ABSTRACT
Manure-based soil amendments (herein “amendments”) are important fertility sources, but differences among amendment types and management can significantly affect their nutrient value and environmental impacts. A 6-month in situ decomposition experiment was conducted to determine how protection from wintertime rainfall affected nutrient losses and greenhouse gas (GHG) emissions in poultry (broiler chicken and turkey) and horse amendments. Changes in total nutrient concentration were measured every 3 months, changes in ammonium (NH4+) and nitrate (NO3−) concentrations every month, and GHG emissions of carbon dioxide (CO2), methane (CH4), and nitrous oxide (N2O) every 7–14 days. Poultry amendments maintained higher nutrient concentrations (except for K), higher emissions of CO2 and N2O, and lower CH4 emissions than horse amendments. Exposing amendments to rainfall increased total N and NH4+ losses in poultry amendments, P losses in turkey and horse amendments, and K losses and cumulative N2O emissions for all amendments. However, it did not affect CO2 or CH4 emissions. Overall, rainfall exposure would decrease total N inputs by 37% (horse), 59% (broiler chicken), or 74% (turkey) for a given application rate (wet weight basis) after 6 months of decomposition, with similar losses for NH4+ (69–96%), P (41–73%), and K (91–97%). This study confirms the benefits of facilities protected from rainfall to reduce nutrient losses and GHG emissions during amendment decomposition.
Implications: The impact of rainfall protection on nutrient losses and GHG emissions was monitored during the decomposition of broiler chicken, turkey, and horse manure-based soil amendments. Amendments exposed to rainfall had large ammonium and potassium losses, resulting in a 37–74% decrease in N inputs when compared with amendments protected from rainfall. Nitrous oxide emissions were also higher with rainfall exposure, although it had no effect on carbon dioxide and methane emissions. Overall, this work highlights the benefits of rainfall protection during amendment decomposition to reduce nutrient losses and GHG emissions.
Introduction
Solid manure-based soil amendments containing urine, feces, bedding, and/or feed (herein “amendments”) are valuable nutrient sources in farming systems, but optimal management is critical to maximize benefits (Lesschen et al. Citation2011). For example, minimizing nutrient losses from leaching, runoff, and gaseous emissions during decomposition is key to maximizing nutrient value and reducing environmental impacts (Hao et al. Citation2001). However, amendment decomposition—during storage and/or composting—contributes a sizeable yet variable portion of total greenhouse gas (GHG) emissions from livestock production (Lesschen et al. Citation2011). For example, nitrous oxide (N2O) and methane (CH4) emissions during storage can account for up to 5% and 10% of total amendment nitrogen (N) and carbon (C), respectively (Chadwick et al. Citation2011). Furthermore, the contribution to the global warming potential (GWP) originating from solid amendment decomposition is typically greater for CH4 than for N2O (Hao et al. Citation2001), although some studies have found the opposite (Amon et al. Citation2001). Therefore, better understanding how the nutrient value and GHG emissions evolve during different amendment decomposition conditions is key to optimizing management practices (Amon et al. Citation2001).
The nutrient value of amendments varies during decomposition as a function of nutrient and C cycling. Nitrogen concentrations often decrease during storage and composting due to gaseous losses: ammonia (NH3) volatilization, N2O emitted during nitrification and denitrification, and nitrogen gas (N2) produced via denitrification (Akiyama et al. Citation2004; Chadwick et al. Citation2011). In contrast, nutrients without a dominant gas phase—e.g., phosphorus (P), potassium (K)—will be retained if leaching losses are minimized (Eghball et al. Citation2002). Carbon losses, where C is lost as carbon dioxide (CO2) primarily during aerobic decomposition and CH4 during anaerobic decomposition (Chowdhury, De Neergaard, and Jensen Citation2014), also affect nutrient concentrations. Ultimately, amendment nutrient concentrations may increase if nutrient losses are proportionally lower than C losses (Hao, Chang, and Larney Citation2004). The fraction of N found as plant-available ammonium (NH4+) and nitrate (NO3−) may also vary (Castellanos and Pratt Citation1981; Li and Xin Citation2010), affecting the nutrient value of amendments after decomposition (Nelson and Janke Citation2007).
Rainfall exposure during amendment decomposition can significantly impact nutrient value and GHG emissions, especially if rainfall is high. For example, storing amendments during the wintertime in areas subject to cool and wet winters can expose amendments to significant rainfall, depending on storage conditions, where field-stored solid amendments may not be protected from rainfall despite regulatory requirements. This exposure to rainfall can be problematic, as it may increase risks of nutrient and pathogen losses to groundwater and surface waters via runoff and leaching (Amon et al. Citation2001). High nutrient losses will also lower amendment nutrient concentrations and nutrient value, increasing application rates required to reach a given nutrient input target. Furthermore, rainfall exposure will increase moisture content and reduce oxygen availability, affecting GHG emissions (El Kader et al. Citation2007). Anoxic conditions should increase CH4 emissions by favoring methanogenesis over CH4 oxidation, reduce C mineralization rates, and potentially increase denitrification rates and N2O emissions (Amon et al. Citation2001). In contrast, lower oxygen availability may reduce N2O emissions by inhibiting nitrification and/or favoring complete denitrification, although the net effect of oxygenation on N2O emissions remains uncertain (Chadwick et al. Citation2011; El Kader et al. Citation2007). This may be due to differences in experimental conditions (e.g., in situ vs. laboratory) affecting how amendment moisture content impacts the nutrient value and emissions from individual GHGs differently (Chowdhury, De Neergaard, and Jensen Citation2014). There is therefore a need to evaluate changes in nutrient value and GHG emissions simultaneously in situ to identify potential trade-offs.
Properties that vary substantially among amendments could also impact the response of nutrient cycling, mineralization rates, and GHG emissions to rainfall exposure (Li et al. Citation2012). Poultry amendments are typically enriched in labile C and nutrients compared with beef cattle and horse amendments, although the latter could behave differently than commonly studied beef cattle and dairy amendments (Castellanos and Pratt Citation1981; Moreno-Caselles et al. Citation2002; Nelson and Janke Citation2007). Ultimately, greater nutrient concentrations in poultry amendments result in a larger potential for nutrient losses, especially when exposed to rainfall, although this may be affected by differences in animal physiology and feeding regimes, e.g., between chicken and under-studied poultry amendments such as turkey manure (Moreno-Caselles et al. Citation2002; Nicholson, Chambers, and Smith Citation1996). Finally, the type of bedding found in amendments may affect nutrient cycling and GHG emissions through changes in particle size and oxygen availability (Monteny, Bannink, and Chadwick Citation2006; Van der Weerden, Luo, and Dexter Citation2014).
The main objective of this study was to determine how protecting amendments from wintertime rainfall during decomposition affected nutrient losses, the nutrient value, and GHG emissions from broiler chicken (herein “broiler”), turkey, and horse amendments. Our work focused on British Columbia, Canada, an area where farmers are encouraged to store amendments protected from rainfall during the wet and cool winters until application during the subsequent spring, prior to the production season. We wanted to identify how typical amendments (manure combined with bedding and/or spilled feed) with different properties responded to rainfall exposure over time and evaluate potential trade-offs between changes in nutrient concentrations and GHG emissions. We hypothesized that nutrient losses and GHG emissions would be highest with wintertime rainfall exposure, especially in nutrient-rich, fast-mineralizing poultry amendments.
Materials and methods
Experimental design
Eighteen amendment piles were installed at the University of British Columbia (UBC) farm (Vancouver, Canada), using fresh broiler, turkey, and horse amendments. Bedding materials varied among amendments and were representative of commonly used materials in animal operations in the area: sawdust in broiler, wood chips in turkey, and straw in horse. Thus, comparisons made in this study do not distinguish between animal waste per se (feces and urine) and bedding material. Fresh poultry amendments were collected from large, individual operations—six two-story barns totaling 200,000 broiler chickens and six single-story barns totaling 30,000 turkeys—that represent typical animal density, diet, and waste management practices for the area. Horse amendment was collected from a small farm—one barn totaling 20 horses—representative of horse operations in the area.
Amendment piles were installed on August 25, 2015, in bays made of pallets covered in permeable landscape fabric on three sides, with the front side left open for sampling (Supplemental Material, Figure S1). At the onset of the experiment, amendment piles were 1.25 m wide, 0.70 m high, 1.05 m deep at the bottom of the pile and 0.85 m deep at the top of the pile, for a total volume of 0.7 m3 and a total bottom area of 1.3 m2. At the end of the experiment, the width and depth of amendment piles were relatively unchanged, and the height decreased to 0.46 m on average (range: 0.10–0.68 m), resulting in a mean volume of 0.44 m3 (range: 0.05–0.63 m3). Covered piles were protected from rainfall with a 0.64-cm-thick plywood sheet that allowed constant airflow. Therefore, covered and uncovered piles were representative of permanent storage protected from rainfall and unprotected field-stored amendments, respectively. Amendment piles were established using a factorial design, with three piles per combination of amendment type with protection (or not) from wintertime rainfall arranged in three randomized blocks.
Amendment piles were monitored until March 1, 2016, during the cool and wet winter experienced in the Vancouver area (; Figure S2). According to regulation in British Columbia, field-stored piles must be covered for 6 months in this area—from October 1 until April 1—because total average precipitation typically exceeds 600 mm during this period. Nevertheless, amendments are often exposed to wintertime rainfall when they are stored temporarily before application. This highlights the need to quantify the agronomic and environmental impacts of wintertime rainfall exposure prior to spring application (i.e., from April onwards) that is typical for this area.
Table 1. Weather conditions during the experimental period, including cumulative rainfall computed for the 2015–2016 winter and 10-yr averages for both temperature and rainfall.
Nutrient sampling and analyses
Amendment piles were sampled at the onset of the experiment (August 28, 2015) and at the end of each month (last sample: February 28, 2016) for nutrient analyses. We sampled piles (depth: 45 cm) using an auger inserted at a perpendicular angle from the sloping front surface of the piles (Figure S1). We collected one core (785 mL) from the center of each pile to minimize the disturbance created by sampling, excluding the top crust layer (5 cm) and large pieces (>5 cm) of bedding from the core. Samples were kept frozen (−18 °C) until analysis. We excluded the bottom of amendment piles where nutrients leaching from the top could have accumulated during the experiment. We used this sampling approach consistently throughout the study to ensure that we would capture nutrient movement and loss from the main volume of amendment piles.
An external laboratory (A&L Canada, London, Ontario, Canada) measured total %N, %P, and %K at the onset of the experiment, and after 3 and 6 months of storage, and total C:N at the beginning and end of the experiment. Samples were dried (85 °C for 24 hr) and ground prior to total-nutrient analyses by combustion (total C and N) or nitric-hydrochloric acid digestion followed by quantification via inductively coupled plasma optical emission spectrometry (ICP-OES) (P and K).
We measured NH4+ –N and NO3− –N concentrations on a monthly basis by extracting duplicate 0.5-g samples of thawed, moist amendments with 30 mL of 2 M potassium chloride for 30 min on a reciprocal shaker. Samples were centrifuged (5000 rpm for 5 min), filtered (Fisherbrand Q2 filters; Fisher Scientific, Ottawa, Ontario, Canada), and analyzed for NH4+–N (Weatherburn Citation1967) and NO3−–N (Doane and Horwath Citation2003) by colorimetry.
All nutrient analyses are expressed as concentrations per unit of dry weight, unless specified otherwise. Moisture content was measured on samples dried at 85°C for 24 hr.
Greenhouse gas measurements
We measured N2O, CO2, and CH4 fluxes from individual piles weekly (August to December 2015) or biweekly (January to March 2016), quantifying fluxes from individual piles once per sampling day. At the surface of each pile, we inserted a 15-cm-long polyvinyl chloride (PVC) collar with a 20-cm inner diameter, leaving 5–10 cm above the surface that resulted in a headspace varying from 1.2 to 3.4 L, with an average volume of 2.2 L.
We measured gas concentrations—N2O, CO2, CH4, NH3, and water—by cavity ring-down spectroscopy (CRDS) using a Picarro G2508 analyzer (Picarro Inc., Santa Clara, CA, USA), using a non-steady-state chamber system. Gas accumulation in the headspace was measured during a 1-min enclosure, allowing for accurate quantification of gas accumulation while minimizing NH3 interference with N2O occurring with this instrument when NH3 is >2 ppm (i.e., NH3 interference was delayed for 1 min after the enclosure period started). We used a 1-min enclosure time for the whole study for consistency, despite ammonia concentrations falling below 0.5 ppm after 2 months. Air was recirculated at a rate of 2 L min−1 using a vacuum pump during measurements, resulting in a near-complete sampling of the full headspace in most cases. We flushed the system with outside air (10 L min−1 for 5 min) between measurements to reduce NH3 concentrations to background levels.
GHG fluxes were computed with MATLAB version R2014a (Mathworks Inc. Citation2014), using the following formula:
where F is the flux (µmol m−2 sec−1), ρ is the molar density (mol m−3) of dry air (i.e., corrected for the average mixing ratio of water measured during measurements), S is the slope of GHG accumulation within the headspace (µmol mol−1 sec−1), V is the headspace volume (m−3), and A is the headspace area (m−2). The slope of gas accumulation was measured by linear regression, and slopes were utilized without further modification if the coefficient of determination (R2) was greater than 0.75. Slopes with R2 < 0.75 were visually examined and converted to zero (if no quantifiable gas accumulation in the headspace) or removed (when measurement issues were observed).
Cumulative GHG fluxes were computed using linear interpolation between measurements (Gana et al. Citation2016), using the following formula:
where F(t1) and F(t2) are fluxes at time t1 and t2, and t1 < t < t2.
We also computed the global warming potential (GWP) on a 100-yr horizon by converting all GHG fluxes in CO2-equivalents, using conversion ratios of 25 for CH4 and 298 for N2O (Intergovernmental Panel on Climate Change [IPCC] Citation2007). Cumulative GHG fluxes were computed for the first month, the first 3 months, and the full length of the experiment.
Statistical analyses
We analyzed data by performing a three-way analysis of variance (ANOVA) for every variable, using amendment type, rainfall exposure, and sampling date as fixed factors, and block as a random factor. However, because every three-way ANOVA had at least one significant interaction term including sampling date, we decided to use one two-way factorial ANOVA per individual sampling date, with amendment type and rainfall exposure as fixed factors and block as a random factor, followed by a post hoc Tukey honestly significant difference (HSD) test. We verified the conditions of normality of residuals (Shapiro-Wilk test) and homogeneity of variances (Levene test) and applied appropriate data transformations (in order, square root, log, rank) to ensure the conditions to perform the ANOVAs were met. We used a significance threshold of P < 0.05 for main effects and results from Tukey’s test but explored interactions when the significance of the interaction term was 0.05 < P < 0.1. All statistical analyses were conducted in R (R Core Team Citation2013).
Results
Nutrient concentrations
Moisture content was higher in uncovered relative to covered poultry amendments after 3 (63–69% vs. 31–32%) and 6 (73–75% vs. 33% for turkey and 53% for broiler) months, with a larger moisture increase in broiler relative to turkey amendment (Table S1). This was likely caused by a larger loss in volume for broiler amendment in the last 3 months of the experiment, resulting in greater relative exposure to rainfall and runoff in the uncovered front side used for sampling. Uncovered horse amendment piles had higher moisture content after 3 (72% vs. 61%) and 6 (73% vs. 61%) months.
Rainfall exposure did not have a significant effect on %N after 3 months (, ). After 6 months of rainfall exposure, %N was 23–36% lower in uncovered compared with covered poultry amendments, whereas amendment C:N was 35–60% greater in uncovered poultry amendments (). In contrast, rainfall exposure did not affect horse amendment %N, although %N increased and C:N decreased with time, regardless of rainfall exposure. Nitrogen concentrations were highest in broiler and lowest in horse, whereas C:N followed the opposite trend.
Table 2. Statistical results for two-way ANOVAs and Tukey post hoc tests computed on total nutrient concentrations and ratios.
Table 3. Variations in C:N and N:P ratios (mean ± standard deviation) at the beginning and end of the experiment.
Figure 1. Variations in amendment total nitrogen, phosphorus, and potassium concentrations (mean ± standard deviation), reported on a dry-matter basis. Refer to for details on statistical results for ANOVAs and post hoc Tukey tests.
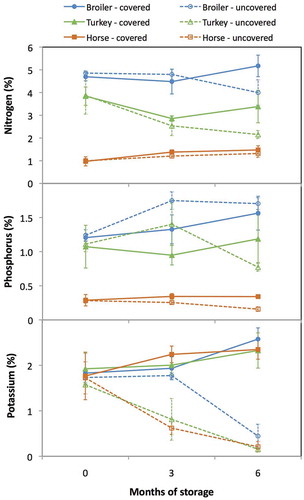
After 3 months, rainfall exposure increased %P by 24–32% in uncovered relative to covered poultry amendments, but it did not affect %P in horse amendment (, ). After 6 months, rainfall exposure decreased %P by 35–65% in horse and turkey amendments, but it had no effect on broiler amendment. Initially, %P was highest and lowest in broiler and horse amendments, respectively, whereas poultry amendments were higher than horse amendment after 3 months. After 6 months, broiler amendment had higher %P than turkey and horse amendments in uncovered conditions, whereas poultry amendments were higher than horse amendment in covered conditions.
Amendment N:P was twice as high in uncovered as compared with covered piles for horse amendment after 6 months, whereas rainfall exposure had no effect on poultry amendments (, ). Amendment N:P decreased in poultry amendments and increased in horse amendment over time.
Potassium concentrations decreased by 59–72% in uncovered relative to covered piles for turkey and horse amendments after 3 months of rainfall exposure, and by 83–93% for all amendments after 6 months (, ). There were no significant differences in %K among amendment types at the beginning and end of the experiment, whereas %K was higher in broiler amendment in uncovered conditions after 3 months.
Ammonium concentrations were quite variable and decreased with time in uncovered poultry amendments, whereas concentrations were more stable in covered piles (, ). Ammonium concentrations were 39–75% higher in uncovered turkey piles during the first 2 months, but less than 35% of values found in covered piles in the last 3 months. In contrast, NH4+-N concentrations were 30–90% higher in uncovered broiler amendment for the first 4 months and dropped to less than 35% of values found in covered piles only in the sixth month. In horse amendment, NH4+ concentrations decreased by 80–85% after the first month and remained low thereafter, with no effect of rainfall exposure. Overall, NH4+-N concentrations were higher in poultry than in horse amendments.
Table 4. Statistical results for two-way ANOVAs and Tukey post hoc tests computed on ammonium (NH4+-N) and nitrate (NO3−-N) concentrations.
Figure 2. Variations in amendment ammonium (NH4+-N) and nitrate (NO3−-N) concentrations (mean ± standard deviation), reported on a dry-matter basis. Refer to for details on statistical results for ANOVAs and post hoc Tukey tests.
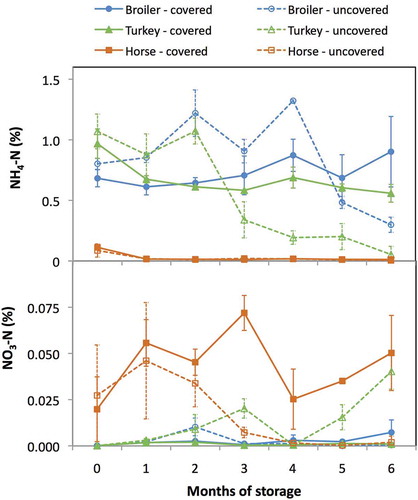
Nitrate concentrations were minimal for all amendments (<0.1%) but were higher in horse amendment for covered piles during the entire experiment (, ). In horse amendment, NO3−-N concentrations in uncovered piles were 90–99% lower relative to covered piles in the last 4 months. In contrast, NO3−-N concentrations were an order of magnitude higher in uncovered relative to covered turkey amendment piles in the last 2 months. Horse amendment had more NO3−-N than poultry amendments for the first 2 months in uncovered piles. In contrast, turkey amendment had more NO3−-N than horse and broiler amendments in the last 2 months in uncovered piles.
Greenhouse gas emissions
Nitrous oxide fluxes peaked during the first (horse) or second and third (poultry) months (). Cumulative N2O fluxes were 2–2.5-fold higher in uncovered relative to covered piles for poultry amendments after 3 months, and 45–95% (poultry) or 11% (horse) higher in uncovered relative to covered piles after 6 months (Figure S3). Cumulative N2O fluxes were higher in horse amendment after 1 month, but higher in poultry amendments after 3 (3–10-fold difference) and 6 (6–10-fold difference) months.
Figure 3. Variations in fluxes (mean ± standard deviation) of nitrous oxide (N2O), carbon dioxide (CO2), and methane (CH4), expressed as daily fluxes (left) or cumulative fluxes after 6 months (right). For ANOVA terms: ***P < 0.001; **P < 0.01; *P < 0.05. Colored plus signs (+) indicate a statistically significant difference (P < 0.05) between covered and uncovered piles for that specific amendment. Letters indicate a statistically significant difference among amendments, regardless of rainfall exposure (i.e., interaction terms were not significant).
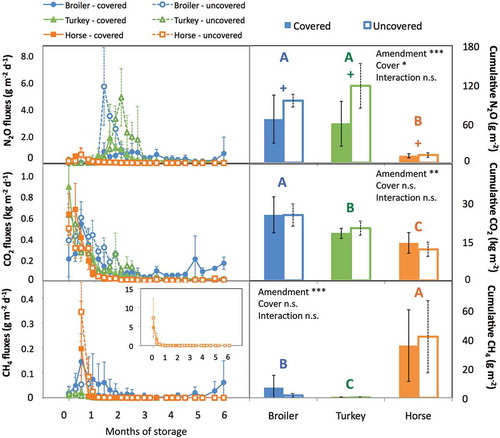
Carbon dioxide fluxes were highest at the beginning of the experiment in all conditions and decreased thereafter, although CO2 fluxes increased in covered broiler amendment during the last month (). Rainfall exposure did not affect cumulative CO2 fluxes for any of the amendment at any time point (Figure S3). Cumulative CO2 fluxes were similar among amendments after 1 month, 70% higher in broiler than in horse amendment in uncovered piles after 3 months, and lowest in horse amendment, intermediate in turkey, and highest in broiler (70–110% higher than horse amendment) after 6 months.
Methane fluxes declined during the experiment, especially in horse amendment, although CH4 fluxes increased in covered broiler amendment during the last month (). Similar to CO2, rainfall exposure did not affect CH4 fluxes (Figure S3). Cumulative CH4 fluxes were always higher in horse amendment, by 3-fold to 2 orders of magnitude, and cumulative CH4 fluxes were 5–50-fold higher in broiler versus turkey amendment after 6 months.
The cumulative GWP was similar among amendments after 1 month, where CO2 fluxes accounted for over 70% (horse) or 90% (poultry) of GWP (; Table S2). After 3 months of rainfall exposure, cumulative GWP was 70–90% higher in uncovered relative to covered poultry amendments (only significant for broiler), whereas poultry amendments were 50–60% (covered) or 3-fold (uncovered) higher than horse amendment (only significant for uncovered piles). Rainfall exposure did not affect cumulative GWP after 6 months, but poultry amendments were 2–3-fold higher than horse amendment. Nitrous oxide had the largest contribution to GWP in uncovered poultry piles after 3 and 6 months; otherwise, CO2 was the main contributor to GWP; CH4 always accounted for less than 10% of cumulative GWP.
Figure 4. Cumulative fluxes (mean ± standard deviation) of nitrous oxide (N2O), carbon dioxide (CO2), and methane (CH4) expressed as CO2-equivalents after 1, 3, and 6 months in covered (Cov) and uncovered (Unc) conditions. For ANOVA terms: **P < 0.01; *P < 0.1. Colored plus signs (+) indicate a statistically significant difference (P < 0.05) between covered and uncovered piles for that specific amendment. Letters indicate a statistically significant difference among amendments at that specific time, where solid letters are used if there is no interaction (i.e., after 6 months) and white-centered letters are used when differences are found only in uncovered piles (i.e., after 3 months).
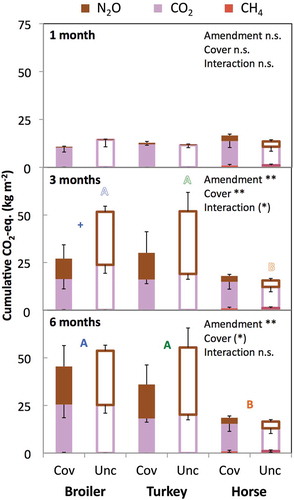
Discussion
Effects of rainfall exposure
Rainfall exposure increased C:N in uncovered poultry amendments, which should reduce N mineralization rates, percentage of plant-available N, and N fertilization value (Gale et al. Citation2006) as compared with covered poultry amendments that experienced smaller changes in C:N. Nitrogen availability may decrease further if rainfall exposure preferentially depletes easily degradable C and N in uncovered conditions (Eghball et al. Citation2002). In contrast, C:N decreased in horse amendment, suggesting that decomposition may increase the nutrient value of this under-studied amendment. However, a final C:N above 20:1 suggests that N immobilization would be expected after field application (Gale et al. Citation2006), regardless of rainfall exposure.
Besides N, uncovered piles had high losses of other nutrients, especially K. Because our sampling approach focused on the center of amendment piles, it is possible that nutrients at the top moved to the bottom of piles where they accumulated instead of leaching out. Regardless of the ultimate fate of nutrients, the response to rainfall exposure varied between poultry amendments. Indeed, nutrient losses in uncovered conditions were either nondetectable (P) or delayed (K and NH4+–N) in broiler as compared with turkey amendment. Given the similar initial nutrient concentrations found in broiler and turkey amendments, greater nutrient retention in broiler amendment could be due to the sawdust bedding increasing the area of reactive surfaces—and potentially sorption capacity—compared with the turkey’s wood chip bedding. Thus, bedding materials could be selected to minimize nutrient losses when amendments are exposed to rainfall, although this should be confirmed in factorial experiments where bedding material varies independently of manure type. Furthermore, sampling nutrient concentrations at different depths in amendment piles and collecting leachate would allow determining if nutrients moved downward or were fully leached.
Rainfall exposure also increased N2O emissions, especially in poultry amendments during the first 3 months of decomposition. Greater moisture content often increases N2O emissions (Akiyama et al. Citation2004), although El Kader et al. (Citation2007) found the opposite effect of moisture on N2O emissions, which they linked to low oxygen availability. High N2O emissions were coupled with low CH4 emissions in this study, suggesting that oxygen was sufficiently available to prevent complete denitrification (Akiyama et al. Citation2004; Chowdhury, De Neergaard, and Jensen Citation2014; Li et al. Citation2012), and similarly inhibit methanogenesis and/or stimulate CH4 oxidation. Higher moisture content and lower oxygen availability in our uncovered in situ piles did not affect CH4 (or CO2) emissions significantly, unlike in laboratory (Li and Xin Citation2010) or in situ (Hao et al. Citation2001) studies. This suggests that low temperatures (below 15 °C) during the last 4 months were the main driver of carbon decomposition rates in our study, similar to Chadwick et al. (Citation2011) and Li et al. (Citation2012), although differences with previous studies could also be caused by a difference in experimental conditions (in situ vs. laboratory). Furthermore, low temperatures could explain the small contribution of CH4 emissions to GWP measured here with poultry and horse amendments compared with previous studies focusing on cattle and dairy solid amendments (Amon et al. Citation2001; Hao et al. Citation2001).
Effects of amendment types
Poultry amendments were enriched in N, NH4+–N, and P relative to horse amendment, similar to previous laboratory incubation studies (Castellanos and Pratt Citation1981; Chae and Tabatabai Citation1986; Gagnon and Simard Citation1999). Higher NH4+ –N concentrations favor NH3 and N2O emissions, however (Akiyama et al. Citation2004), and we observed greater cumulative N2O emissions and contribution to GWP in poultry relative to horse amendment after 3 and 6 months, especially when exposed to rainfall. Our in situ measurements are thus consistent with the higher N2O emissions Chowdhury, De Neergaard, and Jensen (Citation2014) measured when amendment C:N decreased in small-scale laboratory composters. Low C:N in poultry amendments should increase mineralization rates (Castellanos and Pratt Citation1981; Gale et al. Citation2006), consistent with the higher CO2 emissions (and C mineralization) we observed in poultry relative to horse amendments. In contrast, CH4 emissions were greater in horse amendment but were too low to impact C losses significantly, similar to previous studies focusing on solid beef cattle amendments (Hao, Chang, and Larney Citation2004). Thus, our in situ results on the under-studied horse amendment are generally consistent with previous studies on high C:N amendments, especially for GHG.
Turkey and broiler amendments behaved similarly for many (e.g., N2O fluxes) but not all variables (e.g., %N, NH4+), unlike in Moreno-Caselles et al. (Citation2002) and Nicholson, Chambers, and Smith (Citation1996) where broiler and turkey amendments behaved similarly for all properties. Broiler amendment emitted more CO2 and CH4 than turkey amendment, which could be due to differences in bedding material (Chadwick et al. Citation2011; Monteny, Bannink, and Chadwick Citation2006). Wood chips used in this specific turkey amendment should mineralize more slowly and emit less CO2 than sawdust (Hao, Chang, and Larney Citation2004), and it could reduce CH4 emissions via lower bulk density (147 vs. 193 kg m−3) and greater oxygen availability. These results, if validated in factorial experiments where bedding material varies independently of manure type, would confirm the importance of bedding material for GHG emissions (Li et al. Citation2012; Monteny, Bannink, and Chadwick Citation2006; Van der Weerden, Luo, and Dexter Citation2014). Alternatively, other differences between broiler and the under-studied turkey amendment (e.g., due to physiology) may have been the main driver of higher GHG emissions in the former.
Ultimately, the effects of rainfall protection varied among amendment types for periods of 3 months or less. Short-term exposure to rainfall resulted in limited benefits for poultry amendments (increases in %P and NH4+–N), although the increase in GWP measured between 1 and 3 months of storage indicates potential trade-offs between higher GHG emissions and higher nutrient availability. In contrast, rainfall exposure had small effects in horse amendment given its low nutrient losses (except for K) and GWP. After 6 months, however, rainfall protection was always beneficial for the three amendments under study, with higher nutrient value and lower N2O emissions.
Implications for management
Exposing amendments to rainfall reduced their nutrient value after 3 and 6 months of field storage due to decreasing nutrient concentrations and increasing water content. Nutrient concentrations (in % wet weight) in uncovered piles decreased by 28–81% relative to those found in covered piles (except for NH4+–N in horse amendment) after 3 months, compared with a decrease of 37–97% after 6 months (Table S1). Thus, after 6 months of decomposition, total N applied with a 20 Mg ha−1 amendment application (wet weight) would decrease from 447–486 kg N ha−1 when poultry amendments are protected from rainfall to 117–200 kg N ha−1 without rainfall protection. However, the decrease in N applied may be underestimated for broiler amendment because the higher than expected moisture content in covered piles after 6 months likely decreased the difference between covered and uncovered amendments. For horse amendment, total N applied would decrease from 113 to 72 kg N ha−1. In addition, higher C:N resulting from rainfall exposure should reduce the fraction of total N that is plant available (Gale et al. Citation2006). Phosphorus and K input rates would also decrease, e.g., K inputs falling from 180–308 kg K ha−1 when amendments are protected from rainfall to less than 25 kg K ha−1 when amendments are exposed to rainfall. Ultimately, lower nutrient value should increase transport and spreading costs for a given nutrient application rate, or decrease yields via suboptimal application rates if amendment supply is limited. The lower N:P ratio in uncovered poultry amendments (especially broiler) will also increase P overfertilization and the risk of P losses when trying to match crop N demand with amendments (Nelson and Janke Citation2007), where the decrease in N:P in broiler amendment may have been underestimated due to the greater than expected moisture content in covered piles.
Exposing poultry amendments to rainfall increased N2O fluxes and GWP, potentially reducing a farmer’s capacity to mitigate GHG emissions. In contrast, protection from rainfall did not significantly affect CO2 and CH4 fluxes during in situ decomposition, unlike previous laboratory studies reporting higher GHG emissions when moisture content increases (Li and Xin Citation2010), highlighting the value of in situ studies on GHG emissions. Furthermore, protection from wintertime rainfall minimized nutrient losses and maintained the nutrient value of amendments, supporting policies that encourage rainfall protection during wintertime storage and composting. Otherwise, amendments exposed to rainfall would need to be applied at higher rates to reach a given fertility target, increasing fuel usage and GWP associated with amendment management, although fuel consumption is often a minor component of this GWP (Hao et al. Citation2001).
Conclusion
Exposing amendments to wintertime rainfall generally resulted in important nutrient losses (especially K and NH4+–N) and higher N2O emissions (especially in poultry amendments), but had no effect on CO2 or CH4 emissions. Thus, the effects of rainfall exposure were generally negative for the nutrient value of amendments as well as for environmental impacts, especially after 6 months of rainfall exposure. This was true for all amendments, including the under-studied turkey and horse amendments, highlighting the benefits of in situ experiments to determine the effects of changes in moisture content on nutrient cycling and GHG emissions.
Our results confirm the value of protecting amendments from wintertime rainfall during decomposition and highlight the need for farmers to have access to adequate storage facilities. Our results also suggest that bedding materials with a high retention capacity could mitigate nutrient losses, especially for ions with a low sorption strength (e.g., NH4+–N, K+), although this would need to be confirmed in future experiments.
Supplemental Document
Download PDF (1 MB)Acknowledgment
The authors thank the University of British Columbia farm staff for logistical support while establishing the experiment, and Judy Chang, Joanna Chenghy, Mark Henry, Amy Norgaard, Linghui Wang, and Tom Zochowski for assistance in the field and in the laboratory.
Supplemental data
Supplemental data for this paper can be access on the publisher’s Web site.
Additional information
Funding
Notes on contributors
Gabriel Maltais-Landry
Gabriel Maltais-Landry is an assistant professor in the Department of Soil and Water Sciences, University of Florida, in Gainesville, Florida, USA.
Katarina Neufeld
Katarina Neufeld, Nicholas Grant, and Zoran Nesic are research assistants and Sean Smukler is an assistant professor in the Faculty of Land and Food Systems, University of British Columbia, in Vancouver, British Columbia, Canada.
David Poon
David Poon is the provincial soil specialist in the British Columbia Ministry of Agriculture, in Abbotsford, British Columbia, Canada.
References
- Akiyama, H., I.P. McTaggart, B.C. Ball, and A. Scott. 2004. N2O, NO, and NH3 emissions from soil after the application of organic fertilizers, urea and water. Water, Air, & Soil Pollution 156 (1):113–29. doi:10.1023/B:WATE.0000036800.20599.46.
- Amon, B., T. Amon, J. Boxberger, and C. Alt. 2001. Emissions of NH3, N2O and CH4 from dairy cows housed in a farmyard manure tying stall (housing, manure storage, manure spreading). Nutrient Cycling in Agroecosystems 60 (1–3):103–13. doi:10.1023/A:1012649028772.
- Castellanos, J.Z., and P.F. Pratt. 1981. Mineralization of manure nitrogen—Correlation with laboratory indexes. Soil Science Society of America Journal 45 (2):354–7. doi:10.2136/sssaj1981.03615995004500020025x.
- Chadwick, D., S.G. Sommer, R. Thorman, D. Fangueiro, L. Cardenas, B. Amon, and T. Misselbrook. 2011. Manure management: Implications for greenhouse gas emissions. Animal Feed Science and Technology 166–7 (Suppl. I):514–31. doi:10.1016/j.anifeedsci.2011.04.036.
- Chae, Y.M., and M.A. Tabatabai. 1986. Mineralization of nitrogen in soils amended with organic wastes. Journal of Environmental Quality 15 (2):193–8. doi:10.2134/jeq1986.00472425001500020021x.
- Chowdhury, M.A., A. De Neergaard, and L.S. Jensen. 2014. Potential of aeration flow rate and bio-char addition to reduce greenhouse gas and ammonia emissions during manure composting. Chemosphere 97:16–25. doi:10.1016/j.chemosphere.2013.10.030.
- Doane, T.A., and W.R. Horwath. 2003. Spectrophotometric determination of nitrate with a single reagent. Analytical Letters 36 (12):2713–22. doi:10.1081/AL-120024647.
- Eghball, B., B.J. Wienhold, J.E. Gilley, and R.A. Eigenberg. 2002. Mineralization of Manure Nutrients. Journal of Soil and Water Conservation 57 (6):470–73.
- El Kader, N.A., P. Robin, J.-M. Paillat, and P. Leterme. 2007. Turning, compacting and the addition of water as factors affecting gaseous emissions in farm manure composting. Bioresource Technology 98 (14):2619–28. doi:10.1016/j.biortech.2006.07.035.
- Gagnon, B., and R.R. Simard. 1999. Nitrogen and phosphorus release from on-farm and industrial composts. Canadian Journal of Soil Science 79 (3):481–89. doi:10.4141/s98-078.
- Gale, E.S., D.M. Sullivan, C.G. Cogger, A.I. Bary, D.D. Hemphill, and E.A. Myhre. 2006. Estimating plant-available nitrogen release from manures, composts, and specialty products. Journal of Environmental Quality 35 (6):2321–32. doi:10.2134/jeq2006.0062.
- Gana, C., Y. Nouvellon, N. Marron, J.L. Stape, and D. Epron. 2016. Sampling and interpolation strategies derived from the analysis of continuous soil CO2 flux. Journal Plant Nutritional Soil Sciences. doi:10.1002/jpln.201600133.
- Hao, X.Y., C. Chang, and F.J. Larney. 2004. Carbon, nitrogen balances and greenhouse gas emission during cattle feedlot manure composting. Journal of Environmental Quality 33 (1):37–44. doi:10.2134/jeq2004.3700.
- Hao, X.Y., C. Chang, F.J. Larney, and G.R. Travis. 2001. Greenhouse gas emissions during cattle feedlot manure composting. Journal of Environmental Quality 30 (2):376–86. doi:10.2134/jeq2001.302376x.
- Intergovernmental Panel on Climate Change. 2007. Climate change 2007: Synthesis report. Contribution of Working Groups I, II and III to the Fourth Assessment Report of the Intergovernmental Panel on Climate Change. Geneva, Switzerland: Intergovernmental Panel on Climate Change.
- Lesschen, J.P., M. Van Den Berg, H.J. Westhoek, H.P. Witzke, and O. Oenema. 2011. Greenhouse gas emission profiles of European livestock sectors. Animal Feed Science and Technology 166–67 (Suppl. I):16–28. doi:10.1016/j.anifeedsci.2011.04.058.
- Li, C., W. Salas, R. Zhang, C. Krauter, A. Rotz, and F. Mitloehner. 2012. Manure-DNDC: A biogeochemical process model for quantifying greenhouse gas and ammonia emissions from livestock manure systems. Nutient Cycling in Agroecosystems 93 (2):163–200. doi:10.1007/s10705-012-9507-z.
- Li, H., and H. Xin. 2010. Lab-scale assessment of gaseous emissions from laying-hen manure storage as affected by physical and environmental factors. Transactions of the ASABE 53 (2):593–604. doi:10.13031/2013.29574.
- Mathworks Inc. 2014. MATLAB (version R2014a). Natick, Massachusetts: The MathWorks Inc.
- Monteny, G.J., A. Bannink, and D. Chadwick. 2006. Greenhouse gas abatement strategies for animal husbandry. Agriculture, Ecosystems & Environment 112 (2–3, Suppl. I):163–70. doi:10.1016/j.agee.2005.08.015.
- Moreno-Caselles, J., R. Moral, M. Perez-Murcia, A. Perez-Espinosa, and B. Rufete. 2002. Nutrient value of animal manures in front of environmental hazards. Communicable Soil Science and Plant Analysis 33 (15–18):3023–32. doi:10.1081/CSS-120014499.
- Nelson, N.O., and R.R. Janke. 2007. Phosphorus sources and management in organic production systems. Horttechnology 17 (4):442–54.
- Nicholson, F.A., B.J. Chambers, and K.A. Smith. 1996. Nutrient composition of poultry manures in England and Wales. Bioresource Technology 58 (3):279–84. doi:10.1016/S0960-8524(97)86087-7.
- R Core Team. 2013. R: A language and environment for statistical computing. Vienna, Austria: R Foundation for Statistical Computing.
- Van der Weerden, T.J., J. Luo, and M. Dexter. 2014. Addition of straw or sawdust to mitigate greenhouse gas emissions from slurry produced by housed cattle: A field incubation study. Journal of Environmental Quality 43 (4):1345–55. doi:10.2134/jeq2013.11.0452.
- Weatherburn, M.W. 1967. Phenol-hypochlorite reaction for determination of ammonia. Analytical Chemistry 39 (8):971–74. doi:10.1021/ac60252a045.