experimental setup and procedure
A schematic diagram of the experimental setup in the whole operating process is shown in . The experimental setup was composed of three sections, which included the low-toluene-concentration adjustment accomplished in the first section. Adsorption/desorption or/and catalytic oxidation test was accomplished in the second section, and toluene and other VOC contents were analyzed in the third section.
In the first section, the desired inlet concentration of toluene was obtained by bubbling Ar into liquid toluene, keeping at 30 °C using a thermostat water bath. The toluene concentration was adjusted to 0.5–5 ppmv scale by increasing flow rate in bypass. Further adjustment on toluene concentration was accomplished by increasing/decrease flow rate in the toluene line and decrease/increasing flow rate in the water line. In order to simulate the indoor environment, the water vapor was controlled by vaporizing water with the compressed air following the same principle to build a steam environment. The resulting gas-vapor mixture from the mixing chamber was sent to the adsorption section.
The second section consisted of the quartz tubular reactor (inner diameter 6 mm) filled with the adsorptive materials with provisions for the gas inlet and outlet. The reactor charged with the adsorbent was placed in a tubular oven for temperature control. The thermocouple used to monitor the bed temperature was mounted vertically in the center of the reactor. There was a provision for varying the bed temperature from 30 to 300 °C with the aid of an electrical heater and a temperature controller. The catalyst bed was set up by the same program.
In the third section, all the compounds were in situ detected by mass spectrometer (MS; QIC-20; Hiden, Warrington, UK).
In the adsorption process, 20 mg of sorbent was deposited on a glass wool layer; VOC gas flowed into the reactor and was adsorbed on the absorbent materials. The adsorption step was performed at 30 °C. After adsorption was accomplished, the VOC gas flow was switched off and replaced by air. Breakthrough was then defined as the time when VOC concentration in the outlet stream reached 3.5 ppmv, which saturation was defined as the point where the inlet and outlet VOC concentrations merged.
In simulating the indoor environment, the VOC gas flowed into the reactor and the wet reconstituted air (relative humidity 2.10%) also flowed into the reactor at same time. The adsorption capacity of the ACF-x materials was determined by a numerical integration of the area defined by the adsorptive concentration curve between t0 and tfinal when the outlet concentration was equal to the inlet one. The adsorption capacities were calculated by numerical integration of the breakthrough curves for the toluene adsorption. The experiments were carried out repeating five times, and the values reported were the average.
The adsorption/oxidation cycle experiments were performed using the following procedures: keeping toluene concentration at 2.05 ppmv, it was then passed through the adsorption materials, and toluene was completely adsorbed in the initial stage. Then when toluene concentration reached the initial value, toluene was switched into air until toluene concentration decreased to zero. The adsorbent bed was heated to 180 °C and kept for a period of time at 180 °C. Before heating the adsorbent bed, the Pt/Ni catalyst was heated to 250 °C and kept for a period of time at 250 °C. After the temperatures of the two furnaces dropped to 30 °C, the adsorption/catalytic process could begin the next cycle.
Results and discussion
Characterization of modified ACF-x
The shape and size of the raw ACF texture were a hole of ~3×5 mm and a diameter of 20 μm. Further steps were prepared to obtain the ACF modified by SiO2. The characterization of modified ACF was carried out by using SEM and EDX. The representative SEM images of the modified ACF samples are shown in . From SEM images, the modified materials on the surface of AFC had a significant and smooth cover layer with low content of silica (, ). Comparing with the raw ACF, the surface of the modified materials by silica had a significant change. The different images of the samples were due to the different contents of the silica, as seen in ; the ACF-80 had the most obvious change of the cover envelope. The coverage on the ACF surface in these images represented silica crystallites, which were also confirmed by EDX analysis.
Figure 2. (a) Digital picture of ACF and scanning electron microscope (SEM) images of (b) ACF, (c) ACF-12.5, (d) ACF-20, (e) ACF-40, and (f) ACF-80.
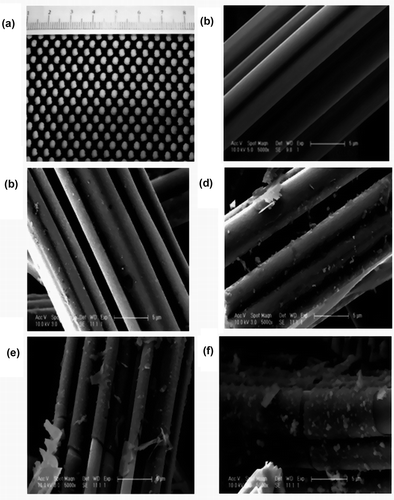
EDX analysis (Zabihi et al., Citation2015) was carried out to determine the chemical composition of the prepared material, a typical result is presented in . There appeared C, O, Si, and Au in all ACF-x samples, the Au being used as the metal coating for the samples to increase electron conduction. It was clear from the results that silica content increased with the added amount of TEOS, which is in agreement with the SEM observation. In order to evaluate the microshape of the ACF and the modified ACF much more precisely, the nitrogen gas physiosorption of materials was carried out in this study. It presented typical N2 adsorption isotherms over the samples and indicated a much higher nitrogen adsorption capacity of ACF-20 as compared with both ACF and ACF-80, as shown in .
Figure 4. Nitrogen adsorption isotherms (77 K) of (a) ACF, (b) ACF-12.5, (c) ACF-20, (d) ACF-40, and (e) ACF-80.
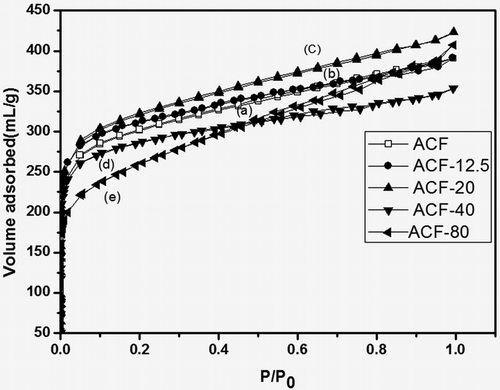
A Langmuir-type isotherm (Type I) was visible for all modified ACF samples, indicating monolayer nitrogen adsorption. It was also observed that ACF-80 slightly enhanced hysteresis loop (H4-type), which suggested an increased mesopore or macropore volume (Chiang et al., Citation2001). As shown in and , the sample ACF-20 increased appreciably the surface area and pore volume while keeping similar N2 adsorption curve shape. This indicated that the material retained microporous properties despite modified by SiO2. So it could be concluded that the rational amount of the silica could improve the SSA, the pore volume, and the adsorption capacity. But a gradual decrease of the porosity could be obtained by increasing the amount of silica excessively. Studies had shown that ACF pore volume and pore size distribution played a vital role in capturing adsorbates at low VOC emission rates, whereas the specific surface area and pore size distribution of ACF-x were primarily responsible for the adsorption of organic compounds (Son et al., Citation2016).
Table 1. Structural properties of ACF, ACF-12.5, ACF-20, ACF-40, and ACF-80.
FTIR spectroscopy is widely used in the analysis of solids and powders and generally requires little sample preparation. The spectra of ACF and the modified ACF modified with different amount of silica are shown in . Strong, broad–OH vibration band (~3400 cm−1) could be observed clearly in all samples; with the increase of the coverage of silica, the –OH band vibration became weaker due to –OH reacting with TEOS. Therefore, these results indicated that ACF modified by the silica could improve its hydrophobicity.
As shown in , the contact angle of the ACF was 50° before modification. The contact angles increased gradually with the addition of TEOS; the contact angle was 89° with addition of 20 μL of TEOS. It indicated that coating SiO2 could certainly enhance the hydrophobicity of ACF-x (Xu et al., Citation2013).
Toluene adsorption over ACF-x in present/absent of water
To determine the effects of the silica coverage on the adsorption of toluene, the experiments were carried out using ACF with various contents of silica in the same condition. In all the test runs, the weight of the ACF samples was taken to be 20 mg and the Ar and Air gas flow rates were maintained constant at 90 mL/min, and the inlet concentration of toluene was kept constantly at 4.5 ppmv.
The breakthrough curves of different samples are shown in . The toluene signal was equal to zero during initial stages; it indicated a total toluene removal and fast kinetics of toluene adsorption on ACF-x. In absent of water, the raw ACF showed the better adsorption property. When small silica content was deposited on ACF-x, it did not affect the adsorption properties of ACF-x. With an increase of silica content, the breakthrough time of ACF-x rapidly declined. The breakthrough time of ACF-80 was only 3.6 min, which was less than one seventh of that of ACF (25.6 min). As seen in , ACF was coating by silica; this coating layer blocked the pore of ACF.
Figure 8. Comparison of toluene breakthrough curves for raw/modified ACFs under anhydrous condition (90 mL/min Ar).
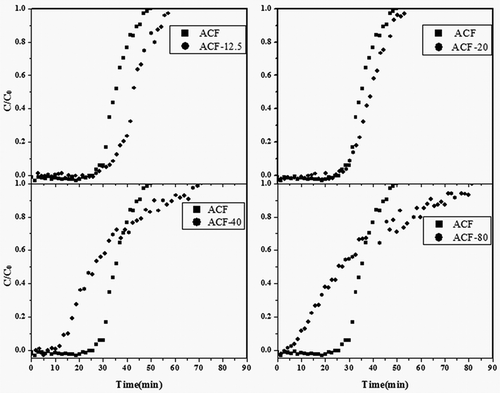
The adsorption experiments were also performed using feed streams containing VOCs in humid air with relative humidity 2.10% to simulate a true indoor air environment and to investigate the effect of water vapor on the performance of the adsorbents. The results showed that the adsorption capacity of the raw ACF was relatively low in the presence of water vapor as compared with ACF exposing to the inert gas (). In order to increase the toluene adsorption capacity, modifications of the raw ACF-x adsorbents by deposition of different proportion silica were carried out. The breakthrough curves of ACF modified by TEOS are shown in . The toluene signal over the unmodified ACF drops to zero at the beginning (total toluene removal) and then quickly returned to the initial level. Compared with the raw ACF, the breakthrough time was increased when the total content of TEOS was in the range of 12.5–20 μL. Also, a study has reported that a longer breakthrough time indicated a greater adsorption capacity for a given concentration (Huang et al., Citation2003). ACF-20 showed the largest adsorption capacity for toluene compared with the other ACF-x. A specific analysis showed that the breakthrough time deceased when the content of TEOS increased up to 40 μL, whereas the shape of the breakthrough curve changed and was different from the raw ACF. In this case, the curve was less steep, suggesting pronounced mass transfer limitations. Moreover, the steepness of the breakthrough curves insured fast adsorption kinetics. The breakthrough curve shape of the modified ACF samples was identical to the raw sample, suggesting similar adsorption kinetics. The adsorption kinetics are usually governed by the diffusion of the gas from the bulk to the fiber, namely, the internal mass transfer coefficient (Das et al., Citation2004; Fournel et al., Citation2010). From these results, it could be concluded by the constant micropore size upon silica treatment that the right amount of silica package could improve the hydrophobicity of the ACF. Therefore, ACF-20 had been selected as the appropriate adsorbent for the following experiments.
Effect of toluene concentration on adsorption capacity
To investigate the effects of very low VOC concentrations on the breakthrough characteristics, adsorption behavior, and the adsorption capacity, ACF-20 was selected to investigate the effects of toluene concentration on adsorption capacity. The experiments were carried out for varying VOC inlet concentrations (below 4 ppmv), which was close to practical condition. To shorten the adsorption time, 20 mg of ACF-20 was taken into the quartz tube for each test run. The bed temperature was set at the predetermined optimum temperature of 30 °C and the argon Ar flow rate kept at 90 mL/min; furthermore, different concentrations of toluene were obtained by adjusting the air flow rate. The breakthrough curves obtained for the toluene adsorption under the above-mentioned gas inlet concentrations are shown in . The breakthrough time decreased from 28.0 to 14.1 min as the inlet concentration was increased from 0.51 to 2.81 ppmv. For a given concentration, the longer breakthrough time indicated a greater adsorption capacity (Huang et al., Citation2003). The total adsorption time decreased from 130.6 to 95.7 min along with the increase of the concentration gradually. The adsorption capacity of ACF-20 under various concentrations could be obtained from this type of curves. The relationship between the concentration and the adsorption capacity could be explained from , which showed that with decrease in the inlet concentration while keeping all other operating parameters constant, the adsorption capacity also decreased gradually.
Figure 10. (a) Concentration effects on the breakthrough of toluene for ACF-20. (1) 0.51 ppmv (90 mL/min Ar, 120 mL/min air); (2) 1.02 ppmv (90 mL/min Ar, 100 mL/min air); (3) 1.54 ppmv (90 mL/min Ar, 90mL/min air); (4) 2.05 ppmv (90 mL/min Ar, 70 mL/min Air); (5) 2.81 ppmv (90 mL/min Ar, 40 mL/min air); (6) 4.5 ppmv (90 mL/min Ar, 90 mL/min air). (b) Toluene adsorption isothermal curve for ACF-20.
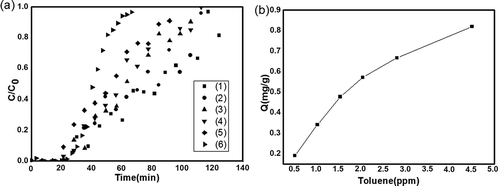
Effect of adsorption temperature on adsorption capacity
Since the adsorption is an exothermic process, which means that significant amounts of heat maybe evolve during the adsorption step, according to Le Chatelier’s principle, the magnitude of adsorption should increase with a decrease of temperature (Huang et al., Citation2004; Thevenet et al., Citation2014). An increase of adsorption temperatures would decrease the adsorption capacity of organic compounds in gas streams and promote VOC desorption. The more effective desorption of VOCs occurs at higher temperatures, reflecting the exothermic nature of their adsorption interaction with the carbon surface (Feng et al., Citation2005).
So in order to determine the effects of temperature on the VOC adsorption, the experiments were carried out at varying bed temperatures (in the range of 30–80 °C). In the series of test runs, the inlet concentration of toluene was kept constant at 3 ppmv, the weight of the selected ACF-20 samples was taken to be 20 mg, the Ar flow rate was maintained constant 200 mL/min, and the air flow rate was maintained constant 100 mL/min. The toluene breakthrough curves for the ACF-20 under the five different adsorption temperatures are shown in . The comparison between adsorption capacities could be done directly from the breakthrough curves. It may be observed in that with an increase of adsorption temperature, the breakthrough time was found to decrease from 5.8 to 2.6 min; correspondingly, the total adsorption time also decreased from 44.4 to 26.8 min. As also observed from the curves, the breakthrough characteristics began to shift to the left as the temperature was gradually increased to 50 °C and higher. When the adsorption temperature increased to 100 °C, it early reached the saturation adsorption, resulting in a sharp rising in the breakthrough curve. By integration of this type of curves, toluene adsorption capacities at these different temperatures were obtained. As shown in , the variable adsorption capacities for toluene over ACF-20 could be achieved under a series of different temperatures. It indicated that toluene adsorption capacities varied from 0.48 to 0.21 mg toluene/g ACF-20 with the increase of the adsorption temperature. The result was ascribed to VOC physical adsorption onto ACF-20 (Huang et al., Citation2003). Studies had shown that the VOC adsorption by ACF in a fixed bed under isothermal condition was considered to be controlled by the diffusion of the gas from the bulk to the fiber, which is dependent on the mass transfer coefficient. With increase in the temperature, the pore diffusion coefficient is expected to be higher and favorable for pore diffusion (Das et al., Citation2004). In this study, the toluene desorption rose gradually when the adsorption temperature was increased to above 48 °C by combining the results of the temperature-programmed desorption of toluene (). The data from the two figures summarized that a temperature of 30 °C was the most favorable for ACF in capturing low level of toluene from the indoor air pollutants. Furthermore, increasing temperature resulted in decreasing capacity, corresponding to decreasing breakthrough time.
Toluene elimination by adsorption and catalytic oxidation
In order to reduce the damage of the adsorbents, a suitable desorption temperature must be ascertained, which was used for the regeneration process. As shown in , toluene desorption was accomplished by increasing the temperature from 25 up to 200 °C at rate of 2 °C/min). Toluene began to desorp at 48 °C, the maximum value appeared at 90 °C, and it desorbed completely at over 180 °C. A suitable desorption temperature was 180 °C, which could ensure toluene desorbed completely and reduced the damage of the adsorbents.
Pt/Ni catalyst for catalytic oxdation is shown in The hole size of foam metal Ni was 70 mesh, with which the advantage of the low pressure drop. The size of Pt nanoparticle on foam metal Ni was about 100 nm. In regeneration experiments, toluene was first adsorbed on the adsorbent bed at 30 °C, and then desorbed, and the desorbed toluene was oxidized over the Pt/Ni catalyst, the catalyst bed of which was first heated to desired temperature, then the adsorbent bed was heated to 180°C.
Figure 13. SEM images of (a) foam metal Ni, (b) specific scale foam metal Ni, and (c) Pt/Ni foam metal catalyst.

shows toluene conversion as a function of temperature of the catalyst. It would cause energy waste if the temperature was too high; otherwise, it would not convert toluene completely. In , it was found that the toluene conversion was only about 10% below the bed temperature of 200 °C; however, further increase in the catalyst bed temperature resulted in significant increase in toluene conversion; toluene converted completely into CO2 and H2O approaching 230 °C. Therefore, 250 °C was identified as the most suitable catalyst bed temperature for toluene oxidation.
Based on the above results, the toluene was first adsorbed for 40 min at 30 °C, corresponding to the amount of the adsorption of toluene stored on the adsorbent until saturation condition, then the air feed switch was opened while the catalyst bed temperature was increased up to 250 °C, lasting 45 min, and the oven of the absorbent bed was heated to the most appropriate temperature (180 °C). As shown in , the toluene concentration remained zero in the first 20 min and then reached the adsorption equilibrium gradually. Subsequently, when the bed temperature of both the adsorbent and catalyst reached the specified criteria, the toluene concentration became lower and lower until it reached zero. Meanwhile, the CO2 concentration began to increase, which indicated that all the toluene desorbed was converted into CO2 and H2O on the catalyst bed. After 70 min, the CO2 and toluene concentrations became zero, which meant that adsorbent was regenerated completely and no by-products were observed.
Figure 15. Toluene (○) and CO2 (△) concentrations during toluene adsorption/oxidation over ACF-20–Pt/Ni system.
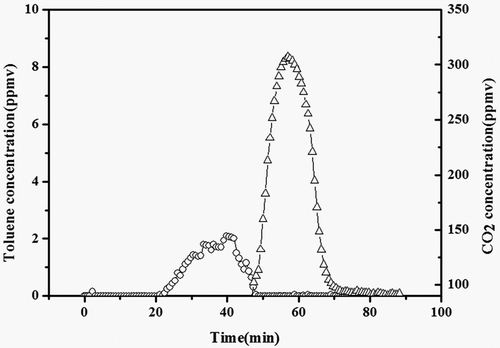
For adsorption/oxidation experiments, VOCs were first adsorbed over the adsorbent bed at room temperature, and then desorbed and oxidized over the catalyst bed by control different temperature of the two ovens. VOC and CO2 concentrations were measured as function of time on stream. ACF-20 was chosen as adsorbent medium because it exhibited the perfect performance among all the adsorbents tested for the adsorption of low level of toluene, in comparison with other published studies (Das et al., Citation2004; Lillo-Ródenas et al., Citation2005, Citation2006).
In order to reduce the damage of the adsorbents, the maximum desorption temperature was kept at 180 °C and the catalyst bed temperature kept at 250 °C. The ability to retain its adsorption capacity after successive adsorption and desorption was one of the major characteristics of a good adsorbent (Das et al., Citation2004). A good adsorbent not only has large adsorption capacity, but also need good renewable performance. However, the regeneration of the adsorbent at high temperatures can damage the surface structure of activated carbons such as ACF-x (Ania et al., Citation2005; Chen et al., Citation2005). So in order to evaluate the modified adsorbent and catalyst stability, five adsorption/oxidation cycles were performed on the same system. After the first adsorption/oxidation step, the second cycle began to work after the adsorption bed temperature cooled down to 30 °C (). It was obvious that the ACF-20 adsorption capacity remained constant along the five cycles, as the toluene breakthrough time did not happen earlier and the whole adsorption time remained almost the same. So the thermal endurance property of the ACF covered with a small amount of silica had improved to a large extent. Moreover, the catalyst did not suffer any deactivation, as not only the amount of CO2 remained unchanged, but also no extra toluene was detected during the oxidation. Therefore, it could be inferred that the dual-function system with ACF-20 and the catalyst is a promising system for the continuous eliminating of low level of VOCs existing widely in the indoor air.
Conclusions
In summary, the silica-coated modification of ACF materials could improve the hydrophobicity and heat stability. Based on the adsorption capacity for toluene, ACF-20 was considered as the most efficient material among the ACF-x used in this study. In addition, the surface specific area of ACF-20 was higher than the other adsorbents. Adsorption/catalytic oxidation system was suggested as an effective method for the treatment of very low concentration levels of VOCs. So in this study, we combined the efficient VOC adsorbent with an oxidative catalyst to purify the indoor air; the modified ACF-20 covered by silica has the best adsorption capacity among these modified ACF materials for toluene adsorption in humid conditions. Moreover, the dual-function system of regenerative materials and combustion catalyst would be efficient in consecutive toluene adsorption/oxidation cycles. Consequently, adsorption/catalytic oxidation could be a promising technique for the removal of toluene, especially in the case of very low concentration levels as the indoor air.
Funding
The authors wish to acknowledge the financial support of National Key Research and Development Program (Grant No. 2016YFC0204301).
Additional information
Funding
Notes on contributors
Zhen-Zhen Xie
Zhen-Zhen Xie and Lin Wang are master’s degree students in the College of Public Health, Jilin University, Changchun, People’s Republic of China.
Lin Wang
Zhen-Zhen Xie and Lin Wang are master’s degree students in the College of Public Health, Jilin University, Changchun, People’s Republic of China.
Ge Cheng
Lei Shi is a professor in the College of Public Health, Jilin University, Changchun, People’s Republic of China.
Lei Shi
Ge Cheng is a lecturer in Jilin University, Changchun, People’s Republic of China.
Yi-Bo Zhang
Yi-Bo Zhang is an associate research fellow in Changchun Institute of Applied Chemistry, Chinese Academy of Sciences, Changchun, People’s Republic of China.
References
- Alcañiz-Monge, J., M. Pérez-Cadenas, and J. Marco-Lozar. 2012. Removal of harmful volatile organic compounds on activated carbon fibres prepared by steam or carbon dioxide activation. Adsorpt. Sci. Technol. 30:473–482. doi: 10.1260/0263-6174.30.6.473
- Ania, C., J. Parra, J. Menendez, and J. Pis. 2005. Effect of microwave and conventional regeneration on the microporous and mesoporous network and on the adsorptive capacity of activated carbons. Microporous Mesoporous Mater. 85:7–15. doi:10.1016/j.micromeso.2005.06.013
- Baek, S.-W., J.-R. Kim, and S.-K. Ihm. 2004. Design of dual functional adsorbent/catalyst system for the control of VOC’s by using metal-loaded hydrophobic Y-zeolites. Catal. Today 93– 95: 575–581. doi:10.1016/j.cattod.2004.06.107
- Baur, G.B., O. Beswick, J. Spring, I. Yuranov, and L. Kiwi-Minsker. 2015a. Activated carbon fibers for efficient VOC removal from diluted streams: The role of surface functionalities. Adsorption 21:255–264. doi: 10.1007/s10450-015-9667-7
- Baur, G.B., I. Yuranov, and L. Kiwi-Minsker. 2015b. Activated carbon fibers modified by metal oxide as effective structured adsorbents for acetaldehyde. Catal. Today 249:252–258. doi:10.1016/j.cattod.2014.11.021
- Bedia, J., J.M. Rosas, J. Rodríguez-Mirasol, and T. Cordero. 2010. Pd supported on mesoporous activated carbons with high oxidation resistance as catalysts for toluene oxidation. Appl. Catal. B 94:8–18. doi:10.1016/j.apcatb.2009.10.015
- Brasquet, C., and P. Le Cloirec. 1997. Adsorption onto activated carbon fibers: Application to water and air treatments. Carbon 35:1307–1313. doi:10.1016/S0008-6223(97)00079-1
- Chen, W., J.S. Zhang, and Z. Zhang. 2005. Performance of air cleaners for removing multiple volatile organic compounds in indoor air. ASHRAE Trans. 111:1101–1114.
- Chiang, Y.-C., P.-C. Chiang, and C.-P. Huang. 2001. Effects of pore structure and temperature on VOC adsorption on activated carbon. Carbon 39:523–534. doi:10.1016/S0008-6223(00)00161-5
- Chintawar, P.S., and H.L. Greene. 1997. Adsorption and catalytic destruction of trichloroethylene in hydrophobic zeolites. Appl. Catal. B 14:37–47. doi:10.1016/S0926-3373(97)00010-6
- Das, D., V. Gaur, and N. Verma. 2004. Removal of volatile organic compound by activated carbon fiber. Carbon 42:2949–2962. doi:10.1016/j.carbon.2004.07.008
- Do, P.T.M., A.J. Foster, J. Chen, and R.F. Lobo. 2012. ‘Bimetallic effects in the hydrodeoxygenation of meta-cresol on γ-Al2O3 supported Pt–Ni and Pt–Co catalysts. Green Chem. 14:1388–1397. doi:10.1039/c2gc16544a
- Economy, J., M. Daley, and C. Mangun. 1996. Activated carbon fibers-past, present, and future. Urbana 51:61801.
- Feng, W., S. Kwon, E. Borguet, and R. Vidic. 2005. Adsorption of hydrogen sulfide onto activated carbon fibers: Effect of pore structure and surface chemistry. Environ. Sci. Technol. 39:9744–9749. doi:10.1021/es0507158
- Fournel, L., P. Mocho, R. Brown, and P. Le Cloirec. 2010. Modeling breakthrough curves of volatile organic compounds on activated carbon fibers. Adsorption 16:147–153. doi:10.1007/s10450-010-9207-4
- Gaur, V., A. Sharma, and N. Verma. 2005. Catalytic oxidation of toluene and m-xylene by activated carbon fiber impregnated with transition metals. Carbon 43:3041–3053. doi:10.1016/j.carbon.2005.06.039
- Guieysse, B., C. Hort, V. Platel, R. Munoz, M. Ondarts, and S. Revah. 2008. Biological treatment of indoor air for VOC removal: Potential and challenges. Biotechnol. Adv. 26:398–410. doi:10.1016/j.biotechadv.2008.03.005
- Guillemot, M., J. Mijoin, S. Mignard, and P. Magnoux. 2007. Adsorption of tetrachloroethylene on cationic X and Y zeolites: Influence of cation nature and of water vapor. Ind. Eng. Chem. Res. 46:4614–4620. doi:10.1021/ie0616390
- Gupta, K.N., N.J. Rao, and G.K. Agarwal. 2015. Gaseous phase adsorption of volatile organic compounds on granular activated carbon. Chem. Eng. Commun. 202:384–401. doi:10.1080/00986445.2013.840827
- Hu, X., S. Qiao, X.S. Zhao, and G.Q. Lu. 2001. Adsorption study of benzene in ink-bottle-like MCM-41. Ind. Eng. Chem. Res. 40:862–867. doi:10.1021/ie000496t
- Huang, Z.-H., F. Kang, and J. Hao. 2004. Effect of temperature on the adsorption of organic vapours on activated carbon fibres. Adsorpt. Sci. Technol. 22:327–335. doi:10.1260/0263617041514884
- Huang, Z.H., F.Y. Kang, K.M. Liang, and J.M. Hao. 2003. Breakthrough of methyethylketone and benzene vapors in activated carbon fiber beds. J. Hazard. Mater. 98:107–115. doi:10.1016/S0304-3894(02)00284-4
- Jo, W.-K., and C.-H. Yang. 2009. Granular-activated carbon adsorption followed by annular-type photocatalytic system for control of indoor aromatic compounds. Sep. Purif. Technol. 66:438–442. doi:10.1016/j.seppur.2009.02.014
- Karimnezhad, L., M. Haghighi, and E. Fatehifar. 2014. Adsorption of benzene and toluene from waste gas using activated carbon activated by ZnCl2. Front. Environ. Sci. Eng. 8:835–844. doi:10.1007/s11783-014-0695-4
- Khan, F.I., and A.K. Ghoshal. 2000. Removal of volatile organic compounds from polluted air. J. Loss Prev. Process Ind. 13:527–545. doi:10.1016/S0950-4230(00)00007-3
- Kim, K.-J., C.-S. Kang, Y.-J. You, M.-C. Chung, M.-W. Woo, W.-J. Jeong, N.-C. Park, and H.-G. Ahn. 2006. Adsorption–desorption characteristics of VOCs over impregnated activated carbons. Catal. Today 111:223–228. doi:10.1016/j.cattod.2005.10.030
- Lee, J.W., W.G. Shim, and H. Moon. 2004. Adsorption equilibrium and kinetics for capillary condensation of trichloroethylene on MCM-41 and MCM-48. Microporous Mesoporous Mater. 73:109–119. doi:10.1016/j.micromeso.2004.04.020
- Lillo-Ródenas, M.A., D. Cazorla-Amorós, and A. Linares-Solano. 2005. Behaviour of activated carbons with different pore size distributions and surface oxygen groups for benzene and toluene adsorption at low concentrations. Carbon 43:1758–1767. doi:10.1016/j.carbon.2005.02.023
- Lillo-Ródenas, M.A., A.J. Fletcher, K.M. Thomas, D. Cazorla-Amorós, and A. Linares-Solano. 2006. Competitive adsorption of a benzene–toluene mixture on activated carbons at low concentration. Carbon 44:1455–1463. doi:10.1016/j.carbon.2005.12.001
- Liu, L., D. Huang, and F. Yang. 2009. Toluene recovery from simulated gas effluent using POMS membrane separation technique. Sep. Purif. Technol. 66:411–416. doi:10.1016/j.seppur.2008.12.023
- Liu, Z.S., J.Y. Chen, and Y.H. Peng. 2013. Activated carbon fibers impregnated with Pd and Pt catalysts for toluene removal. J. Hazard. Mater. 256–257:49–55. doi:10.1016/j.jhazmat.2013.04.007
- Lu, C.-Y., M.-Y. Wey, and K.-H. Chuang. 2009. Catalytic treating of gas pollutants over cobalt catalyst supported on porous carbons derived from rice husk and carbon nanotube. Appl. Catal. B 90:652–661. doi:10.1016/j.apcatb.2009.04.030
- Makowski, W., and P. Kuśtrowski. 2007. Probing pore structure of microporous and mesoporous molecular sieves by quasi-equilibrated temperature programmed desorption and adsorption of n-nonane. Microporous Mesoporous Mater. 102:283–289. doi:10.1016/j.micromeso.2007.01.009
- Manjare, S.D., and A.K. Ghoshal. 2006. Studies on adsorption of ethyl acetate vapor on activated carbon. Ind. Eng. Chem. Res. 45:6563–6569. doi:10.1021/ie0603060
- Nigar, H., N. Navascues, O. de la Iglesia, R. Mallada, and J. Santamaria. 2015. Removal of VOCs at trace concentration levels from humid air by microwave swing adsorption, kinetics and proper sorbent selection. Sep. Purif. Technol. 151:193–200. doi:10.1016/j.seppur.2015.07.019
- Raso, R.A., M. Zeltner, and W.J. Stark. 2014. Indoor air purification using activated carbon adsorbers: Regeneration using catalytic combustion of intermediately stored VOC. Ind. Eng. Chem. Res. 53:19304–19312. doi:10.1021/ie503851q
- Serrano, D.P., G. Calleja, J.A. Botas, and F.J. Gutierrez. 2004. Adsorption and hydrophobic properties of mesostructured MCM-41 and SBA-15 materials for volatile organic compound removal. Ind. Eng. Chem. Res. 43:7010–7018. doi:10.1021/ie040108d
- Sharmin, R., and M.B. Ray. 2012. Application of ultraviolet light-emitting diode photocatalysis to remove volatile organic compounds from indoor air. J. Air Waste Manage. Assoc. 62(9):1032–1039. doi:10.1080/10962247.2012.695760
- Son, H., S. Sivakumar, M. Rood, and B. Kim. 2016. Electrothermal adsorption and desorption of volatile organic compounds on activated carbon fiber cloth. J. Hazard. Mater. 301:27–34. doi:10.1016/j.jhazmat.2015.08.040
- Subrenat, A.S., and P.A. Le Cloirec. 2006. Volatile organic compound (VOC) removal by adsorption onto activated carbon fiber cloth and electrothermal desorption: An industrial application. Chem. Eng. Commun. 193:478–486. doi:10.1080/00986440500191768
- Sullivan, P.D., M. Rood, K. Hay, and S. Qi. 2001. Adsorption and electrothermal desorption of hazardous organic vapors. J. Environ. Eng. 127:217–223. doi:10.1061/(ASCE)0733-9372(2001)127:3(217)
- Sullivan, P.D., M.J. Rood, G. Grevillot, J.D. Wander, and K.J. Hay. 2004. Activated carbon fiber cloth electrothermal swing adsorption system. Environ. Sci. Technol. 38:4865–4877. doi:10.1021/es0306415
- Thevenet, F., L. Sivachandiran, O. Guaitella, C. Barakat, and A. Rousseau. 2014. Plasma-catalyst coupling for volatile organic compound removal and indoor air treatment: A review. J. Phys. D Appl. Phys. 47(22):224011–224015. doi:10.1088/0022-3727/47/22/224011
- Tsai, J.-H., H.-M. Chiang, G.-Y. Huang, and H.-L. Chiang. 2008. Adsorption characteristics of acetone, chloroform and acetonitrile on sludge-derived adsorbent, commercial granular activated carbon and activated carbon fibers. J. Hazard. Mater. 154:1183–1191. doi:10.1016/j.jhazmat.2007.11.065
- Xu, F., S. Xian, Q. Xia, Y. Li, and Z. Li. 2013. Effect of textural properties on the adsorption and desorption of toluene on the metal-organic frameworks HKUST-1 and MIL-101. Adsorpt. Sci. Technol. 31:325–340. doi:10.1260/0263-6174.31.4.325
- Xu, K.J., Q.W. Sun, Y.Q. Guo, and S.H. Dong. 2013. Effects of modifiers on the hydrophobicity of SiO2 films from nano-husk ash. Appl. Surf. Sci. 276:796–801. doi:10.1016/j.apsusc.2013.03.173
- Yamaguchi, T., K. Aoki, M. Sakurai, and H. Kameyama. 2013. Development of new hybrid VOCs treatment process using activated carbon and electrically heated alumite catalyst. J. Chem. Eng. Japan 46:802–810. doi:10.1252/jcej.13we112
- Yu, W., L. Deng, P. Yuan, D. Liu, W. Yuan, P. Liu, H. He, Z. Li, and F. Chen. 2015. Surface silylation of natural mesoporous/macroporous diatomite for adsorption of benzene. J. Colloid Interface Sci. 448:545–552. doi:10.1016/j.jcis.2015.02.067
- Zabihi, M., F. Khorasheh, and J. Shayegan. 2015. Supported copper and cobalt oxides on activated carbon for simultaneous oxidation of toluene and cyclohexane in air. RSC Adv. 5:5107–5122. doi:10.1039/C4RA14430A
- Zabihi, M., J. Shayegan, M. Fahimirad, and F. Khorasheh. 2015. Preparation, characterization and kinetic behavior of supported copper oxide catalysts on almond shell-based activated carbon for oxidation of toluene in air. J. Porous Mater. 22:101–118. doi:10.1007/s10934-014-9877-5
- Zhang, J., X. Liu, R. Blume, A.H. Zhang, R. Schlögl, and D.S. Su. 2008. Surface-modified carbon nanotubes catalyze oxidative dehydrogenation of n-butane. Science 322:73–77. doi:10.1126/science.1161916
- Zhao, X., Q. Ma, and G. Lu. 1998. VOC removal: Comparison of MCM-41 with hydrophobic zeolites and activated carbon. Energy Fuels 12:1051–1054. doi:10.1021/ef980113s
- Zuo, S., F. Liu, R. Zhou, and C. Qi. 2012. Adsorption/desorption and catalytic oxidation of VOCs on montmorillonite and pillared clays. Catal. Commun. 22:1–5. doi:10.1016/j.catcom.2012.02.002