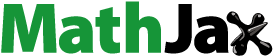
ABSTRACT
As a convenient method, the closed chamber method has been applied to determine gaseous emission fluxes from fully open animal feeding operations despite the measured fluxes being theoretically affected by deployment time, wind speed over the emitting surface and detected gas mass. This laboratory study evaluated the effects of deployment time (0 to 120 min) and external surface wind speed (ESWS) (0.00, 0.25, 0.50, 0.75, 1.00, 1.50 and 2.00 m sec-1) on the measurement accuracy of a 300 mm (diameter) × 400 mm (height) (D300×H400) closed chamber using methane (CH4), nitrous oxide (N2O) and sulfur hexafluoride (SF6) as reference gases. The results showed that the overall deviation ratio between the measured and reference CH4 fluxes ranged from 9.99 % to -37.32 % and the flux was overestimated in the first 20 min. The measured N2O and SF6 emissions were smaller than the reference fluxes using the chamber. N2O measurement accuracy decreased from -14.47 to -35.09% with deployment time extended to 120 min, while SF6 accuracy sharply increased in the first 40 min, with the deviation stabilizing at approximately -5.00%. CH4, N2O and SF6 measurements were significantly affected by deployment time and ESWS (P<0.05), and the interaction of those two factors greatly influenced CH4 and SF6 measurements (P<0.05). With the D300×H400 closed chamber, deployment times of 20 to 30 min and 10 to 20 min are recommended to measure CH4 and N2O, respectively, from the open operations of dairy farms under wind speeds lower than 2 m sec-1.
Implications: This study recommended the suitable deployment times and wind speeds for using a D300 × H400 closed chamber to measure CH4, N2O, and SF6 in an open system, such as a dairy open lot and manure stockpile, to help researchers and other related industry workers get accurate data for gas emission rate. Deployment times of 20 to 30 min and 10 to 20 min were recommended to measure CH4 and N2O emissions using the D300 × H400 closed chamber, respectively, from the open operations of dairy farms under wind speeds lower than 2 m sec−1. For the measurement of SF6, a typical tracer gas, a deployment of 70 to 90 min was suggested.
Introduction
Open lots are ancillary operations commonly used for keeping animals outside the barns, especially for dairy cows in China, where the bedding area, water tank, and open space for free activities are typically provided. Open dairy lots are important emission source of contaminant gases, including ammonia (NH3) and greenhouse gases (GHGs), to the atmosphere because of the animals and manure accumulation on it. It is still challenging to directly measure the emissions from lots, due to their fully open characteristic, relatively low gas flux, and interference with other emissions sources such as barns and cows (Ding et al. Citation2016a; Citation2016b).
Several techniques, including flux chambers, tracer gas, and micrometeorological methods, have been applied to quantify NH3 and GHGs emissions from open sources (Laubach et al. Citation2013; Lovanh, Warren, and Sistani Citation2010; McGinn et al. Citation2011; Misselbrook et al. Citation2001; Spiehs et al. Citation2012). Tracer gas and micrometeorological methods are considered ideal techniques; however, these methods either require expensive equipment (Laubach et al. Citation2013; McGinn et al. Citation2011) or are typically influenced by the emission sources, including wind profile, and by the detection errors of low emission gases (Harper, Denmead, and Freney Citation1999). Under these conditions, the closed chamber method is a good alternative for field detection because it is easier, smaller, and cheaper (Denmead Citation2008).
The closed chamber method estimates gas flux based on the accumulated concentrations of target gases by inserting the closed chamber into emission surface within a certain period (Ding et al. Citation2015) and is widely used to monitor gaseous emissions between the earth’s surface and the atmosphere (Livingston, Hutchinson, and Spartalian Citation2006), for soils in particular. Closed chamber measurements are documented as being impacted by their own configurations and settings (de Klein and Harvey Citation2013; Ding et al. Citation2015; Pihlatie et al. Citation2013; Pumpanen et al. Citation2004), the microclimate of the covered emitting surface (Davidson et al. Citation2002; Reichman and Rolston Citation2002), measured molecular gas (Davidson et al. Citation2002; Wan et al. Citation2005; Yao et al. Citation2009), and deployment time (Davidson et al. Citation2002; Wan et al. Citation2005), as well as sampling interval and location (de Klein and Harvey Citation2013).
Theoretically, wind speed and deployment time can change the mass emissions process by altering concentration and pressure gradient between both sides of the emitting surface. Previous studies reported that water evaporation rates increased with wind speed increasing over soil columns (Acharya and Prihar Citation1969; Benoit and Kirkham Citation1963). Kimball and Lemon (Citation1971) documented that wind speeds from 0 to 5 m sec−1 positively affected heptane evaporation from soil. However, because most of these studies are carried out for soils, there is little information on using closed chambers on livestock farms, which are regarded as important GHGs sources.
The main GHGs emissions from dairy open lots are CO2, CH4, and NO2. Suitable deployment times for these gas emission measurements from soil sources reported in previous studies varied greatly (de Klein and Harvey Citation2013; Livingston, Hutchinson, and Spartalian Citation2006; Petersen et al. Citation2012; Tanka, Poul, and Lars Citation2016; Wan et al. Citation2005). Wan et al. (Citation2005) recommended that deployment times for CO2, N2O, and CH4 measurements should be within 25 min, 15–30 min, and less than 30 min, respectively. At the same time, other studies have suggested capturing small fluxes of CO2, N2O, and CH4 with a relatively longer deployment time of 30–60 min (de Klein and Harvey Citation2013; Livingston, Hutchinson, and Spartalian Citation2006; Petersen et al. Citation2012; Tanka, Poul, and Lars Citation2016). Obviously, there is no explicit deployment time for detecting these gases with different molecular masses, for livestock operation emission measurements in particular, and it is essential to understand the effect of deployment time and to confirm the special operations for gases of different molecular masses using a certain chamber technique.
To scientifically operate a closed chamber to quantify GHGs emissions from an open operations of dairy farms, a simulation system was set up in the laboratory to (1) evaluate the influences of external surface wind speed (ESWS) and deployment time on measurement accuracy for different GHGs emissions; (2) find appropriate deployment time for chamber operations; and (3) recommend appropriate operational approach of the chamber on open operations of dairy farms.
Materials and methods
Experimental design
Ding et al. (2016a; Citation2016b) found that up to 92.97% of the average surface wind speeds over open lots and manure stockpile in a commercial dairy farm from spring to winter in Beijing were within 0–2 m sec−1. Thus, seven expected ESWS gradients within that range were designed in this study, namely, 0.00 m sec−1, 0.25 m sec−1, 0.50 m sec−1, 0.75 m sec−1, 1.00 m sec−1, 1.50 m sec−1, and 2.00 m sec−1. Five replicate measurements of each gradient were conducted.
To assess the impacts of deployment time on the measurement accuracy of the closed chamber for reference gases, a gradient ranging from 0 to 120 min with a 10-min interval was designed, covering nearly all of the studied deployment time before. Five replicates were tested for each deployment time.
Experimental setup
Tests were carried out at the Key Laboratory of Agricultural Engineering in Structure and Environment, Ministry of Agriculture and Rural Affairs, Beijing, China. A wind tunnel was employed to offer expected airflows at the emitting surface to simulate wind effect in practice (). The closed chamber system was placed 1 m away from the air outlet of the wind tunnel, where a steady wind profile, including wind speed and direction, could be generated in the test area ().
Figure 1. Schematic diagram of the experimental setup in the laboratory. All units are in meters. (a) Top view of wind tunnel and testing area. (b) Closed chamber and gas sampling systems. ×, T and RH are sensors to measure air temperature and relative humidity of the test area; ∆, hot wire anemometer to measure wind speed over the emitting surface of the closed chamber headspace.
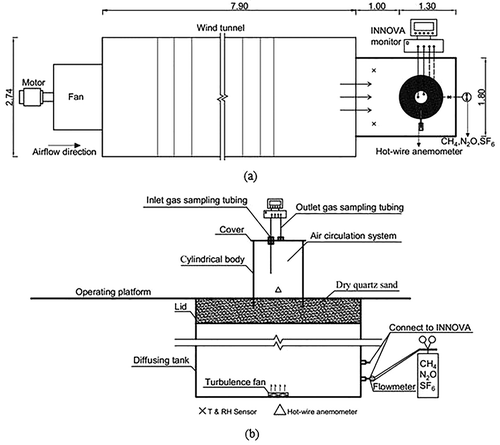
Wind tunnel
The wind tunnel was designed based on the criteria of ANSI/AMCA Standard 210–99 and GB20068418-T326 and its structure was given by Li et al. (Citation2006). Controlled by a frequency adaptor, the rated power, maximum airflow rate, and adjustable rotating rates of the fan in the wind tunnel were 37 kW, 60,000 m3 hr−1, and 30–150 rpm, respectively.
Closed chamber and calibration system
A cylindrical closed chamber with a size of 300 mm (diameter) × 400 mm (height) (D300× H400), made of acrylic, was designed to evaluate the impacts of ESWS and deployment time on measurement accuracy. The closed chamber consisted of a cylindrical body, a cover, and an air circulation system (). The air circulation system was set for gas mixing in the headspace. A gas monitor was connected to the air circulation system by Teflon tubes (3 mm inner diameter) to continuously monitor gas concentrations. Two ports on the cover served as air inlet and outlet (). The air sampling point was located in the middle of the body, and the air outlet port was in the cover. The gap between the cylindrical body and its cover was sealed with a rubber gasket.
A chamber calibration system, designed based on Widén and Lindroth’s study (Citation2003), was constructed to offer an emission source with known reference gas fluxes. The system was made up of a reference gas resource, a cylindrical diffusion tank with a lid, a turbulence fan, and a gas analyzer. A detailed description was given by Ding et al. (Citation2015). The lid was full of quartz sand with its aeration porosity of 35% and bulk density of 2.2 g cm−3 measured using the Archimedean method, to simulate the agricultural fields of gas emissions (Grünzweig et al. Citation2003). In China, unpaved soil is mostly used as the floor base for open dairy lots. The bulk density of unpaved soil is 2.1 g cm−3, and its porosity is about 28% to 32%, which are similar to those of quartz sand. In this study, 0.2% CH4, 0.05% N2O, and 0.5% SF6 sources, with molecular masses of 16.04 g mol−1, 44.01 g mol−1, and 146.05 g mol−1, respectively, were released into the calibration system as target gases. The reference flux in this system was assumed as a constant and steady supply because of the very small concentration decreased over the deployment time (Pihlatie et al. Citation2013).
Measurement of gas concentration, wind speed, temperature and relative humidity
As shown in , wind speed over the emitting surface was recorded at a 10-min interval by a hot-wire anemometer (model KA41 L, Kanomax, Osaka, Japan), which was located 5 cm above the operating platform. The test was not started until the wind speed was under a steady state of the targeted value for at least 15 min. Ambient temperature (T) and relative humidity (RH) in the test area were automatically monitored with three T and RH sensors (Testo175-H1, FLEXIM, Berlin, Germany), which were located in an equilateral triangle pattern on the platform (in ). The sampling interval was 30 min.
In order to reduce the effect of edge turbulence, the closed chamber was inserted into the quartz sand at a depth of 50 mm, 24 hr prior to the first measurement. Before starting a test, a certain amount of targeted gas was injected into the diffusion tank through the valve to reach an initial concentration of approximately 90 mg m−3 and then completely mixed by the turbulence fan set on the bottom of the tank. The emission fluxes of CH4, N2O, and SF6 from quartz sand under ESWS of 0 m sec−1 were 2.93 ± 0.43 mg m−2 hr−1, 2.52 ± 0.28 mg m−2 hr−1, and 2.00 ± 0.19 mg m−2 hr−1, which were similar to the average CH4 and N2O emissions from open dairy lots of 0.85–12.41 mg m−2 hr−1 and 1.48–2.40 mg m−2 hr−1 (Ding et al. Citation2016b). The flow was measured by a digital flowmeter (LZB-3 WB, Changzhou Shuanghuan Metal Products Co., Ltd, Changzhou city, China), keeping each gas flux down to 100 L min−1. After the reference gas in the diffusion tank had been released into the quartz sand for approximately 2 hr to achieve a stabilization state, testing began. Reference gas concentrations of CH4, N2O, and SF6 in the closed chamber and diffusion tank were continuously measured and automatically stored by a photoacoustic multigas monitor (model: INNOVA 1412i, Lumasense Technologies A/S, Santa Clara, CA; range: 0.4–4000 ppm for CH4, 0.03–300 ppm for N2O, 0.006–50 ppm for SF6; accuracy: ±12 ppb for CH4, ±15 ppb for N2O, ±10 ppb for SF6) at every 10-min interval, combined with a multiplexer. The sampling flux of the photoacoustic multigas monitor with a built-in pump was 4 L min−1, and the sampling time was set up to 1.5 min. During sampling, target gases from the headspace were continuously extracted into the middle sampling point by the gas monitor, and were circulated into the closed chamber at the same time through the air outlet port on the cover to improve the gas mixing in the headspace. During the sampling, the surface wind speed inside the chamber headspace was measured at 0.13 ± 0.04 m sec−1. Ding et al. (Citation2015) reported that the measured fluxes by a D300× H400 closed chamber were no statistical difference under the inner surface wind speed of 0.00 m sec−1 and 0.45 m sec−1. Hence, the influence of the air flow inside the headspace caused by the monitor when sampling was ignored.
Gas emission estimation
Estimation method has a great influence on accurately assessing the emission flux. Gao and Yates (Citation1998) reported that when the chamber height was 40 cm, gas concentrations in the chamber showed linear increase within 90 min. In our prestudy, accumulated concentrations versus deployment times fit linear regressions well under different ESWS at the longest deployment time (120 min) for CH4, N2O, and SF6, with R2 values all greater than 0.97. Thus, a linear calculation scheme was used to estimate flux. The gas flux from the emitting surface, measured with the closed chamber method, was calculated by Butterbach-Bahl, Kiese, and Liu (Citation2011):
where F1 is the gas flux measured by the closed chamber (mg m−2 min−1); V is volume in the headspace of the chamber (m3); A is the emitting surface area covered by the chamber (m2); dc is the accumulated gas concentration in the chamber headspace during a certain time (mg m−3); and dt is a certain time of chamber enclosure (min).
The reference gas flux of the calibration system, considered as the “true” emissions rate (reference flux) from the emitting surface, was estimated by Pumpanen et al. (Citation2004):
where F2 is the reference flux of the target gas (mg m−2 min−1), and V2 and Vs are the volumes of the diffusion tank and the quartz sand pore layer (m3), respectively. The volume of sand pore layer is equal to the volume of the lid by the sand porosity of 35%; Cf(t1) and Camb(t1) are the target gas concentrations in the diffusion tank and ambient atmosphere at t1 (mg m−3), respectively; Cf(t2) and Camb(t2) are the target gas concentrations in the diffusion tank and ambient atmosphere at t2 (mg m−3), respectively; t1 and t2 are the times of the test starting and finishing (min), respectively; and A2 is the emitting surface area of the diffusion tank (m2).
Assuming Camb was constant during the whole experiment, this equation can be rearranged as
Then, a deviation ratio (DR) between the measured flux with the closed chamber method and the “truth” emission rate (reference flux) can be described as
Statistical analyses
When calculating the emission flux of the closed chamber, the maximum and minimum values of the five replications were excluded. Results were expressed as “means ± standard deviation” (with a significance level of 0.05), and SPSS (version 21.0) was used to do statistical analyses. Least significant differences, repeated measurement analyses of variance (ANOVAs), and correlation analyses were done to analyze the influences of deployment time and ESWS on the measurement accuracies of the closed chamber method.
Results and discussion
Performance of closed chamber on CH4, N2O, and SF6 measurements
shows the overall average deviation ratios between the measured flux with the closed chamber and the reference flux of the target gases under different deployment times. The results indicate that the deviations between detected and reference CH4, N2O, and SF6 fluxes were from 9.99 to −37.32%, −14.47 to −35.09%, and −4.21 to −27.55%, respectively, during the whole deployment period. The molecular masses of CH4 and N2O are comparatively light, so they could mix rapidly into the closed chamber during a relative short enclosure period to reduce the concentration difference between the emission surface and the chamber. As a macromolecule gas, SF6 might not be completely mixed at different heights of the closed chamber for a relative short enclosure period, which might be part of the reason that the measurement accuracy was the lowest at the deployment period of 20 min. Moreover, a slight SF6 concentration difference was observed in different heights inside the closed chamber in the pre-experiment, which suggested more time needed to mix the gas released into the chamber without turbulence.
Table 1. Deviation ratios (%) between measured and reference fluxes under different deployment times.
Significant differences were found among CH4 deviation ratios for each of the closed periods in the first 1 hr (p < 0.05), with values changing from 9.99% to −20.38%. The deviations at 20 min and 30 min were comparatively close to 0%, suggesting that those might be appropriate deployment times. Weishampel and Kolka (Citation2008), as well as Wan et al. (Citation2005), also recommended a deployment time of 30 min to measure CH4 with the chamber technique. No significant difference was observed between N2O deviations at 10 min and 20 min (p > 0.05), and the ratios were −14.47% and −15.26%, respectively. At the same time, the measurements at these two deployments were significantly different from the others (p < 0.05). This suggests that a comparatively good time to measure N2O with a D300× H400 closed chamber without a vent or fan is 10 to 20 min, which is similar to the recommendations of Philippe and Nikita (Citation2008) and Wan et al. (Citation2005). Generally, SF6 measurement accuracies at 10, 20, and 30 min were significantly lower than at other times (p < 0.05), and there were no significant differences among measurements from 40 to 120 min (p > 0.05), with deviation ratios from −4.21% to −8.57%. Additionally, SF6 deviation ratios were all less than −5% at the 70 to 90 min deployment times, which were recommended in this paper. Our finding is longer than the 55 min recommendation of Ding et al. (Citation2015) for measuring low emission rates by the chamber technique, while the deviation was approximately −15% in Ding et al. (Citation2015), a value much bigger than we found in this study.
Influence of deployment time on measurement accuracy of closed chamber
Deployment time is an important factor on the operation performances of certain chambers. Covered by a closed chamber, the microenvironment of the measured emitting surface and chamber headspace can change over deployment time (Gao and Yates Citation1998; Reichman and Rolston Citation2002), which may include factors such as surface wind speed, temperature, humidity, and the pressure gradient, increasing the uncertainty of the chamber measurements.
shows CH4, N2O, and SF6 measurement accuracies using the closed chamber method at varied deployment times and ESWS. Deviation ratios of the measured and reference fluxes of the three gases showed the same trend under different wind speeds with extended closed time (), which illustrated that ESWS values of less than 2.0 m sec−1 did not dramatically impact gas emissions under the closed chamber. At the same time, measurement was remarkably affected by the vertical emission of gases over time, which agreed with previous findings (Gao and Yates Citation1998; Reichman and Rolston Citation2002). As shown in , CH4 flux measured by the closed chamber method was overestimated in the first 20 min, and then underestimation occurred when the deployment was extended over 30 min. With a closed lid, the atmospheric mixing processes near the emission surface were instantaneously affected (Hutchinson et al. Citation2000), and the feedback would increase the volume-to-area (V/A) ratio of the chamber, causing an overestimation in a short enclosure period (Rachhpal et al. Citation2012). N2O and SF6 fluxes measured by the closed chamber method were all smaller than the reference fluxes during the test, which was consistent with the findings of Rochette and Bertrand (Citation2007). For N2O, measurement accuracy decreased with increased deployment time, and the deviation ratios were lower than 20% in the first 20 min. SF6 deviation decreased in the first 40 min, and then it trended toward stabilization with measurements extending to 120 min. It could be seen that the post-lid-closure feedback had a bigger impact on lighter gases.
Figure 2. Deviations among CH4, N2O, and SF6 fluxes measured with the closed chamber method, and reference fluxes of target gases under different external surface wind speeds (ESWS) as well as different deployment times: (a) CH4, (b) N2O, and (c) SF6.
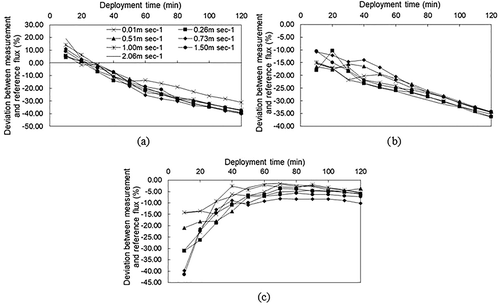
As a key factor on emission measurements from an open source, deployment period is also documented to be dependent on chamber configuration, including the V/A ratio and the vent and fan settings. shows some study findings, suggesting similar deployment times of chambers with different configurations and settings. Installation of a vent and fan in a closed chamber may also affect the inner environment, which may consequently impact the measurement accuracy of the chamber. In the case of Weishampel and Kolka (Citation2008), the V/A ratio of the chamber was smallest, while a vent helped to balance the gas concentration gradient between the inner chamber and atmosphere, hence relatively extending deployment time. Wan et al. (Citation2005) applied a much larger V/A ratio and illustrated that the setting of the fan in the chamber headspace shortened the closed time because of quicker, better blending. Air cycling by a built-in pump of the INNOVA analyzer used in this study may also serve a fan function to some extent.
Table 2. Examples of suitable deployment times for measuring GHG emissions in closed chambers with different configurations and settings.
Effect of external surface wind speed (ESWS) on measurement accuracy of closed chamber
During the study, ESWS of the test setup was close to the designed value and is shown in .
Table 3. Fan frequency and average wind speed over the measurement surface provided by the wind tunnel during the test.
shows the deviation ratios of CH4, N2O, and SF6 under different ESWSs, in which the curves of the three gases reflected the influence of ESWS on measurement with deployment times of 20 to 30 min, 10 to 20 min, and 70 to 90 min, respectively. The results indicated that the deviation ratios were from 2.21 to −3.02% and from −12.74 to −17.17% for CH4 and N2O, respectively; there were no significant differences on the measurements under varied ESWS from 0 to 2 m sec−1 (p ˃ 0.05) during a relatively short deployment time. Redeker, Baird, and Teh (Citation2015) reported that CH4 flux was underestimated using the closed chamber technique, and significant differences on deviation ratio were observed with the ESWS increasing from 0.00 to 2.00 m sec−1. However, forest, grassland, and peat bog were explored as emission surfaces in their study, and were usually more porous than quartz sand. For example, the porosity in the humus layer could exceed 80% (Pihlatie et al. Citation2013). Gas fluxes from these porous surfaces are more easily positively affected by wind turbulence (Widén and Lindroth Citation2003), which hence increases the CH4 flux underestimation beneath the chamber as the ESWS increasing. Reicosky and Denmead (Citation2003) found large differences in CO2 loss between tilled and nontilled soil, and stated higher air permeability of the soil was more sensitive to wind turbulence, which caused higher gas exchange. Thus, porous emission surface might be the reason leading to greater underestimation. Meanwhile, the soil-water content in these natural emission surfaces may also enhance the phenomenon. The soluble gas flux, such as CO2, N2O, and H2S, might be underestimated even more with the increasing of humidity in manure (Lund et al. Citation1999). According to Philippe and Nikita (Citation2008), the confidence level of the CH4 measurement using a D300× H400 closed chamber in our study was high (<10%) and that of N2O was at a medium confidence level (<30%). The influence of ESWS on SF6 measurement differed from that of CH4 and N2O; the underestimation ratios under different wind speeds were 2.39, 4.04, 4.83, 8.28, 2.77, 6.17, and 1.99%, respectively, which were all within a high confidence level (<10%) (Philippe and Nikita Citation2008).
Interaction between deployment time and ESWS
The correlation analysis, given in , shows there was no correlation between ESWS (under 2.06 m sec−1) and measurement accuracies of the closed chamber for CH4, N2O, or SF6 (p > 0.05), while all three gases were significantly influenced by deployment time. Deployment negatively affected CH4 and N2O measurement accuracies (p < 0.05), and it was positively correlated with SF6 measurement (p < 0.05) because more time was needed for mixing after being released into the chamber headspace, illustrating that varied deployment times should be considered when measuring different molecular gases. Data in and also indicate that the influence of deployment time played a more important role for chamber measurements than ESWS, mainly because the chamber cut off the lateral flux of gases beneath the emitting surface, and the measurement accuracy was generally affected by vertical emission. The deviations in SF6 for all tested ESWS had no significant differences (p > 0.05) with a deployment time of 40 min, except for the wind speeds of 0.51 m sec−1 and 1.00 m sec−1. This result differed from that of another deployment time. It indicated that deployment time and wind speed may have a complex interaction on the measurement accuracy of the closed chamber.
Table 4. Correlation analysis of deployment time and ESWS for CH4, N2O, and SF6 measurement accuracies as detected in a closed chamber.
The main effect analysis listed in indicates influences of deployment time, ESWS, and the interaction of the two factors on the measurement accuracies of CH4, N2O, and SF6, where the ranges of deployment time and ESWS were 0 to 120 min and 0.00 to 2.06 m sec−1, respectively. This shows that all three gases were clearly affected by deployment time and ESWS (p < 0.05) and that the interaction of the two factors significantly affected CH4 and SF6 measurement accuracies (p < 0.05), except for N2O (p > 0.05).
Table 5. Main effect analysis of deployment time and ESWS and the interaction of the two factors for CH4, N2O, and SF6 measurement accuracies detected in a closed chamber.
shows the influences of deployment time on different gases and illustrates the importance of finding a suitable time for measuring gas emission fluxes. With a suitable deployment period, the CH4 and N2O measurement accuracies were not affected by ESWS (). When deployment time was extended or shortened, this phenomenon changed. For N2O, with a time from 30 to 60 min, the ESWS had a significant influence, especially with wind speeds of 1.50 m sec−1 and 2.06 m sec−1, which dramatically decreased accuracy (p < 0.05). At ESWS of 1.50 m sec−1 and 2.06 m sec−1, the Reynolds numbers were 4530 and 6040, respectively, suggesting that turbulent flow promoted gas emissions out of the chamber, making accuracy worse.
Operation of closed chamber to measure GHG emissions from dairy production
In China, open lots are typical open dairy operations housing dairy cattle, where the manure is commonly scraped out, and the collected solid manure is temporally stacked outside uncovered from weeks to months. The accumulation and penetration of manure on the lots as well as the manure stockpile are important sources of GHGs and NH3 emissions to the atmosphere, especially for the CH4 and N2O. As reported, the annual CH4 and N2O emissions from open dairy lots with diurnal and seasonal variations are of 0.85–757.27 mg m−2 hr−1 and 0.15–27.2 mg m−2 hr−1 (Ding et al. Citation2016b; Leytem et al. Citation2011), respectively. Because of its open characteristic, direct measurement of the emission is still challenging, especially for the comparatively low flux rate. Pihlatie et al. (Citation2013) proved the possibility of measuring low emission flux levels from 0.20 to 2.30 mg m−2 hr−1 using CH4 as the target gas by the closed chamber technique, and showed the mean chamber fluxes to reference fluxes ratios were not significantly different among the four fluxes (p = 0.99). In this study, the tested flux rates of the three target gases were 2.93 ± 0.43 mg m−2 hr−1, 2.52 ± 0.28 mg m−2 hr−1, and 2.00 ± 0.19 mg m−2 hr−1 for CH4, N2O, and SF6, respectively, close to the high level that Pihlatie et al. (Citation2013) explored.
Based on the results of this study, deployment times of 20 to 30 min and 10 to 20 min were recommended to measure CH4 and N2O emissions using the D300× H400 closed chamber, respectively, from the open operations of dairy farms under wind speeds lower than 2 m sec−1. For the measurement of SF6, a typical tracer gas, a deployment of 70 to 90 min was suggested. This recommendation satisfied the configuration suggested by de Klein and Harvey (Citation2013), which specified that the chamber height to deployment time ratio and insertion depth to deployment time ratio should be not less than 40 cm hr−1 and 12 cm hr−1, respectively. In practice, aluminum foil wrapping for the whole chamber is suggested to avoid the effect of solar radiation when operating an open field measurement. To avoid air leakage of the headspace, the chamber should be cut into the manure or sealed 24 hr prior to the test. When used on dairy farms, the chamber should be guarded or protected away from the animals.
The quartz sand used in this study was a homogeneous resource, which was differed from most natural emission resources of dairy operations, including the open lots ground and the manure stockpile. Unpaved soil is a typical type for open dairy lots, and the bulk density and porosity are similar to quartz sand. However, dairy manure is a complex porous medium containing GHGs sources from both heterotrophic and autotrophic respiration, and is usually more porous and much wetter than quartz sand, as indicated by Ding et al. (Citation2016b), so that the humidity in the fresh manure can exceed 84%. Compared to quartz sand, the turbulence out of the chamber has been proven to have a bigger influence on dairy manure because of its higher porosity, which then increases the underestimation of target gas measurement (Lai et al. Citation2012; Widén and Lindroth Citation2003). Furthermore, the target gas underneath the chamber, storied in the pores of manure, would help to increase the underestimation (Conen and Smith, Citation2000). However, increased water content of dairy manure in the opposite direction is expected to decrease the effects of positive pressure within the chamber (Lund et al. Citation1999) to diminish the underestimation. In reality, the interaction of both porosity and humidity differences of the emission surfaces on gas emission is more complicated, which may alter the measurement accuracy of the closed chamber. Also, the influence for the GHGs emission measurement on open dairy lots and manure stockpile using a closed chamber should be further verified by field tests.
Summary
The effects of deployment time and wind speed over the emitting surface on the measurement accuracies of a D300× H400 closed chamber were evaluated in the laboratory using CH4, N2O, and SF6 as reference gases. CH4 measurement was overestimated in the first 20 min by the closed chamber, and then underestimation occurred as the deployment time extended, while the N2O and SF6 fluxes were all underestimated under all tested runs. Deployment time negatively affected CH4 and N2O measurement accuracies, but positively affected that of SF6. CH4 and SF6 measurement accuracies were also greatly influenced by the interaction of the deployment times and ESWS.
At surface wind speed under 2.00 m sec−1, deployment times of 20 to 30 min and 10 to 20 min are recommended to measure CH4 and N2O emissions from open dairy lots and manure stockpile using the D300× H400 closed chamber, respectively. Measures to protect the closed chamber from solar radiation, air leakage and animal activities should be taken for a better accuracy when operating the field measurements.
Additional information
Funding
Notes on contributors
Yu Liu
Yu Liu is a Ph.D. student in the Department of Agricultural Structure and Bioenvironmental Engineering, China Agricultural University (CAU), China.
Chaoyuan Wang
Chaoyuan Wang is a professor in the Department of Agricultural Structure and Bioenvironmental Engineering, College of Water Resources and Civil Engineering, China Agricultural University, China.
Zixin Wang is an undergraduate student in the Department of Agricultural Structure and Bioenvironmental Engineering of CAU.
Luyu Ding
Luyu Ding is an assistant scientist in the National Engineering Research Center for Information Technology in Agriculture, China.
Zixin Wang
Zixin Wang is an undergraduate student in the Department of Agricultural Structure and Bioenvironmental Engineering of CAU.
Guanghui Teng
Guanghui Teng, Zhengxiang Shi, and Baoming Li are professors in the Department of Agricultural Structure and Bioenvironmental Engineering, College of Water Resources and Civil Engineering, China Agricultural University, China.
Zhengxiang Shi
Guanghui Teng, Zhengxiang Shi, and Baoming Li are professors in the Department of Agricultural Structure and Bioenvironmental Engineering, College of Water Resources and Civil Engineering, China Agricultural University, China.
Baoming Li
Guanghui Teng, Zhengxiang Shi, and Baoming Li are professors in the Department of Agricultural Structure and Bioenvironmental Engineering, College of Water Resources and Civil Engineering, China Agricultural University, China.
References
- Acharya, C. L., and S. S. Prihar. 1969. Vapor losses through soil mulch at different wind velocities. Agron. J. 61:666–668. doi:10.2134/agronj1969.00021962006100050004x.
- Benoit, G. R., and D. Kirkham. 1963. The effect of soil surface conditions on evaporation of soil water. Soil Sci. Soc. Amer. J. 27:495–498. doi:10.2136/sssaj1963.03615995002700050009x.
- Butterbach-Bahl, K., R. Kiese, and C. Liu. 2011. Measurements of biosphere-atmosphere exchange of CH4 in terrestrial ecosystems. Method. Enzymol. 495:271–287. doi:10.1016/B978-0-12-386905-0.00018-8.
- Conen, F., and K. A. Smith. 2000. An explanation of linear increases in gas concentration under closed chambers used to measure gas exchange between soil and the atmosphere. Eur. J. Soil Sci. 51:111–117. doi:10.1046/j.1365-2389.2000.00292.x.
- Davidson, E. A., K. Savage, L. V. Verchot, and R. Navarro. 2002. Minimizing artifacts and biases in chamber-based measurements of soil respiration. Agr. Forest. Meteorol. 113:21–37. doi:10.1016/S0168-1923(02)00100-4.
- de Klein, C., and M. Harvey. 2013. Nitrous oxide chamber methodology guidelines. Wellington, New Zealand: Ministry for Primary Industries.
- Denmead, O. T. 2008. Approaches to measuring fluxes of methane and nitrous oxide between landscapes and the atmosphere. Plant Soil 309:5–24. doi:10.1007/s11104-008-9599-z.
- Ding, L., W. Cao, Z. Shi, B. Li, C. Wang, G. Zhang, and S. Kristensen. 2016a. CO2 and CH4 emissions from the ground level of dairy open lots. J. Air Waste Manage. Assoc. 66 (7):715–725. doi:10.1080/10962247.2016.1173605.
- Ding, L., Q. Lu, C. Wang, Z. Shi, W. Cao, and B. Li. 2015. Effects of configuration and headspace mixing on the accuracy of flux chambers for dairy farm gas emission measurement. Appl. Eng. Agric. 31:153–162. doi:10.13031/aea.31.10853.
- Ding, L., Q. Lu, L. Xie, J. Liu, W. Cao, Z. Shi, B. Li, C. Wang, G. Zhang, and S. Ren. 2016b. Greenhouse gas emissions from dairy open lots and manure stockpile in Northern China: A case study. J. Air Waste Manage. Assoc. 66 (3):267–279. doi:10.1080/10962247.2015.1124058.
- Gao, F., and S. R. Yates. 1998. Simulation of enclosure-based methods for measuring gas emissions from soil to the atmosphere. J. Geophys. Res. 103:26127–26136. doi:10.1029/98JD01345.
- Grünzweig, J. M., T. Lin, E. Rotenberg, A. Schwartz, and D. Yakir. 2003. Carbon sequestration in arid-land forest. Global Change Biol. 9:791–799. doi:10.1046/j.1365-2486.2003.00612.x.
- Harper, L. A., O. T. Denmead, and J. R. Freney. 1999. Direct measurements of methane emissions from grazing and feedlot cattle. Anim. Sci. J. 77:1392–1401. doi:10.2527/1999.7761392x.
- Hutchinson, G. L., G. P. Livingston, R. W. Healy, and R. G. Striegl. 2000. Chamber measurements of surface–Atmosphere trace gas exchange: Numerical evaluation of dependence of soil, interfacial layer, and source/sink properties. J. Geophys. Res-Atmos. 105:8865–8875. doi:10.1029/1999JD901204.
- Kimball, B. A., and E. R. Lemon. 1971. Air turbulence effects upon soil gas exchange. Soil Sci. Soc. Amer. Proc 35:16–21. doi:10.2136/sssaj1971.03615995003500010013x.
- Kutzbach, L., J. Schneider, T. Sachs, M. Giebels, H. Nyk Nen, N. J. Shurpali, P. J. Martikainen, J. Alm, and M. Wilmking. 2007. CO2 flux determination by closed-chamber methods can be seriously biased by inappropriate application of linear regression. Biogeosciences 4:1002–1025. doi:10.5194/bg-4-1005-2007.
- Lai, D. Y. F., N. T. Roulet1, E. R. Humphreys, T. R. Moore, and M. Dalva. 2012. The effect of atmospheric turbulence and chamber deployment period on autochamber CO2 and CH4 flux measurements in an ombrotrophic peatland. Biogeosciences 9:3305–3322. doi:10.5194/bg-9-3305-2012.
- Laubach, J., M. Bai, C. S. Pinares-Patino, and F. A. Phillips. 2013. Accuracy of micrometeorological techniques for detecting a change in methane emissions from a herd of cattle. Agr. Forest. Meteorol. 176:50–63. doi:10.1016/j.agrformet.2013.03.006.
- Leytem, A. B., R. S. Dungan, D. L. Bjorneberg, and C. K. Anita. 2011. Emissions of ammonia, methane, carbon dioxide, and nitrous oxide from dairy cattle housing and manure management systems. J. Environ. Qual. 40:1383–1394. doi:10.2134/jeq2009.0515.
- Li, B., S. E. Ford, Y. Li, and Y. Zhang. 2006. Development of a fan testing chamber for agricultural and horticultural fans in China. Appl. Eng. Agric. 22:115–119. doi:10.13031/2013.18344.
- Livingston, G. P., G. L. Hutchinson, and K. Spartalian. 2006. Trace gas emission in chambers: A non-steady-state diffusion model. Soil. Sci. Soc. Am. J. 70:1459–1469. doi:10.2136/sssaj2005.0322.
- Lovanh, N., J. Warren, and K. Sistani. 2010. Determination of ammonia and greenhouse gas emissions from land application of swine slurry: A comparison of three application methods. Bioresource. Technol. 101:1662–1667. doi:10.1016/j.biortech.2009.09.078.
- Lund, C. P., W. J. Riley, L. L. Pierce, and C. B. Field. 1999. The effects of chamber pressurization on soil-surface CO2 flux, and the implications for NEE measurements under elevated CO2. Global Change Biol. 5:269–281. doi:10.1046/j.1365-2486.1999.00218.x.
- McGinn, S. M., D. Turner, N. Tomkins, E. Charmley, G. Bishophurley, and D. Chen. 2011. Methane emissions from grazing cattle using point-source dispersion. J. Environ. Qual. 40:22–27. doi:10.2134/jeq2010.0239.
- Misselbrook, T. H., J. Webb, D. R. Chadwick, S. Ellis, and B. F. Pain. 2001. Gaseous emissions from outdoor concrete yards used by livestock. Atoms. Environ. 35:5331–5338. doi:10.1016/S1352-2310(01)00289-8.
- Parker, D. B., K. D. Casey, R. W. Todd, H. M. Waldrip, G. W. Marek, B. W. Auvermann, T. H. Marek, K. Webb, W. M. Willis, B. Pemberton, and B. Meyer. 2017. Improved chamber systems for rapid, real-time nitrous oxide emissions from manure and soil. T. Asabe. 60:1235–1258. doi:10.13031/trans.1215a.
- Petersen, S. O., C. C. Hoffmann, C. M. Schafer, G. Blichermathiesen, L. Elsgaard, K. Kristensen, S. E. Larsen, S. B. Torp, and M. H. Greve. 2012. Annual emissions of CH4 and N2O, and ecosystem respiration, from eight organic soils in Western Denmark managed by agriculture. Biogeosciences 9:403–422. doi:10.5194/bg-9-309-2012.
- Philippe, R., and S. E. H. Nikita. 2008. Chamber measurements of soil nitrous oxide flux: Are absolute values reliable? Soil. Sci. Soc. Am. J. 72:331–342. doi:10.2136/sssaj2007.0215.
- Pihlatie, M. K., J. R. Christiansen, H. Aaltonen, J. F. J. Korhonen, A. Nordbo, T. Rasilo, G. Benanti, M. Giebels, M. Helmy, J. Sheehy, S. Jones, R. Juszczak, R. Klefoth, R. Lobo-do-Vale, A. P. Rosa, P. Schreiber, D. Serça, S. Vicca, B. Wolf, and J. Pumpanen. 2013. Comparison of static chambers to measure CH4 emissions from soils. Agr. Forest. Meteorol. s171–172 (8):124–136. doi:10.1016/j.agrformet.2012.11.008.
- Pumpanen, J., P. Kolari, H. Ilvesniemi, K. Minkkinen, T. Vesala, S. Niinistö, A. Lohila, T. Larmola, M. Morero, M. Pihlatie, I. Janssens, J. C. Yuste, J. M. Grünzweig, S. Reth, J. A. Subke, K. Savage, W. Kutsch, G. Østreng, W. Ziegler, P. Anthoni, A. Lindroth, and P. Hari. 2004. Comparison of different chamber techniques for measuring soil CO2 efflux. Agr. Forest. Meteorol. 123:159–176. doi:10.1016/j.agrformet.2003.12.001.
- Rachhpal, S. J., T. A. Black, Z. Nesic, and D. Gaumontguay. 2012. Using automated non-steady-state chamber systems for making continuous long-term measurements of soil CO2 efflux in forest ecosystems. Agr. Forest. Meteorol. 161:57–65. doi:10.1016/j.agrformet.2012.03.009.
- Redeker, K. R., A. J. Baird, and Y. A. Teh. 2015. Quantifying wind and pressure effects on trace gas fluxes across the soil–Atmosphere interface. Biogeosciences 12:7423–7434. doi:10.5194/bg-12-7423-2015.
- Reichman, R., and D. E. Rolston. 2002. Design and performance of a dynamic gas flux chamber. J. Environ. Qual. 31:1774–1781. doi:10.2134/jeq2002.1774.
- Reicosky, D. C., and O. T. Denmead. 2003. Tillage-induced soil properties and chamber effects on gas exchange. Proceedings of the International Soil Tillage Research Organization, Brisbane, Australia, July, 971–976.
- Rochette, P., and N. Bertrand. 2007. Soil-surface gas emissions. In Soil sampling and methods of analysis, ed. M. Carter and E. G. Gregorich, 851–861. Boca Raton, FL: CRC Press.
- Spiehs, M. J., M. H. Whitney, G. C. Shurson, R. E. Nicolai, J. A. R. Flores, and D. B. Parker. 2012. Oder and gas missions and nutrient excretion from pigs fed diets containing dried distillers grains with soluble. Appl. Eng. Agric. 28:431–437. doi:10.13031/2013.41492.
- Tanka, P. K., E. L. Poul, and E. Lars. 2016. Effect of chamber enclosure time on soil respiration flux: A comparison of linear and non-linear flux calculation methods. Atoms. Environ. 141:245–254. doi:10.1016/j.atmosenv.2016.06.062.
- Wan, Y., Y. Li, E. Lin, Q. Gao, and X. Qin. 2005. Studies on closing time in measuring greenhouse gas emission from dry cropland by static chamber method. Chin. J. Agrometeorology 27:122–124. in Chinese.
- Weishampel, P., and R. Kolka. 2008. Measurement of methane fluxes from terrestrial landscapes using static, non-steady state enclosures. In Field measurements for forest carbon monitoring: A landscape scale approach, ed. C. M. Hoover, 163–170. New York, NY: Springer.
- Widén, B., and A. Lindroth. 2003. A calibration system for soil carbon dioxide-efflux measurement chambers: Description and application. Soil. Sci. Soc. Am. J. 67:327–334. doi:10.2136/sssaj2003.3270.
- Yao, Z., X. Zheng, B. Xie, C. Liu, B. Mei, H. Dong, K. Butterbach-Bahl, and J. Zhu. 2009. Comparison of manual and automated chambers for field measurements of N2O, CH4, CO2 fluxes from cultivated land. Atoms. Environ. 43:1888–1896. doi:10.1016/j.atmosenv.2008.12.031.