ABSTRACT
Activated carbons were prepared from tobacco stem by chemical activation using potassium hydroxide (KOH), potassium carbonate (K2CO3), and zinc chloride (ZnCl2). The effects of the impregnation ratio (activating agent/precursor) and activating agents on the physical and chemical properties of activated carbons were investigated. The textual structure and surface properties of activated carbons were characterized by nitrogen (N2) adsorption isotherm, scanning electron microscopy (SEM), Fourier-transform infrared spectroscopy (FT-IR), x-ray photoelectron spectroscopy (XPS), and thermogravimetry (TG). ZnCl2, acting as a superior activating agent compared to the others, produced much more porosity. The maximum specific surface area reached 1347 m2/g, obtained by ZnCl2 activation with an impregnation ratio of 4.0. Moreover, ZnCl2 activation yielded products with an excellent thermostability, attributed to different activation mechanisms. Various oxygen functions were detected on the activated carbon surface, and hydroxyl and ester groups were found to be in the majority.
Implications: Tobacco stem, the residue from cigarette manufacturing, is usually discarded as waste, leading to serious resource waste and environmental problems. This study provides an effective utilization available for this solid residue by using it as the starting material in the preparation of activated carbon with chemical activation. Activated carbons with high specific area and various surface functions have been prepared, and the effects of the amount and type of activating agents on the physical and chemical properties of activated carbon were investigated as well.
Introduction
China is the largest producer of tobacco in the world. According to statistics from the China Tobacco Monopoly Bureau, Chinese tobacco leaf output in 2014 reached 2,325,000 tons, while the stem content accounts for 25–30% of the total weight on average. Tobacco stem is the residue from cigarette manufacturing, and it is easy to collect as its production is concentrated in cigarette factories. At present, more than 95% of tobacco stem ends up in landfills or incineration, leading to serious waste of resource and to environmental problems (Mumba and Phiri, Citation2008). The properties of raw tobacco stem are given in .
Table 1. Characteristics of tobacco stem.
Raw tobacco waste has certain inherent defects, such as high oxygen content, high alkaline earth metal content, and low heat value, which limit its application in thermal power plants. Several proposals have been put forth to manage biomass wastes, for instance, microbiological degradation and conversion to energy with thermochemical methods such as pyrolysis, gasification, and liquefaction (Loh, Citationin press). However, the lignocellulosic nature of tobacco stem is a major challenging technical issue for its biodegradation (Herrmann et al., Citation2016). Besides, thermochemical methods always result in high energy consumption and additional problems like tar and tail-gas treatment (Hosoya et al., Citation2007). Actually, the high carbon, high volatile matter, and low ash content of tobacco stem make it an appropriate precursor for activated carbon.
Activated carbon has been widely used in adsorption and catalysis on account of its well-developed porous structure and remarkable adsorption capacity. Much research has been reported on the preparation of activated carbon from various agricultural wastes and on the effects of different preparation conditions on the characteristics of the activated carbon (Abioye and Ani, Citation2015; Jain et al., Citation2016; Kumar and Mohan Jena, Citation2015; Ozdemir et al., Citation2014; Torrellas et al., Citation2015). The structure and properties of the final activated carbon are greatly dependent on the nature of the precursor as well (Açıkyıldız et al., Citation2014; Falco et al., Citation2013). There is little systematic research on the preparation of activated carbon from tobacco residues. Kilic et al. (Citation2011) prepared activated carbon from tobacco residues by chemical activation with potassium hydroxide (KOH) and potassium carbonate (K2CO3) for phenol removal, in which the effect of impregnation ratio on surface area was briefly mentioned. Li et al. (Citation2008a, Citation2008b) investigated the pyrolysis characteristics of plain tobacco stem and tobacco stem impregnated with phosphoric acid (H3PO4) through thermogravimetry, and reported the preparation of activated carbon from tobacco stem with K2CO3 activation using microwave radiation. The influence of impregnation ratio on the adsorption capacity of activated carbon was observed. However, the porous structure and surface chemistry of activated carbon have been paid little attention, and these in fact significantly influence the capacity of activated carbon for adsorption, catalysis, and other applications.
In this study, tobacco stem was used as a raw material for the preparation of activated carbon, and three activating agents, KOH (a strong base), K2CO3 (a Lewis base), and zinc chloride (ZnCl2, a Lewis acid), were utilized to study the influence of different chemicals on the structure of the activated carbon products. The effects of the amount and type of activating agents on the porous structure of activated carbon, including the specific surface area, pore volume, and pore size distribution, were investigated, and variations of the surface chemical characteristics and thermostability of activated carbon were analyzed as well.
Material and methods
Materials
Tobacco stem collected from the China Tobacco Hunan Industrial Co., Ltd., was first washed with distilled water and dried at 378 K, and then ground by a high-speed rotary cutting mill, with a particle size around 100–300 µm. KOH (>85.0%, Xilong), K2CO3 (>99.0%, HengXing), ZnCl2 (>98.0%, Sinopharm), and hydrochloride acid (HCl, 36–38%, XingKong) were used as received. Nitrogen (N2) gas of ultrahigh purity (99.999%) was provided by High-tech Gas Co., Ltd., China.
Preparation of activated carbon
Ten grams of dried precursor was mixed with 100 mL distilled water and 10 g of an activating agent, and the impregnation ratio was 1.0 in this case. After 1 hr of stirring at 323 K and 12 hr of aging at room temperature, the mixture was filtered and then dried at 378 K overnight to prepare the impregnated sample. The impregnated sample was heated up to 873 K at a heating rate of 10 K/min and held for 1.5 hr at the temperature under N2 atmosphere (1 L/min) in a horizontal tubular furnace, then cooled to room temperature with continuous N2 flush. The product was washed by HCl solution (1.0 M) and then distilled water until the pH became neutral, followed by drying at 378 K for 2 hr.
Textural and chemical characterization
The ultimate analysis of tobacco stem was performed using an elemental analyzer (Vario EL III, Elementar Analysensysteme GmbH, Germany). The proximate analysis was carried out in accordance with standards prescribed by the American Society for Testing and Materials (ASTM) (Köseoğlu and Akmil-Başar, Citation2015). The hemicellulose, cellulose, and lignin contents in tobacco stem were tested according to the China National Standards (Ding et al., Citation2013). The textural characterization of activated carbon was determined by N2 adsorption at 77 K with a surface area analyzer (SA3100, Beckman Coulter, Inc., USA). The sample was ground to powders and degassed at 373 K for 4 hr in vacuum before the test. The specific surface area (SBET), micropore volume (Vm), and micropore and mesopore size distribution were calculated from the adsorption data by the Brunauer–Emmett–Teller (BET) equation, t-plot method, Barrett–Joyner–Halenda (BJH) method, and Horvath–Kawazoe (HK) method, respectively (Fuertes and Sevilla, Citation2015; Jiménez-Cordero et al., Citation2014; Ma et al., Citation2016). The total pore volume (V) was estimated from the volume of N2 (as liquid) held at a relative pressure of 0.98. The microstructures of raw material and activated carbons were observed through a scanning electron microscope (SEM; Helios NanoLab 600i, FEI Co., USA) with an accelerating voltage of 5.0 kV. Fourier-transform infrared spectroscopy (FT-IR; Nicolet 6700 FT-IR, Thermo Fisher Scientific, Inc., USA) was used to qualitatively evaluate the surface chemical properties, and the spectrum was obtained over the frequency range of 400–4000 cm−1. X-ray photoelectron spectroscopy (XPS; ESCALAB 250Xi, Thermo Fisher Scientific, Inc., USA) was employed to investigate the chemical state of selected elements (i.e., carbon, oxygen, and nitrogen) and surface composition of activated carbon. The thermal characteristics of tobacco stem and activated carbons were determined by thermogravimetry (TG; STA 449 F3, Netzsch Co., Germany) from 313 to 1273 K under argon (Ar) atmosphere, with a heating rate of 10 K/min.
Results and discussion
TG characterization of raw tobacco stem
Thermogravimetric and differential thermogravimetric (DTG) analyses of tobacco stem are shown in . The weight loss of 3 wt% occurring at 373 K originated from the loss of moisture retained in tobacco stem. The main thermal degradation with a weight loss of 62 wt% took place between 373 and 873 K, ascribed to the release of volatiles resulted from the decomposition of cell wall biopolymer, such as cellulose, hemicellulose, lignin, sugars, and pectin (Mechati et al., Citation2015; Saka, Citation2012; Sung and Seo, Citation2009). In this temperature range, two strong peaks appeared around 508 and 549 K in the DTG curve, respectively. Moreover, there was no significant weight loss observed above 873 K, indicating that a temperature of 873 K could be chosen as the activation temperature for the preparation of activated carbon. From 313 to 1273 K, the total weight loss was 71 wt%.
Effects of impregnation ratio on pore structure
The surface area analyses of activated carbons prepared at various impregnation ratios by different activating agents are listed in .
Table 2. Pore structural properties of activated carbon (the activation temperature of 873 K and the activation time of 1.5 hr).
The BET surface area and micropore volume are plotted versus the impregnation ratio as displayed in . For each activating agent, the effects of impregnation ratio on the textural characteristics are discussed in subsequent sections.
Figure 2. Effects of impregnation ratio on (a) the BET surface area and (b) micropore volume of activated carbons.
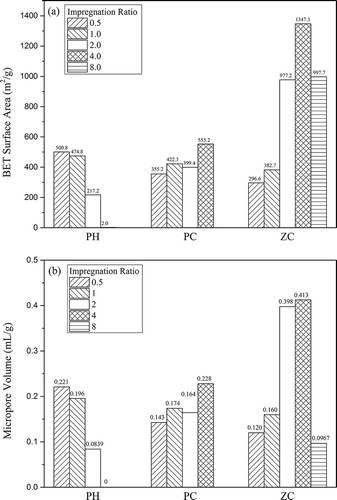
KOH activation
As shown in , the surface area and micropore volume followed a similar pattern, decreasing with increased impregnation ratio. For KOH activation, sample PH-0.5 was the optimal one, with the SBET of 501 m2/g and Vm of 0.22 mL/g. An incremental impregnation ratio reduced the porosity, with few pores obtained when the impregnation ratio was up to 4.0. Moreover, the micropore percentage declined as well, from 73% to 0 as the impregnation ratio rose from 0.5 to 4.0, indicating the widening of micropores to mesopores or even macropores. The results are different from those of other works (Lozano-Castelló et al., Citation2001; Muniandy et al., Citation2014), which have suggested that increasing the amount of KOH brings about an increase in surface area and pore volume.
The pore size distributions of activated carbons with KOH activation are presented in .
Figure 3. (a) Micropore and (b) mesopore volume distribution of activated carbons with KOH activation.
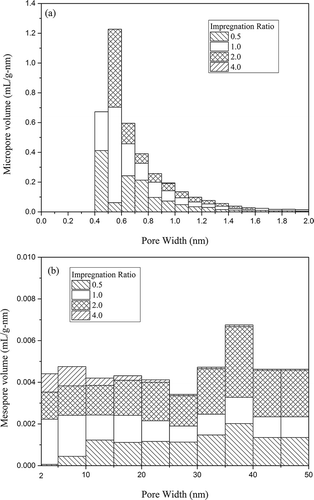
The micropores were concentrated in a range of 0.4–1.1 nm (). The widths of micropores were gradually enlarged with the increasing impregnation ratio. Micropore volume of PH-0.5 in 0.4–1.2 nm accounted for 95% of the total, while those of PH-1 and PH-2 almost distributed in 0.4–1.3 and 0.5–1.5 nm, respectively, and that of PH-4 totally in 0.9–2.0 nm. As shown in , except for PH-4, the column length corresponding to mesopore volume exhibited an uptrend with increasing impregnation ratio, with the longest one achieved at 35–40 nm, indicating pore widening that is in accord with the reduction of micropore percentage mentioned before.
KOH extracts to some extent the lignin component from tobacco stem and reacts with hydroxyl groups at the impregnation stage. During heat treatment, a reaction between the carbon that existed in the precursor and the hydroxide takes place, which facilitates intermolecular condensation reaction and retards the thermal degradation of cellulose molecules (Lillo-Ródenas et al., Citation2003; Oh et al., Citation2003). It is well known that two different mechanisms exist in the chemical activation: micropore formation and pore widening. The former starts with the addition of activating agent to the raw material, and the latter, which looms large with a reasonable megadose of chemicals, results from the reaction inside the existing pores. An increased dosage of KOH may cause overreaction between the precursor and activating reagent, leading to the widening of micropores and even the collapse of the framework structure, which eventually contributes to the decline of the micropore volume and its percentage and hence the surface area of the activated carbon.
K2CO3 activation
As shown in , for K2CO3 activation, increasing the impregnation ratio produced similar trends for the surface area and micropore volume, which presented a gentle rise on the whole. Also, the micropore percentage improved slightly according to , revealing an improvement of microporous structure. The maximum surface area and micropore volume, up to 553 m2/g and 0.23 mL/g, respectively, were obtained by PC-4, with a micropore percentage of 71%.
The pore size distributions of activated carbons with K2CO3 activation are exhibited in .
Figure 4. (a) Micropore and (b) mesopore volume distribution of activated carbons with K2CO3 activation.
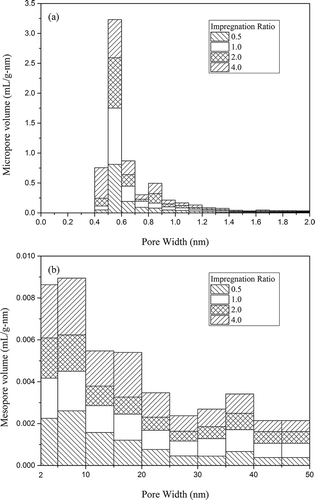
K2CO3 activation yielded mainly microporous activated carbons with no significant differences in the pore size distributions at different impregnation ratios. As shown in , a range of 0.4–1.1 nm was observed as the concentration area of micropore distribution, which accounted for more than 93% of the total, with an obvious bulge in the micropore volume at 0.5–0.6 nm. From , mesopores were dispersive all over the scale, and maximums were achieved at 5–10 nm, which accounted for approximately 20% of the total mesopore volume.
The impregnated K2CO3 restricts the formation of tar and intensifies dehydration and formation of cross-links (Adinata et al., Citation2007). The carbon is consumed by the reaction with K2CO3 during the activation process, producing K metal and carbon monoxide (CO), and high activation temperature would accelerate the activation reaction (Zhang et al., Citation2011). In terms of the experimental results, micropore formation holds all the trumps in the competition with pore widening, proved by the rising micropore percentage with the elevated impregnation ratio. Consequently, an incremental amount of K2CO3 promotes the formation of micropores, yielding products with a much larger surface area, as well as larger pore volume.
ZnCl2 activation
As shown in , the surface area and micropore volume first increased sharply with an incremental amount of ZnCl2, reaching the maximum at impregnation ratio 4.0, of 1347 m2/g and 0.41 mL/g, respectively, and then decreased. It is noteworthy that the total pore volume exhibited a sustained growth with increasing impregnation ratio, and the maximum up to 0.92 mL/g was achieved by ZC-8. Increasing the impregnation ratio from 2.0 to 4.0, the surface area presented a notable enhancement while the micropore volume was almost constant, indicating a remarkable growth of mesopores. In addition, according to , the micropore percentage declined from 88% to 11% with increasing the impregnation ratio from 2.0 to 8.0, coupled with an obvious increment in the total pore volume ascribed to mesopore development. There is an interesting contrast between ZC-2 and ZC-8, which have about the same surface areas around 1000 m2/g but totally different pore structures, demonstrating the competition between micropore formation and pore widening, which is explained in detail hereinafter.
The pore size distributions of activated carbons with ZnCl2 activation are displayed in .
Figure 5. (a) Micropore and (b) mesopore volume distribution of activated carbons with ZnCl2 activation.
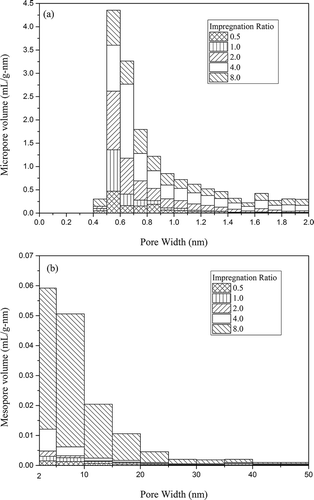
The analysis of pore size distribution also suggests that pore evolvement was greatly affected by the impregnation ratio. As shown in , micropores of ZC-0.5, ZC-1, ZC-2, ZC-4, and ZC-8 concentrated in the range of 0.4–1.2, 0.4–1.3, 0.5–1.6, 0.5–2.0, and 0.4–2.0 nm, respectively. It is clear that with increasing the impregnation ratio, the micropores were widened, and with a further increase of ZnCl2 amount the micropores were changed into mesopores. In , correspondingly, the columns were elongated with incremental reagent dosage pronouncing the mesopore growth, particularly rapidly from ZC-2 to ZC-4 to ZC-8. Moreover, mesopores with the pore width below 20 nm accounted for about 80% of the total mesopore volume.
ZnCl2 acts as a dehydration agent during the carbonization process, resulting in charring and aromatization reactions and development of the pore structure (Liou, Citation2010). Also, molecular hydrogen is evolved from the hydroaromatic structure of the precursor and leaves behind some sites for reaction (Uçar et al., Citation2009). There is a range in the impregnation ratio where the micropore formation and pore widening are competing with each other (Yang and Qiu, Citation2011). In the present case, the micropore creation mechanism is predominant as the impregnation ratio is below 2.0. Both mechanisms are responsible for porosity creation when the impregnation ratio is between 2.0 and 4.0, and with further increase in this ratio, pore widening gradually becomes the preferred one. In conclusion, increasing impregnation ratio promotes the formation of new pores and the widening of existing pores, resulting in an improvement of surface area. Conversely, an excessive amount of ZnCl2 would significantly destroy the micropore structure and enhance the formation of mesoporous structure which leads to a decline in surface area.
Effects of activating agent on surface characteristics
The adsorption capacity of activated carbon is greatly related to its specific surface area, pore volume, and pore size distribution (Li et al., Citation2008b). As samples with the highest surface areas obtained by each activating agent, PH-0.5, PC-4, and ZC-4 were selected for further investigation to discuss the effects of activating agent kind on the surface characteristics.
Pore structure
According to , the SBET of PH-0.5, PC-4 and ZC-4 are 501, 553, and 1347 m2/g, respectively. The total pore volume and micropore volume of PH-0.5 are 0.30 and 0.22 mL/g, respectively. PC-4 quite resembles PH-0.5, with a total pore volume of 0.32 mL/g and micropore volume of 0.23 mL/g. KOH activation resulted in carbon of low surface area similar to K2CO3 activation, whereas ZnCl2 activation yielded carbon of superior surface area due to a highly developed microporous structure. ZC-4 presents a total pore volume of 0.67 mL/g with a micropore volume of 0.41 mL/g, approximately twice that of PH-0.5 or PC-4. The results are consistent with other studies (Köseoğlu and Akmil-Başar, Citation2015; Molina-Sabio and Rodrı́guez-Reinoso, Citation2004), which indicate that carbon with high surface area and pore volume activated by potassium compounds is produced above 1073 K whereas the one by ZnCl2 is at lower temperatures (673–873 K).
To clearly illustrate differences in the effects of these three chemicals on the pore structure of activated carbons, the pore size distributions of PH-0.5, PC-4, and ZC-4 are shown in .
From , micropores of PH-0.5 and PC-4 concentrated in the range of 0.4–1.2 nm while those of ZC-4 concentrated in the range of 0.5–2.0 nm. The much wider pore size distribution of ZC-4 compared to PH-0.5 and PC-4 further confirmed its highly developed microporous structure. The mesopore distributions of these three samples varied from each other. As shown in , PH-0.5 generated mesopores mainly in the range of 10–50 nm, whereas PC-4 displayed a dispersive distribution throughout the mesopore region. In comparison, for ZC-4, mesopores with the pore width below 15 nm accounted for 84% of the total mesopore volume.
SEM technique was used to observe the surface physical morphology of raw tobacco stem and activated carbon samples. The micrographs are given in .
Figure 7. SEM graphs of (a, b) raw material and activated carbon samples: (c, d) PH-0.5, (e, f) PC-4, and (g, h) ZC-4.
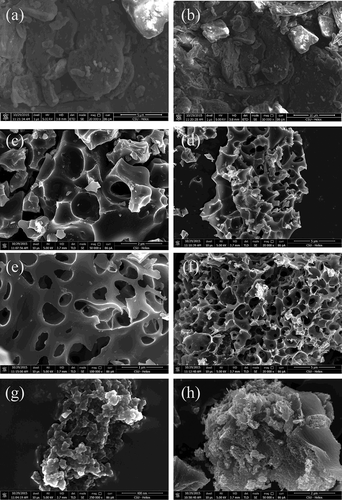
There are significant differences between the surface morphology of the tobacco stem and activated carbon samples. The tobacco stem presented a rough surface with little porosity, while surfaces of activated carbon samples were full of cavities as porous structures were created after chemical activation. Cracks, crevices, and some grains in various sizes in large holes appeared on activated carbon surfaces. Furthermore, there were differences among the activated carbons prepared by diverse activation agents as well. Pores of different sizes and shapes could be observed. The surface of PH-0.5 was similar to that of PC-4, with a honeycomb-like structure and parallel pore size. Comparatively, ZC-4 formed a sponge-like morphology, and the pore size was much smaller in general. Compared to the image with a same scale of 2 nm for PH-0.5 (Figure 7c), it is obvious that more micropores were developed in ZC-4 (), resulting in a notable increase of surface area.
As shown, ZnCl2 is a superior activating agent compared to KOH and K2CO3, and produces large quantities of micropores, generating higher micropore volume and therefore yielding porous carbon with a higher surface area.
Surface chemistry
The FT-IR spectra of the raw material and activated carbon samples are shown in . The spectrum of tobacco stem displayed a broad band at 3404 cm−1 ascribed to the O–H stretching vibration of hydroxyl groups and intrinsic moisture, and another at 2925 cm−1 due to C–H stretching vibration in aliphatic structures (Lua and Yang, Citation2005). The bands at 1617, 1423, 1246, and 1057 cm−1 could be corresponding to aromatic ring stretching vibration of the lignin, C–H deformation vibration in the lignin and carbohydrates, C–O stretching vibration, and C–O–C stretching vibration in the lignocellulose texture, respectively (Sevilla et al., Citation2011). In addition, a band presented at 604 cm−1, referring to N–H out-of-plane deformation vibration (Kumar and Mohan Jena, Citation2015). After activation treatment, the spectrum changed greatly. In spectra of PH-0.5, PC-4, and ZC-4, the band of O–H stretching vibration occurred at 3310 cm−1, indicating the presence of carboxylic or phenolic groups. Another broad one between 1400 and 1600 cm−1, assigned to a complicated combination of aromatic skeletal stretching vibration, C=O stretching vibration in, for instance, esters and carboxylic groups, and C–N stretching vibration in nitro surface groups (Kohl et al., Citation2010; Li et al., Citation2011; Yao et al., Citation2014; Zhou et al., Citation2007), was observed in each spectrum of activated carbons. The band at around 1200 cm−1 could be associated with the C–O stretching vibration in esters, carboxylic groups, or phenolic groups, occurring at 1211, 1161, and 1222 cm−1 for PH-0.5, PC-4, and ZC-4, respectively. Furthermore, a new band at 873 cm−1 appeared in the spectrum of PC-4, which might be attributed to the metallic compound resulted from K2CO3 activation (Wang et al., Citation2002).
The high lignocellulose content in raw tobacco stem contributes to the plentiful functional groups as observed in the FT-IR spectrum. The activating reagents contribute to aromatization of the structure during heat treatment (Köseoğlu and Akmil-Başar, Citation2015; Lillo-Ródenas et al., Citation2003; Uçar et al., Citation2009). The carbonyl and carboxyl groups are possibly formed during the impregnation and activation stage as a result of the extraction of hydrogen element and hydroxyl groups, which are the main components of lignocellulosic materials from the aromatic rings (Lua and Yang, Citation2005). A nitrogen content of 2% in the precursor might introduce nitrogen functions to the activated carbon surface. Moreover, HCl used to remove residual mineral matters after activation process promotes the formation of carboxyl groups and esters (Köseoğlu and Akmil-Başar, Citation2015).
The atom concentrations of carbon, oxygen, and nitrogen on the surface of activated carbon were estimated by XPS analysis and are listed in .
Table 3. Atomic percentage on the activated carbon surface from XPS analysis.
As shown, all the samples have much more oxygen content than conventional activated carbon (Fuertes and Sevilla, Citation2015; Jiménez-Cordero et al., Citation2014), coupled with a nitrogen concentration at about 2%. ZC-4 obtained the highest concentrations of carbon and nitrogen but the lowest oxygen concentration, while PC-4 obtained the lowest concentrations of carbon and nitrogen but the highest oxygen concentration. This could be illustrated by the different activation mechanisms associated with the three chemicals. Several mechanisms have been proposed by previous researchers on porosity development by activating agents. As a Lewis acid, ZnCl2 plays a dehydrating agent role in the activation process, contributing to aromatization of the structure without reaction with carbon (Köseoğlu and Akmil-Başar, Citation2015). Therefore, carbon concentration of the activated carbon by ZnCl2 activation is higher compared to that by KOH or K2CO3 activation, in which carbon is removed from the precursor by the reaction with the reagents. Activated carbon rich in oxygen content often means rich oxygen functional groups.
The XPS spectra of C1s and O1s peaks for different samples are shown in .
Figure 9. Peak deconvolutions of the C1s: (a) PH-0.5, (c) PC-4, and (e) ZC-4, and O1s XPS spectra: (b) PH-0.5, (d) PC-4, and (f) ZC-4.
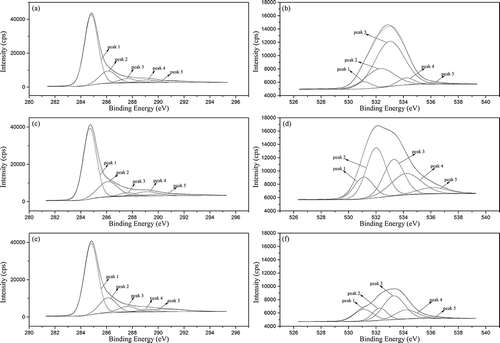
The C1s XPS spectra were deconvoluted into five peaks after baseline subtraction using Shirley’s method (Wang et al., Citation2002). Besides a symmetric peak centered at 284.7±0.1 eV ascribed to amorphous and aromatic carbon (this peak is generally referred to graphitic carbon), four other deconvoluted peaks were obtained at 286.1, 287.6, 289.1, and 290.6 eV, and corresponding to C–O or C–N bond, carbonyl groups, carboxyl groups, and carbonate species, respectively (Figueiredo and Pereira, Citation2010; Wang et al., Citation2015). Meanwhile, five peaks were obtained from the deconvolution of the O1s spectra. The peaks were assigned to different forms of O atoms: carbonyl groups (531.1 eV), hydroxyl groups (532.2 ± 0.2 eV), esters (533.2 ± 0.1 eV), carboxyl groups (534.2 eV), and water or oxygen adsorbed (536.1 eV) (Sedghi et al., Citation2015; Wang et al., Citation2015). These analyses coincide with the results from FT-IR measurements.
Distributions of carbon and oxygen species on the activated carbon surface were estimated from curve fitting and are listed in .
Table 4. The contribution of carbon and oxygen species.
From C1s spectra analysis, an amorphous carbon content above 60% in these samples was observed, in accordance with the nature of activated carbon. The dominance of hydroxyls and ester groups among all oxygen functions was clearly illustrated and further confirmed by the results of O1s spectra analysis. The close carbonate contents could be interpreted as the carbon dioxide adsorbed from ambient atmosphere. According to the analysis of O1s spectra, PH-0.5 showed a relatively low carboxyl content coupled with a high esters content, resulting from the reaction between KOH and carboxyl groups during activation process. In contrast, PC-4 presented the highest carboxyl content, in accordance with its high oxygen concentration, as a higher oxygen content could be attributed to more acidic groups on the surface (Gorgulho et al., Citation2008).
Thermal behavior
The thermal evolutions of activated carbons produced by different reagents were investigated by thermogravimetric analysis from 313 to 1273 K under Ar atmosphere, and the results are shown in .
As shown, for all the tested samples, the curves were descending all along, with different slopes. A loss at about 373 K was observed ascribed to moisture release. In addition, for PC-4, a loss equal to about 8 wt% occurred at 913–973 K, which might be related to the carbonate decomposition resulted from K2CO3 activation. PC-4 showed a larger presence of moisture and a higher presence of volatile matter with respect to PH-0.5 and ZC-4, and ZC-4 presented an excellent thermostability. The total mass losses were 22, 30, and 14 wt% for PH-0.5, PC-4, and ZC-4, respectively. It is deduced that the differences in thermal behaviors are attributed to the different chemical structures of activated carbon surfaces, as the surface with a higher amount of oxygen releases more volatile compounds.
Conclusion
Activated carbon with high specific surface area and excellent thermostability has been prepared from tobacco stem. Activation with different chemical agents and impregnation ratios significantly affects the characteristics of activated carbon, and ZnCl2 has a superior activation performance compared to KOH and K2CO3 under the experiential conditions. An incremental amount of ZnCl2 promotes the formation of new pores and the widening of existing pores, and pore widening gradually becomes dominant by further increment. Furthermore, various surface functional groups have been detected. Activated carbon with K2CO3 activation presents high oxygen concentration as well as high hydroxyl and carboxyl contents on the surface.
Funding
We acknowledge financial support from the National Nature Science Foundation of China (number 21376274) and the National Key Technology R&D Program of China (number 2015BAL04B02).
Additional information
Funding
Notes on contributors
Ruofei Chen
Ruofei Chen is a Ph.D. candidate and Liqing Li is a professor at the School of Energy Science and Engineering, Central South University, People’s Republic of China.
Zheng Liu
Zheng Liu is a Ph.D. graduated from the School of Energy Science and Engineering, Central South University, China. He is also a lecturer at the School of Environment, Guangxi University, People’s Republic of China.
Mingming Lu
Mingming Lu is an associate professor at the School of Energy, Environment, Biological and Medical Engineering, University of Cincinnati, USA.
Chunhao Wang
Chunhao Wang is a Ph.D. candidate, Hailong Li is a professor, and Weiwu Ma is an associate professor at the School of Energy Science and Engineering, Central South University, People’s Republic of China.
Shaobin Wang
Shaobin Wang is a professor at the Department of Chemical Engineering, Curtin University, Australia.
References
- Abioye, A.M., and F.N. Ani. 2015. Recent development in the production of activated carbon electrodes from agricultural waste biomass for supercapacitors: A review. Renew. Sustain. Energy Rev. 52:1282–93. doi:10.1016/j.rser.2015.07.129
- Açıkyıldız, M., A. Gürses, and S. Karaca. 2014. Preparation and characterization of activated carbon from plant wastes with chemical activation. Microporous Mesoporous Mater. 198:45–9. doi:10.1016/j.micromeso.2014.07.018
- Adinata, D., W.M. Wan-Daud, and M.K. Aroua. 2007. Preparation and characterization of activated carbon from palm shell by chemical activation with K2CO3. Bioresource Technol. 98(1):145–9. doi:10.1016/j.biortech.2005.11.006
- Ding, L., B. Zou, H. Liu, Y. Li, Z. Wang, Y. Su, Y. Guo, and X. Wang. 2013. A new route for conversion of corncob to porous carbon by hydrolysis and activation. Chem. Eng. J. 225:300–5. doi:10.1016/j.cej.2013.03.090
- Falco, C., J.P. Marco-Lozar, D. Salinas-Torres, E. Morallón, D. Cazorla-Amorós, M.M. Titirici, and D. Lozano-Castelló. 2013. Tailoring the porosity of chemically activated hydrothermal carbons: Influence of the precursor and hydrothermal carbonization temperature. Carbon 62:346–55. doi:10.1016/j.carbon.2013.06.017
- Figueiredo, J.L., and M.F.R. Pereira. 2010. The role of surface chemistry in catalysis with carbons. Catal. Today 150(1–2):2–7. doi:10.1016/j.cattod.2009.04.010
- Fuertes, A.B., and M. Sevilla. 2015. High-surface area carbons from renewable sources with a bimodal micro-mesoporosity for high-performance ionic liquid-based supercapacitors. Carbon 94:41–52. doi:10.1016/j.carbon.2015.06.028
- Gorgulho, H.F., J.P. Mesquita, F. Gonçalves, M.F.R. Pereira, and J.L. Figueiredo. 2008. Characterization of the surface chemistry of carbon materials by potentiometric titrations and temperature-programmed desorption. Carbon 46(12):1544–55. doi:10.1016/j.carbon.2008.06.045
- Herrmann, C., C. Idler, and M. Heiermann. 2016. Biogas crops grown in energy crop rotations: Linking chemical composition and methane production characteristics. Bioresource Technol. 206:23–35. doi:10.1016/j.biortech.2016.01.058
- Hosoya, T., H. Kawamoto, and S. Saka. 2007. Pyrolysis behaviors of wood and its constituent polymers at gasification temperature. J. Anal. Appl. Pyrolysis 78(2):328–36. doi:10.1016/j.jaap.2006.08.008
- Jain, A., R. Balasubramanian, and M.P. Srinivasan. 2016. Hydrothermal conversion of biomass waste to activated carbon with high porosity: A review. Chem. Eng. J. 283:789–805. doi:10.1016/j.cej.2015.08.014
- Jiménez-Cordero, D., F. Heras, M.A. Gilarranz, and E. Raymundo-Piñero. 2014. Grape seed carbons for studying the influence of texture on supercapacitor behaviour in aqueous electrolytes. Carbon 71:127–38. doi:10.1016/j.carbon.2014.01.021
- Kilic, M., E. Apaydin-Varol, and A.E. Putun. 2011. Adsorptive removal of phenol from aqueous solutions on activated carbon prepared from tobacco residues: Equilibrium, kinetics and thermodynamics. J. Hazard. Mater. 189(1–2):397–403. doi:10.1016/j.jhazmat.2011.02.051
- Kohl, S., A. Drochner, and H. Vogel. 2010. Quantification of oxygen surface groups on carbon materials via diffuse reflectance FT-IR spectroscopy and temperature programmed desorption. Catal. Today 150(1):67–70. doi:10.1016/j.cattod.2009.05.016
- Köseoğlu, E., and C. Akmil-Başar. 2015. Preparation, structural evaluation and adsorptive properties of activated carbon from agricultural waste biomass. Adv. Powder Technol. 26(3):811–8. doi:10.1016/j.apt.2015.02.006
- Kumar, A., and H. Mohan Jena. 2015. High surface area microporous activated carbons prepared from fox nut (Euryale ferox) shell by zinc chloride activation. Appl. Surf. Sci. 356:753–61. doi:10.1016/j.apsusc.2015.08.074
- Li, L., S. Liu, and J. Liu. 2011. Surface modification of coconut shell based activated carbon for the improvement of hydrophobic VOC removal. J. Hazard. Mater. 192(2):683–90. doi:10.1016/j.jhazmat.2011.05.069
- Li, W., J. Peng, L. Zhang, H. Xia, N. Li, K. Yang, and X. Zhu. 2008a. Investigations on carbonization processes of plain tobacco stems and H3PO4-impregnated tobacco stems used for the preparation of activated carbons with H3PO4 activation. Ind. Crops Prod. 28(1):73–80. doi:10.1016/j.indcrop.2008.01.006
- Li, W., L. Zhang, J. Peng, N. Li, and X. Zhu. 2008b. Preparation of high surface area activated carbons from tobacco stems with K2CO3 activation using microwave radiation. Ind. Crops Prod. 27(3):341–7. doi:10.1016/j.indcrop.2007.11.011
- Lillo-Ródenas, M.A., D. Cazorla-Amorós, and A. Linares-Solano. 2003. Understanding chemical reactions between carbons and NaOH and KOH: An insight into the chemical activation mechanism. Carbon 41(2):267–75. doi:10.1016/S0008-6223(02)00279-8
- Liou, T.H. 2010. Development of mesoporous structure and high adsorption capacity of biomass-based activated carbon by phosphoric acid and zinc chloride activation. Chem. Eng. J. 158(2):129–42. doi:10.1016/j.cej.2009.12.016
- Loh, S.K. In press. The potential of the Malaysian oil palm biomass as a renewable energy source. Energy Convers. Manage. doi:10.1016/j.enconman.2016.08.081
- Lozano-Castelló, D., M.A. Lillo-Ródenas, D. Cazorla-Amorós, and A. Linares-Solano. 2001. Preparation of activated carbons from Spanish anthracite: I. Activation by KOH. Carbon 39(5):741–9. doi:10.1016/S0008-6223(00)00185-8
- Lua, A.C., and T. Yang. 2005. Characteristics of activated carbon prepared from pistachio-nut shell by zinc chloride activation under nitrogen and vacuum conditions. J. Colloid Interface Sci. 290(2):505–13. doi:10.1016/j.jcis.2005.04.063
- Ma, X., L. Li, S. Wang, M. Lu, H. Li, W. Ma, and T.C. Keener. 2016. Ammonia-treated porous carbon derived from ZIF-8 for enhanced CO2 adsorption. Appl. Surf. Sci. 369:390–7. doi:10.1016/j.apsusc.2016.01.274
- Mechati, F., C. Bouchelta, M.S. Medjram, R. Benrabaa, and N. Ammouchi. 2015. Effect of hard and soft structure of different biomasses on the porosity development of activated carbon prepared under N2/microwave radiations. J. Environ. Chem. Eng. 3(3):1928–38. doi:10.1016/j.jece.2015.07.007
- Molina-Sabio, M., and F. Rodrı́guez-Reinoso. 2004. Role of chemical activation in the development of carbon porosity. Colloids Surf. A Physicochem. Eng. Aspects 241(1–3):15–25. doi:10.1016/j.colsurfa.2004.04.007
- Mumba, P.P., and R. Phiri. 2008. Environmental impact assessment of tobacco waste disposal. Int. J. Environ. Res. 2(3):225–30.
- Muniandy, L., F. Adam, A.R. Mohamed, and E.P. Ng. 2014. The synthesis and characterization of high purity mixed microporous/mesoporous activated carbon from rice husk using chemical activation with NaOH and KOH. Microporous Mesoporous Mater. 197:316–23. doi:10.1016/j.micromeso.2014.06.020
- Oh, G.H., C.H. Yun, and C.R. Park. 2003. Role of KOH in the one-stage KOH activation of cellulosic biomass. Carbon Lett. 4(4):180–4.
- Ozdemir, I., M. Şahin, R. Orhan, and M. Erdem. 2014. Preparation and characterization of activated carbon from grape stalk by zinc chloride activation. Fuel Process. Technol. 125:200–6. doi:10.1016/j.fuproc.2014.04.002
- Saka, C. 2012. BET, TG–DTG, FT-IR, SEM, iodine number analysis and preparation of activated carbon from acorn shell by chemical activation with ZnCl2. J. Anal. Appl. Pyrol. 95:21–4. doi:10.1016/j.jaap.2011.12.020
- Sedghi, S., S.H. Madani, C. Hu, A. Silvestre-Albero, W. Skinner, P. Kwong, P. Pendleton, R.J. Smernik, F. Rodríguez-Reinoso, and M.J. Biggs. 2015. Control of the spatial homogeneity of pore surface chemistry in particulate activated carbon. Carbon 95:144–9. doi:10.1016/j.carbon.2015.08.019
- Sevilla, M., Maciá-Agulló, J.A., Fuertes, A.B. 2011. Hydrothermal carbonization of biomass as a route for the sequestration of CO2: Chemical and structural properties of the carbonized products. Biomass Bioenergy 35(7):3152–9. doi:10.1016/j.biombioe.2011.04.032
- Sung, Y.J., and Y.B. Seo. 2009. Thermogravimetric study on stem biomass of Nicotiana tabacum. Thermochim. Acta 486(1–2):1–4. doi:10.1016/j.tca.2008.12.010
- Torrellas, S.Á., R. García-Lovera, N. Escalona, C. Sepúlveda, J.L. Sotelo, and J. García. 2015. Chemical-activated carbons from peach stones for the adsorption of emerging contaminants in aqueous solutions. Chem. Eng. J. 279:788–98. doi:10.1016/j.cej.2015.05.104
- Uçar, S., M. Erdem, T. Tay, and S. Karagöz. 2009. Preparation and characterization of activated carbon produced from pomegranate seeds by ZnCl2 activation. Appl. Surf. Sci. 255(21):8890–6. doi:10.1016/j.apsusc.2009.06.080
- Wang, L., H. Zhang, G. Cao, W. Zhang, H. Zhao, and Y. Yang. 2015. Effect of activated carbon surface functional groups on nano-lead electrodeposition and hydrogen evolution and its applications in lead-carbon batteries. Electrochim. Acta 186:654–63. doi:10.1016/j.electacta.2015.11.007
- Wang, Z.M., H. Kanoh, K. Kaneko, G. Lu, and D. Do. 2002. Structural and surface property changes of macadamia nut-shell char upon activation and high temperature treatment. Carbon 40(8):1231–9. doi:10.1016/S0008-6223(01)00286-X
- Yang, J., and K. Qiu. 2011. Development of high surface area mesoporous activated carbons from herb residues. Chem. Eng. J. 167(1):148–54. doi:10.1016/j.cej.2010.12.013
- Yao, X., L. Li, H. Li, and S. He. 2014. A new method for preparing hydrophilic-activated carbon through ester hydrolysis in an alkaline environment. J. Mater. Sci. 49(14):4807–15. doi:10.1007/s10853-014-8180-9
- Zhang, J., Z. Zhong, D. Shen, J. Zhao, H. Zhang, M. Yang, and W. Li. 2011. Preparation of bamboo-based activated carbon and it’s application in direct carbon fuel cells. Energy Fuels 25(5):2187–93. doi:10.1021/ef200161c
- Zhou, J.H., Z.J. Sui, J. Zhu, P. Li, D. Chen, Y.C. Dai, and W.K. Yuan. 2007. Characterization of surface oxygen complexes on carbon nanofibers by TPD, XPS and FT-IR. Carbon 45(4):785–96. doi: 10.1016/j.carbon.2006.11.019