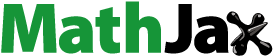
ABSTRACT
Xylene is the main component of many volatile industrial pollution sources, and the use of biotechnology to remove volatile organic compounds (VOCs) has become a growing trend. In this study, a biotrickling filter for gaseous xylene treatment was developed using activated sludge as raw material to study the biodegradation process of xylene. Reaction conditions were optimized, and long-term operation was performed. The optimal pH was 7.0, gas-liquid ratio was 15:1 (v/v), and temperature was 25 °C. High-throughput sequencing technique was carried out to analyze microbial communities in the top, middle, and bottom layers of the reactor. Characteristics of microbial diversity were elucidated, and microbial functions were predicted. The result showed that the removal efficiency (RE) was stable at 86%–91%, the maximum elimination capacity (EC) was 303.61 g·m−3·hr−1, residence time was 33.75 sec, and the initial inlet xylene concentration was 3000 mg·m−3, which was the highest known degradation concentration reported. Kinetic analysis of the xylene degradation indicated that it was a very high-efficiency-activity bioprocess. The rmax was 1059.8 g·m−3·hr−1, and Ks value was 4.78 g·m−3 in stationary phase. In addition, microbial community structures in the bottom and top layers were significantly different: Pseudomonas was the dominant genus in the bottom layer, whereas Sphingobium was dominant in the top layer. The results showed that intermediate metabolites of xylene could affect the distribution of community structure. Pseudomonas sp. can adapt to high concentration xylene–contaminated environments.
Implications: We combined domesticated active sludge and reinforced microbial agent on biotrickling filter. This system performed continuously under a reduced residence time at 33.75 sec and high elimination capacity at 303.61 g·m−3·hr−1 in the biotrickling reactor for about 260 days. In this case, predomestication combined with reinforcing of microorganisms was very important to obtaining high-efficiency results. Analysis of microbial diversity and functional prediction indicated a gradient distribution along with the concentration of xylene. This implied a rational design of microbial reagent and optimizing the inoculation of different sites of reactor could reduce the preparation period of the technology.
Introduction
Xylene is a kind of hydrophobic volatile organic compounds (VOCs), which are widely applied in a variety of chemical products, such as paints, adhesives, inks, dyes, plastics, synthetic fibers, etc. During the manufacturing process, they are released into the environment and may pose a hazard to public health (Ling and Guo Citation2014; Liu et al. Citation2006). In case of the hard-degree pollution, many scholars have studied VOC pollution control technology. Among those technologies, biotechniques showed many advantages, such as energy saving and low cost, compared with physiochemical technology (Burgess, Parsons, and Stuetz Citation2001). They include bioscrubbers, conventional biofilters, biotrickling filters, and novel biofilters for treating waste gases containing hydrophobic and multicomponent VOCs (Gallastegui et al. Citation2011; Granström et al. Citation2002; Qian et al. Citation2018; Yang, Qian, and Li Citation2018). Due to the advantages of low operating cost, convenient operation, and small floor space, biotrickling filter is broadly used for the removal of hydrophobic VOCs. He et al. (Citation2009) showed that biotrickling filters packed with molecular sieve eliminated waste gas containing toluene with a maximum elimination capacity (EC) of about 373.24 g·m−3·hr−1 (removal efficiency [RE] = 100%) at the inlet concentration of 9.19 mg·L−1. Salamanca, Dobslaw, and Engesser (Citation2017) found that the biotrickling filter packed with polyurethane foam had degradation efficiency values of 98.5%–99.2% when the cyclohexane concentration was 120 mg·m−3 and the residence time was 25 sec. In addition, pretreatments such as ultraviolet (UV) oxidation, dielectric barrier discharge, and photocatalytic oxidation have been combined with biotrickling filter to treat hydrophobic and recalcitrant VOCs (Jiang et al. Citation2015; Yu et al. Citation2014).
Effective elimination of VOCs in biotrickling filters was based on the presence of active microorganisms and biofilms, which were mainly composed of bacteria or fungi. Cheng et al. (Citation2016a) found that bacterial-fungal biofilters had better removal and performance stability than fungal biofilter or bacterial biofilters, but fungal biofilters removed less toluene than bacterial biofilters. Moreover, fungi initially grow more slowly than bacteria, which may prolong the start-up period, and their filamentary structure may accelerate the rate of reactor clogging (Cheng et al. Citation2019; Vergara-Fernández et al. Citation2012). Cheng et al. (Citation2016b) also reported that some fungal strains had potential pathogenicity. Therefore, bacteria have always been selected for elimination of VOCs in biological treatment processes. Moreover, biomass in biotrickling filters was critical to the stable operation of the system. Lu et al. (Citation2016) found that the biomass from bottom to top along the biotrickling filter showed a significant decrease layer by layer. Uneven distribution and excess accumulation of biomass in biofilters often led to decrease in removal efficiency for VOCs (Yang et al. Citation2003). Due to the important role biomass plays in the control of VOCs, many biomass accumulation models have been reported (Nukunya, Devinny, and Tsotsis Citation2005; Song and Kinney Citation2002; Yang et al. Citation2003), which established a theoretical foundation for the improved design and operation of biofilters. To maintain system performance, it was necessary to take some measures to control biomass, such as physical methods, chemical methods, biological methods, etc. (Yang et al. Citation2010; Zhu et al. Citation2004).
Since the VOC degradation process was an open system, pure microbial cultures were prone to variation, resulting in a completely different effect from that in the laboratory (Xu et al. Citation2017). To obtain a stable and robust system, application of microbial consortia on waste gas treatment became more attractive. At present, many efforts have been made on removing xylene through bioreactors inoculated with mixed microbial cultures, including factors affecting system performance, resistance to shock loadings, different filling materials, substrate interactions, biodegradation kinetics, etc. (Elmrini et al. Citation2004; Feisther et al. Citation2015; Gallastegui et al. Citation2011; Jorio et al. Citation2000; Liu et al. Citation2006). These research efforts provided essential information on biological mechanism and help us make more progress. With the development of high-throughput sequencing and bioinformatics, more data could be mined and information acquired. Thus, more studies on community structure are required to obtain deep insight into the microorganisms’ mechanism in biotrickling filters. Wu etal. (Citation2018) reported that the removal efficiency reached up to 90.86% under an inlet concentration of 2.259 g·m−3 and 61.5 sec of residence time using biotrickling filters filled with conductive packing material for the treatment of toluene, and the predominant bacterial genera in the reactor were Acidovorax, Rhodococcus, Hydrogeophaga, Brevundimonas, Arthrobacter, Pseudoxanthomonas, Devosia, Gemmobacter, Rhizobium, Dokdonella, and Pseudomonas. However, little research has been reported on microbial community in biotrickling filters for treating xylene. Singh et al. (Citation2017) reported that the removal efficiency was 70%–75% with the inlet xylene concentration of 1.6–2.2 g·m−3 and the residence time of 11.76 sec; however, only the dominating species in the biofilm were indicated, no detailed analyses of the microbial community were reported.
As a matter of fact, many industrial waste gases containing xylene are mixtures, which include o-xylene, m-xylene, and p-xylene. In order to more closerly model the actual situation, xylene mixture (the volume ratio of o-xylene, m-xylene, and p-xylene was 3:10:4) was used as the basis of simulation experiment and calculation to guide future industrial applications. In this study, a biotrickling filter packed with the polyurethane for gaseous xylene treatment was developed using activated sludge as raw material to study the biodegradation process of xylene. The system was tested under shorter residence times and with various inlet concentrations. Mineralization efficiency and reaction kinetics of the system were elucidated to provide a theoretical basis for efficient treatment of xylene. For insight into biological mechanisms, the dynamic changes of microbial communities in different layers of the reactor were analyzed and their functions were predicted. The outcome could provide a theoretical basis for strain combination and help in better design for putative applications.
Materials and methods
Biotrickling filter system
The process map of the xylene biodegrading system is described in . The biotrickling filter reactor had acrylic resin with an inner diameter of 130 mm and a height of 540 mm. Square polyurethane foam with a volume of 1 cm3 was used for supporting cell growth. Packing polyurethane was autoclaved at 121 °C for 20 min before use. Total packing volume was 4.5 L, and a space of 10 cm in height was reserved at the bottom and top of the column. A porous plate was placed between the packing for distribution of liquids and gases. Sampling ports were at the top and bottom of the column. The air was supplied by an air pump. The air stream was divided into two parts. One part of the air stream was connected to the mixing chamber, and the other part entered the bottle containing the xylene solution. The two streams were mixed in a mixing chamber. Two rotameters (3LPM and 8LPM; Changzhou Shuanghuan Thermal Instrument Co. Ltd., Changzhou, China and Yuyao Kingtai Instrument Co. Ltd., Zhejiang, China) were used to control the air stream. The xylene concentration was adjusted from 300 to 3000 mg·m−3, and residence time was from 60 to 33.75 sec. Flow direction of the gaseous stream was from the bottom to the top of the reactor, and it was coupled with a reverse-flow liquid stream. Xylene was degraded by contact with the wetting biofilm. Clean gas was exhausted from the top of the column. The nutrient solution was evenly sprayed on the packing with a metering peristaltic pump from the top of the column, and it then flowed back to the bottle from the bottom of the column. The nutrient solution was circulated at a fixed rate of 300 mL/min in a closed loop during operation. In order to ensure sufficient nitrogen and phosphorus sources, the composition of the nutrient solution for microorganism growth in 2 L distilled water was 11.5 g (NH4)2SO4 and 2.2 g KH2PO4. The nutrient solution was supplemented every 3 days with the same formulation as above. The pH was measured by an online monitor and adjusted to the desired value with 1 M NaOH solution. The liquid medium in the bottle was evenly mixed and heated with a magnetic stirrer. The gaseous sample was collected from sample ports (sampling 10 mL using a syringe) every 24 hr for further analysis. At least three replicate samples were collected for each sample port.
Figure 1. Schematic of the laboratory-scale biotrickling filter. (1) Air pump. (2) Air-mixing chamber. (3) Air rotameter. (4) Impinger for xylene. (5) Air inlet port. (6) Biotrickling filter. (7) Polyurethane packing. (8) Air outlet port. (9) Nutrient medium peristaltic pump. (10) NaOH solution. (11) Lye peristaltic pump. (12) pH online monitor. (13) Nutrient medium. (14) Magnetic stirrer. (15) Support table.
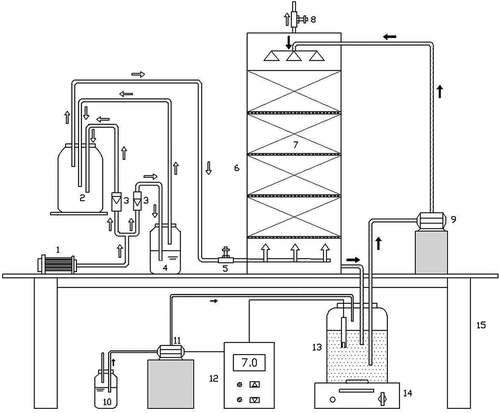
The optimal characteristics of the biotrickling filter, including gas-liquid ratio, pH, and temperature, were determined by the removal efficiency and removal capacity for xylene. The inlet xylene concentration was 2000 mg·m−3, and the residence time was 60 sec. The gas-liquid ratios were 22:1 (v/v), 15:1 (v/v), 10:1 (v/v), and 7:1 (v/v); the pH values were 5.0, 6.0, 7.0, and 8.0; and the temperatures were 25, 30, 35, and 40 °C.
Microorganisms
Aerobic activated sludge was collected at the Tianjin wastewater treatment plant (WWTP), and it was used as the inoculum. Laboratory microflora, a microflora enriched from soil using xylene as substrate, was also added for enhancing the biodegradation of xylene. Activated sludge and laboratory microflora were inoculated into the reactor at a ratio of 2:1 (v/v).
Sample analysis
The concentration of gaseous xylene was determined by gas chromatography (FULI 9790; China) equipped with a flame ionization detector (FID) and packed column (10% SE-30; 0.6 m × 3 mm [inner diameter]). The flow rate of nitrogen was 50 mL·min−1, and the column pressure was 0.08 MPa. The temperatures of the injector, oven, and detector were maintained at 160, 90, and 140 °C, respectively. The performance of the biotrickling filter was evaluated by removal efficiency (RE, %) and elimination capacity (EC, g·m−3·hr−1) for different inlet xylene concentrations (g·m−3), estimated by the following equations:
where Q is the gas flow rate (L·min−1), V is the total volume of the polyurethane medium in the biotrickling filter (L), and Ci and Co are the inlet and outlet xylene concentrations (g·m−3), respectively.
To evaluate the extent of degradation of xylene, the CO2 production rate (PCO2) was calculated using eq 3. CO2 concentrations were measured using an automatic CO2 analyzer (IAQM-7515; TSI) at the inlet and outlet ports of the reactor (Padhi and Gokhale Citation2016). The average of two repetitions (n = 2) was used as the CO2 final concentration.
where PCO2 is the CO2 production rate (g·m−3·hr−1), and CO2,out and CO2,in are the CO2 concentrations at the inlet and outlet ports in the biotrickling filter (g·m−3), respectively.
Kinetics of xylene biodegradation
The kinetic behavior of xylene biodegradation conformed to the Michaelis-Menten model. At present, this model has been widely used to elucidate the biodegradation process and to evaluate rmax (Mathur, Sundaramurthy, and Balomajumder Citation2006; Padhi and Gokhale Citation2016; Rahul et al. Citation2012). Assuming that there was no oxygen limitation in the system, the conversion was in a reaction-controlled state (i.e., the biofilm was fully active) and the growth rate of the microorganisms was at equilibrium with the decay rate (i.e., at steady state); the kinetic equation is
where Ci and Co are the inlet and outlet xylene concentrations, Cln is the log mean xylene concentration [(Cln = (Ci − Co)/ln(Ci/Co)], Q is the volumetric flow rate of gas (m3·hr−1), and V is the biotrickling filter volume (m3). Parameter rmax is the maximum biodegradation rate per unit volume (g·m−3·hr−1); Ks is the saturation constant (g·m−3) in the gas phase and it represents the affinity of microorganisms for xylene. rmax and Ks, were determined by plotting versus
using eq 4.
High-throughput sequencing and bioinformatic analysis
When the xylene removal efficiency in the deep acclimation phase was stable, the upper (10 cm from the surface), middle, and lower (10 cm from the bottom) packings in the biotrickling filter were collected. Cells attached to the packings were washed with sterilized 0.85% NaCl solution and centrifuged at 10,000 × g for 1 min. The precipitate was retained for total DNA extraction using the DNeasy PowerSoil Kit (Qiagen, Hilden, Germany) following the procedure in the product manual. Three replicate samples for each layer were extracted and numbered t1, t2, t3, m1, m2, m3, b1, b2, and b3, respectively. Spectrophotometry (Thermo Scientific NanoDrop 2000C, Thermo Fisher Scientific Inc, Massachusetts, USA) and agarose gel electrophoresis were used to determine the quality of the DNA. High-throughput sequencing was conducted using the Illumina MiSeq 2500 platform at Novogene (Beijing, China). The V4 region of the 16S rRNA gene was amplified in each DNA sample. The sequencing results were analyzed using the Quantitative Insights Into Microbial Ecology (QIIME) software (Caporaso et al. Citation2010).
The Greengenes database was used to determine bacterial species diversity in the samples. The community composition of each sample was counted at the kingdom, phylum, class, order, family, and genus levels. The relative abundance of different bacterial genera in the samples was analyzed. Combined with principal coordinate analysis (PCoA; performed using R, Package Vegan) and SPSS one-way analysis of variance (ANOVA) analysis (version 19; IBM, Armonk, NY, USA), the similarities and differences between the top, middle, and bottom microbial communities were determined. Furthermore, microbial community function was predicted using PICRUSt (Langille et al. Citation2013).
Results and discussion
Performance of the system
To make sure that biofilters were at pseudo-steady state, Song et al. (Citation2012) operated the biofilters under the reference condition for few days before and after changing the operation parameters. Similarly, this study was based on the system removal efficiency to ensure that the biotrickling filter was operated at a steady or pseudo-steady state. Since the continuous high inlet concentration was greatly affected by the height of the xylene liquid level in the bottle, in the experiment, if the inlet xylene concentration was fixed at 2000 or 3000 mg·m−3, it was considered that the fluctuation within 500 mg·m−3 was a reasonable error range. The xylene biodegradation reactor operated at various conditions over 260 days. As shown in biofilm formation and start-up of the biotrickling filter ran for 14 days using the immersion method (phase I). In this phase, the inlet xylene concentration was 1000–2000 mg/m3. After the start-up of the system, the water in the reactor was withdrawn and entered the acclimation phase. The inlet xylene concentration was initially set to 300 mg·m−3 and gradually increased to 3000 mg·m−3, with a constant residence time of 60 sec in the primary acclimatization phase (phase II). The biotrickling filter was determined to be at pseudo-steady state when the removal efficiency of xylene was stable at 90% (phase II, after 52 days of operation). Then the effects of the gas-liquid ratio, pH, and temperature on xylene degradation were investigated with an inlet xylene concentration of 2000 mg·m−3 and a residence time of 60 sec (phase III). In this experiment, the control variable method was adopted, and the system performance was reflected by the removal efficiency and removal capacity for xylene. The optimal value of the gas-liquid ratio was 15:1, the optimal pH was 7.0, and the optimal temperature was 25 °C. In this phase, the effects of some inappropriate conditions can cause system performance degradation. In order to confirm that the system was at pseudo-steady state, this reactor was operated in the recovery and adjustment phase (phase IV) with an inlet xylene concentration of 1000–2000 mg·m−3 and a residence time of 33.75–60 sec. When the removal efficiency of xylene was stable at 90%, changing the operating parameters began to enter the next phase. To further reduce the residence time, based on the phase III optimal conditions, a deep acclimation phase (phase V) was operated with an inlet xylene concentration of 300–3000 mg·m−3 and a residence time of 33.75 sec. The inlet xylene concentration fluctuated between 300 and 3000 mg·m−3 at the beginning of this phase, which was mainly to improve the shock resistance capacity of the system. In the last 20 days of phase V, the inlet xylene concentration of 3000 mg·m−3 and the residence time of 33.75 sec remained unchanged. Finally, xylene removal efficiency was stable at 86%–91% and the maximum elimination capacity reached 303.61 g·m−3·hr−1.
All the time, long residence time and low loading seriously hindered the application of biological methods to purify organic waste gas. Cao et al. (Citation2015) reported that inlet xylene concentration had to be restricted to less than 1000 mg·m−3 for an acceptable removal efficiency at 33.9 sec of residence time. Malakar et al. (Citation2017) reported that the residence time of exhaust gas in the system needed to be 96 sec to have good performance, when inlet toluene concentration was 1900–3000 mg·m−3. In this study, a high-activity microflora was obtained under the conditions of short residence time and high organic loading.
Carbon dioxide (CO2) production analysis
CO2 production is an important parameter to evaluate the performance of the biotrickling filter. It can characterize the mineralization rate of organic compounds, since xylene is eventually converted to water (H2O), CO2, and biomass. The more CO2 produced in the biodegradation process, the less the amount of biomass generated in the reactor, thus slowing the blocking rate of the system. The relationship between CO2 production and EC is described as PCO2 = 3.32EC, where the constant 3.32 is the theoretical value representing all xylene converted to CO2 and H2O. The mineralization rate of this system was calculated in phase V. The linear relationship between CO2 production and the EC of xylene was PCO2 = 2.68EC (). This is consistent with values reported in the literature: the ratio of CO2 production to BTEX (benzene, toluene, ethylbenzene, and xylene) elimination capacity is 2.50–2.93 (Rene, Murthy, and Swaminathan Citation2010; Singh et al. Citation2017), indicating that the bacteria caused relatively complete xylene degradation, with a high mineralization rate of 81%. However, The CO2 production in this study differed from the theoretical value, which may have been due to partially consumed organic carbon used for microbial growth, remaining intermediates, or some of the produced CO2 dissolved in the liquid phase and existed in ion forms (HCO3−, H2CO3, or CO32−).
Kinetics of xylene biodegradation
As shown in the kinetic constants of xylene were determined by plotting versus
. The values of rmax for phase II and phase V were calculated to be 545.7 and 1059.8 g·m−3·hr−1 and Ks values were 6.12 and 4.78 g·m−3, respectively. The correlation coefficients (R2) of the fitted results for phase II and phase V were 0.9922 and 0.9989, respectively. After deep acclimation in phase V, the maximum elimination capacity per unit biotrickling filter volume per unit time was enhanced, indicating the microflora was efficient with a shorter residence time and high loading conditions. Singh et al. (Citation2017) treated xylene with a biofilter under transient and high loading conditions, and their values of rmax and Ks for xylene were 1001.4 g·m−3·hr−1 and 1.83 g·m−3, respectively.
Community structure analysis
The spatial diversity of the microbial community was investigated in the bottom (b1, b2, b3), middle (m1, m2, m3), and top (t1, t2, t3) packing layers of the reactor. The Chao1 index values for estimating the operational taxonomic units (OTUs) from the bottom, middle, and top samples were 1331.70, 1203.40, and 413.53, respectively. The Shannon index values of the bottom, middle, and top layers were 5.70, 5.41, and 4.47. The results showed that species richness and diversity decreased with the increase in reactor height. The weighted UniFrac PCoA results showed that three groups were distinctly separated across the first principal coordinates (PCoA 1 axis), with an interpretation of 81.74% in this direction (). For the second principle coordinates, the interpretation was only 10.99%, with some overlap of microbial community composition in this direction. In the PCoA 1 axis, samples from the middle and bottom layers were located more closely than samples from the top and bottom layers, which indicated that the microbial communities in the middle samples and the bottom samples were more similar than those in the top samples and the bottom samples. These findings suggested that there was a gradual vertical stratification of the microbial communities in the biotrickling filter, which may be due to the differences in the biodegradation extent of the different compounds fed to the system (Lebrero et al. Citation2012).
Figure 5. Principal coordinate analysis (PCoA) of weighted UniFrac distances of the bacterial communities in different samples. T: top samples; M: middle samples; B: bottom samples.
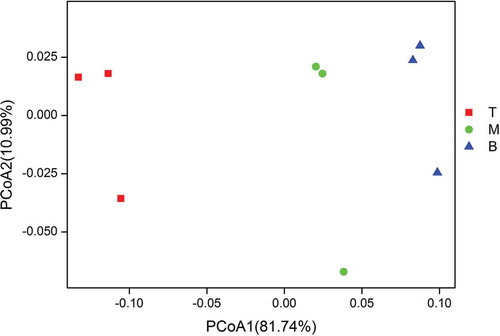
To further study the structure of microbial community in the three layers of the reactor, the community composition of each sample was counted at the genus level (). The abundance levels of genera Pseudomonas and Sphingobium were 15.94%, 20.78%, and 24.64% and 29.36%, 12.07%, and 6.84% for the top, middle, and bottom samples, respectively. Obviously, the abundance of genus Pseudomonas was higher than that of genus Sphingobium in the bottom of reactor, whereas the distribution at the top was reversed. Some species of genus Sphingobium have been reported to have polycyclic aromatic hydrocarbon and monocyclic aromatic hydrocarbon pollutant degradation ability (Chadhain et al. Citation2007; Fu et al. Citation2014). However, a large number of studies had described that only or predominantly strains of genus Pseudomonas degraded xylene in microbial community (Jeong, Hirai, and Shoda Citation2009; Singh and Fulekar Citation2015; Sun et al. Citation2018; Zhao, Huang, and Wei Citation2014). Since the xylene concentration gradually decreased from bottom to top along the reactor, it may be an important parameter affecting the community structure, which was consistent with previous published results (Estrada et al. Citation2012). These findings indicated that the genus Pseudomonas was more tolerant to high xylene concentration than the genus Sphingobium. High xylene concentration in the bottom packing layer would help the enrichment of specialized microorganisms (Wu et al. Citation2017), indicating that xylene was mainly removed by the genus Pseudomonas. In other words, the genus Pseudomonas was more efficient than the genus Sphingobium in degrading xylene. In addition, it has been reported that some strains of Pseudomonas express key enzymes of the biodegradation pathway for xylene, including monooxygenase and catechol-2,3-dioxygenase, and can utilize important intermediate metabolites (Benjamin, Voss, and Kunz Citation1991; Davey and Gibson Citation1974; Suzuki et al. Citation1991). Since the nutrient solution continuously circulated from top to bottom in the packing layers, xylene intermediate metabolites dissolved in the nutrient solution became another important factor affecting the distribution of the community structure. For the purpose of a clearer understanding to the changes of other genera among different samples, significant difference was obtained by SPSS one-way ANOVA (). The proportion of genera Burkholderia, Rhodococcus, Pandoraea, and Stenotrophomonas decreased from the bottom to the top of the reactor. The distribution of genera Novosphingobium and Comamonas from the bottom to the top layer of reactor was opposite to that mentioned above. The abundance of genus Bacillus was the highest in the middle packing layer. Little difference was observed in the distribution of genus Dokdonella within the reactor. These genera were related to the degradation of xylene or its metabolites. Above was the vertical distribution of bacterial community at the end of phase V, which can provide indications for possible further improvement of biotrickling filter design and performance.
Figure 6. Abundances of different bacterial genera in the samples. The abundance is presented in terms of percentage among the total effective bacterial sequences in each sample. The top 10 abound taxa are shown.
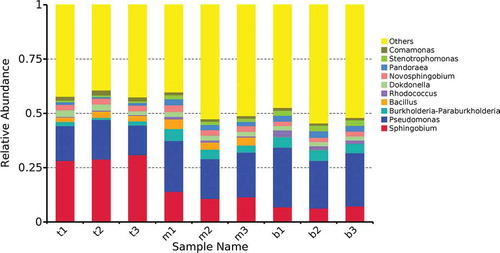
Table 1. Statistically significant differences in genera of the top 10 relative abundance levels in different samples.
The PICRUSt analysis results obtained approximately 300 predicted functions, including transcription factors, ABC transporters, two-component systems, secretion systems, DNA repair and recombination proteins, etc. In addition to some general functional predictions, the overall data also demonstrated the great potential of the studied microbial community in the degradation of aromatics. Naphthalene degradation, toluene degradation, polycyclic aromatic hydrocarbon degradation, styrene degradation, ethylbenzene degradation, and xylene degradation were all predicted (). The comprehensiveness of community functions indirectly explained the reasons for the good performance of the system. Cluster analysis indicated similar functional activity in the middle and bottom packing layers, which was consistent with the result of the middle and lower community structure similarity. Obviously, some cell basic functions, such as bacterial motility proteins, other ion-coupled transporters, and ribosome biogenesis, were different in each layer. Furthermore, the functions of chlorobenzene, nitrotoluene, and xylene degradation were more significant in the bottom packing layer. The reason for this result was directly associated with the dominant species in the bottom packing layer. Some strains of genera Pseudomonas, Rhodococcus, and Pandoraea have been reported to have xylene, nitrotoluene, and chlorobenzene degradation ability, respectively (Baptista et al. Citation2010; Jeong, Hirai, and Shoda Citation2009; Kundu, Hazra, and Chaudhari Citation2016).
Conclusion
Our study revealed effective removal of xylene using a mixed microbial culture dominating by Pseudomonas sp. and Sphingobium sp. supported on polyurethane in a laboratory-scale biotrickling filter under a reduced residence time and a high organic loading. At approximately 25 °C, a gas-liquid ratio of 15:1, a pH of 7, and a gas residence time of 33.75 sec, the RE was stable at 86%–91% for an inlet xylene concentration of 3000 mg·m−3. The maximum EC was 303.61 g·m−3·hr−1. Mass production of CO2 and kinetic analysis showed the high efficiency of the microflora and the possibility that the system could achieve higher EC. From the results of microbial community analysis, a spatial distribution coupling with xylene concentration of the genera was observed, indicating meaningful metabolic strategy adopted by the bacteria in the microbial community of different layers.
Additional information
Funding
Notes on contributors
Mingxue Li
Mingxue Li is a postgraduate student at Tianjin University of Science & Technology, Tianjin, People’s Republic of China.
Yantao Shi
Yantao Shi and Yixuan Li are system engineers of air pollution control at SwanShine (Tianjin) Biotechnology & Development Ltd., Tianjin, People’s Republic of China.
Yizhe Sun
Yizhe Sun is a postgraduate student at Tianjin Institute of Industrial Biotechnology, Tianjin, People’s Republic of China.
Chunhui Song
Chunhui Song is a professor at Key Laboratory of Western China’s Mineral Resources of Gansu Province, School of Earth Sciences, University of Lanzhou, Lanzhou, Gansu, People’s Republic of China.
Zhiyong Huang
Zhiyong Huang is a research professor at Tianjin Key Laboratory for Industrial Biological Systems and Bioprocessing Engineering, Tianjin Institute of Industrial Biotechnology, Tianjin, People’s Republic of China.
Zongzheng Yang
Zongzheng Yang is a research professor at Tianjin University of Science & Technology, Tianjin, China.
Zongzheng Yang was a professor of college of chemical engineering and materials science, Tianjin University of Science & Technology.
Yifan Han
Yifan Han is an assistant researcher at Tianjin Key Laboratory for Industrial Biological Systems and Bioprocessing Engineering, Tianjin Institute of Industrial Biotechnology, Tianjin, People’s Republic of China.
References
- Baptista, I. I. R., N. Y. Zhou, E. A. C. Emanuelsson, L. G. Peeva, A. Mantalaris, and A. G. Livingston. 2010. Evidence of species succession during chlorobenzene biodegradation. Biotechnol. Bioeng. 99 (1):68–74. doi:10.1002/bit.21576.
- Benjamin, R. C., J. A. Voss, and D. A. Kunz. 1991. Nucleotide sequence of xylE from the TOL pDK1 plasmid and structural comparison with isofunctional catechol-2,3-dioxygenase genes from TOL, pWW0 and NAH7. J. Bacteriol. 173 (8):2724–28. doi:10.1128/jb.173.8.2724-2728.1991.
- Burgess, J. E., S. A. Parsons, and R. M. Stuetz. 2001. Developments in odour control and waste gas treatment biotechnology: A review. Biotechnol. Adv. 19 (1):35–63. doi:10.1016/S0734-9750(00)00058-6.
- Cao, C., L. Wang, Q. Wang, and Y. Wang. 2015. High efficient removal of xylene by biotrickling filter. Petrochem. Technol. 44 (9):1121–26.
- Caporaso, J. G., J. Kuczynski, J. Stombaugh, K. Bittinger, F. D. Bushman, E. K. Costello, N. Fierer, A. G. Pena, J. K. Goodrich, J. I. Gordon, G. A. Huttley, S. T. Kelley, D. Knights, J. E. Koenig, R. E. Ley, C. A. Lozupone, D. McDonald, B. D. Muegge, M. Pirrung, J. Reeder, J. R. Sevinsky, P. J. Turnbaugh, W. A. Walters, J. Widmann, T. Yatsunenko, J. Zaneveld, and R. Knight. 2010. QIIME allows analysis of high-throughput community sequencing data. Nat Methods. 7 (5):335–336. doi:10.1038/nmeth.f.303.
- Chadhain, S. M. N., E. M. Moritz, E. Kim, and G. J. Zylstra. 2007. Identification, cloning, and characterization of a multicomponent biphenyl dioxygenase from Sphingobium yanoikuyae B1. J. Ind. Microbiol. Biot. 34 (9):605–13. doi:10.1007/s10295-007-0235-3.
- Cheng, Y., H. J. He, C. P. Yang, G. M. Zeng, X. Li, H. Chen, and G. L. Yu. 2016b. Challenges and solutions for biofiltration of hydrophobic volatile organic compounds. Biotechnol. Adv. 34(6):1091–102. doi:10.1016/j.biotechadv.2016.06.007.
- Cheng, Z. W., L. C. Lu, C. Kennes, J. M. Yu, and J. M. Chen. 2016a. Treatment of gaseous toluene in three biofilters inoculated with fungi/bacteria: Microbial analysis, performance and starvation response. J. Hazard. Mater. 303:83–93. doi:10.1016/j.jhazmat.2015.10.017.
- Cheng, Z. W., X. M. Zhang, C. Kennes, J. M. Chen, D. Z. Chen, J. X. Ye, S. H. Zhang, and D. D. Dionysiou. 2019. Differences of cell surface characteristics between the bacterium pseudomonas veronii and fungus ophiostoma stenoceras and their different adsorption properties to hydrophobic organic compounds. Sci. Total Environ. 650:2095–106. doi:10.1016/j.scitotenv.2018.09.337.
- Davey, J. F., and D. T. Gibson. 1974. Bacterial metabolism of para- and meta-xylene: Oxidation of a methyl substituent. J. Bacteriol. 119 (3):923–29.
- Elmrini, H., N. Bredin, Z. Shareefdeen, and M. Heitz. 2004. Biofiltration of xylene emissions: Bioreactor response to variations in the pollutant inlet concentration and gas flow rate. Chem. Eng. J. 100 (1–3):149–58. doi:10.1016/j.cej.2004.01.030.
- Estrada, J. M., E. Rodríguez, G. Quijano, and R. Muñoz. 2012. Influence of gaseous VOC concentration on the diversity and biodegradation performance of microbial communities. Bioprocess Biosyst. Eng. 35 (9):1477–88. doi:10.1007/s00449-012-0737-x.
- Feisther, V. A., A. A. Ulson de Souza, D. E. G. Trigueros, J. M. M. de Mello, D. de Oliveira, and S. M. A. Guelli Ulson de Souza. 2015. Biodegradation kinetics of benzene, toluene and xylene compounds: Microbial growth and evaluation of models. Bioproc. Biosyst. Eng. 38 (7):1233–41. doi:10.1007/s00449-015-1364-0.
- Fu, B., Q. X. Li, T. Xu, Z. L. Cui, Y. Sun, and J. Li. 2014. Sphingobium sp. FB3 degrades a mixture of polycyclic aromatic hydrocarbons. Int. Biodeter. Biodegr. 87 (2):44–51. doi:10.1016/j.ibiod.2013.10.024.
- Gallastegui, G., A. Ávalos Ramirez, A. Elías, J. P. Jones, and M. Heitz. 2011. Performance and macrokinetic analysis of biofiltration of toluene and p-xylene mixtures in a conventional biofilter packed with inert material. Bioresource Technol. 102 (17):7657–65. doi:10.1016/j.biortech.2011.05.054.
- Granström, T., P. Lindberg, J. Nummela, J. Jokela, and M. Leisola. 2002. Biodegradation of vocs from printing press air by an on-site pilot plant bioscrubber and laboratory scale continuous yeast cultures. Biodegradation 13 (2):155–62. doi:10.1023/a:1020425901752.
- He, Z., L. C. Zhou, G. Y. Li, X. Y. Zeng, T. C. An, G. Y. Sheng, J. M. Fu, and Z. P. Bai. 2009. Comparative study of the eliminating of waste gas containing toluene in twin biotrickling filters packed with molecular sieve and polyurethane foam. J. Hazard. Mater. 167 (1):275–81. doi:10.1016/j.jhazmat.2008.12.116.
- Jeong, E., M. Hirai, and M. Shoda. 2009. Removal of xylene by a mixed culture of Pseudomonas sp. NBM21 and Rhodococcus sp. BTO62 in biofilter. J. Biosci. Bioeng. 108 (2):136–41. doi:10.1016/j.jbiosc.2009.03.024.
- Jiang, L. Y., S. L. Cao, R. Y. Zhu, J. M. Chen, and F. Su. 2015. Analysis of characteristics and products of chlorobenzene degradation with dielectric barrier discharge. Environ. Sci. 36 (3):831–38. doi:10.13227/j.hjkx.2015.03.011.
- Jorio, H., L. Bibeau, G. Viel, and M. Heitz. 2000. Effects of gas flow rate and inlet concentration on xylene vapors biofiltration performance. Chem. Eng. J. 76 (3):209–21. doi:10.1016/S1385-8947(99)00160-6.
- Kundu, D., C. Hazra, and A. Chaudhari. 2016. Bioremediation potential of rhodococcus pyridinivorans nt2 in nitrotoluene-contaminated soils: The effectiveness of natural attenuation, biostimulation and bioaugmentation approaches. J. Soil. Contam. 25 (6):15. doi:10.1080/15320383.2016.1190313.
- Langille, M.G.I., J. Zaneveld, J.G. Caporaso, D. McDonald, D. Knights, J.A. Reyes, J.C. Clemente, D.E.Burkepile, R.L. Vega Thurber, R. Knight, et al. 2013. Predictive functional profiling of microbial communities using 16S rRNA marker gene sequences. Nat. Biotechnol. 31(9):814–21. doi:10.1038/nbt.2676.
- Lebrero, R., E. Rodríguez, J. M. Estrada, P. A. García-Encina, and M. Raúl. 2012. Odor abatement in biotrickling filters: Effect of the ebrt on methyl mercaptan and hydrophobic vocs removal. Bioresource Technol. 109:38–45. doi:10.1016/j.biortech.2012.01.052.
- Ling, Z. H., and H. Guo. 2014. Contribution of VOC sources to photochemical ozone formation and its control policy implication in Hong Kong. Environ. Sci. Policy. 38:180–91. doi:10.1016/j.envsci.2013.12.004.
- Liu, Q., A. E. Babajide, P. Zhu, and L. P. Zou. 2006. Removal of xylene from waste gases using biotrickling filters. Chem. Eng. Technol. 29 (3):320–25. doi:10.1002/ceat.200500132.
- Lu, R. B., Q. P. Du, Y. B. Xu, Y. X. Li, and J. X. Liu. 2016. Biofilm characteristics and microbial diversity in ethylbenzene degradation BTF during the stable operation periods. Acta Scien. Circum. 36 (10):3561–68. doi:10.13671/j.hjkxxb.2016.0107.
- Malakar, S., P. D. Saha, D. Baskaran, and R. Rajamanickam. 2017. Microbial biofilter for toluene removal: Performance evaluation, transient operation and theoretical prediction of elimination capacity. Sustainable Environ. Res. 28 (3):121–27. doi:10.1016/j.serj.2017.12.001.
- Mathur, A. K., J. Sundaramurthy, and C. Balomajumder. 2006. Kinetics of the removal of mono-chlorobenzene vapour from waste gases using a trickle bed air biofilter. J. Hazard. Mater. 137 (3):1560–68. doi:10.1016/j.jhazmat.2006.04.042.
- Nukunya, T., J. S. Devinny, and T. T. Tsotsis. 2005. Application of a pore network model to a biofilter treating ethanol vapor. Chem. Eng. Sci. 60:665–75. doi:10.1016/j.ces.2004.08.038.
- Padhi, S. K., and S. Gokhale. 2016. Benzene control from waste gas streams with a sponge-medium based rotating biological contactor. Int. Biodeter. Biodegr. 109:96–103. doi:10.1016/j.ibiod.2016.01.007.
- Qian, H., Y. Cheng, C. Yang, S. Wu, G. Zeng, and J. Xi. 2018. Performance and biofilm characteristics of biotrickling filters for ethylbenzene removal in the presence of saponins. Environ. Sci. Pollut. R 25 (30):30021–30. doi:10.1007/s11356-017-0776-6.
- Rahul, A. K. Mathur, S. Bala, and C. Majumder. 2012. Modelling and computational fluid dynamic behaviour of a biofilter treating benzene. Bioresource Technol. 200:1089–1089. doi:10.1016/j.biortech.2015.10.033.
- Rene, E. R., D. V. S. Murthy, and T. Swaminathan. 2010. Effect of flow rate, concentration and transient-state operations on the performance of a biofilter treating xylene vapors. Water Air Soil. Poll. 211 (1–4):79–93. doi:10.1007/s11270-009-0282-7.
- Salamanca, D., D. Dobslaw, and K. H. Engesser. 2017. Removal of cyclohexane gaseous emissions using a biotrickling filter system. Chemosphere. 176:97–107. doi:10.1016/j.chemosphere.2017.02.078.
- Singh, D., and M. H. Fulekar. 2015. Biodegradation of petroleum hydrocarbons by Pseudomonas putida strain MHF 7109. CLEAN - Soil, Air, Water. 38 (8):781–86. doi:10.1002/clen.200900239.
- Singh, K., B. S. Giri, A. Sahi, S. R. Geed, M. K. Kureel, S. Singh, S. K. Dubey, B. N. Rai, S. Kumar, S. N. Upadhyay, and R.S. Singh. 2017. Biofiltration of xylene using wood charcoal as the biofilter media under transient and high loading conditions. Bioresource Technol. 242:351–58. doi:10.1016/j.biortech.2017.02.085.
- Song, J., and K. A. Kinney. 2002. A model to predict long-term performance of vapor-phase bioreactors: A cellular automaton approach. Environ. Sci. Technol. 36(11):2498–507. doi:10.1021/es0156183.
- Song, T., C. Yang, G. Zeng, G. Yu, and C. Xu. 2012. Effect of surfactant on styrene removal from waste gas streams in biotrickling filters. J Chem Technol Biotechnol 87 (6):785–90. doi:10.1002/jctb.3717.
- Sun, Y. L., S. Xue, L. Li, W. J. Ding, J. X. Liu, and Y. P. Han. 2018. Sulfur dioxide and o -xylene co-treatment in biofilter: Performance, bacterial populations and bioaerosols emissions. J. Environ. Sci. 69:41–45. doi:10.1016/j.jes.2017.03.039.
- Suzuki, M., T. Hayakawa, J. P. Shaw, M. Rekik, and S. Harayama. 1991. Primary structure of xylene monooxygenase: Similarities to and differences from the alkane hydroxylation system. J. Bacteriol. 173 (5):1690–95. doi:10.1128/jb.173.5.1690-1695.1991.
- Vergara-Fernández, A., S. Hernández, R. Muñoz, and S. Revah. 2012. Influence of the inlet load, EBRT and mineral medium addition on spore emission by Fusarium solani in the fungal biofiltration of hydrophobic VOCs. J. Chem. Technol. Biot. 87(6):778–84. doi:10.1002/jctb.3762.
- Wu, C., P. L. Xu, B. L. Xu, W. Li, S. J. Li, and X. Q. Wang. 2017. o-Xylene removal using one- and two-phase partitioning biotrickling filters: Steady/transient-state performance and microbial community. Environ Technol 39 (1):109–19. doi:10.1080/09593330.2017.1296892.
- Wu, H., C. Y. Guo, Z. H. Yin, Y. Yue, and C. R. Yin. 2018. Performance and bacterial diversity of biotrickling filters filled with conductive packing material for the treatment of toluene. Bioresource Technol. 257:201–09. doi:10.1016/j.biortech.2018.02.108.
- Xu, R. W., Y. C. Xu, S. H. Wei, L. P. Wang, and C. Cao. 2017. Nonionic surfactant-enhanced biodegradation of m-xylene by mixed bacteria. Acta Scien. Circum. 37 (8):3107–13. doi:10.13671/j.hjkxxb.2017.0115.
- Yang, C., H. Qian, and X. Li. 2018. Simultaneous removal of multicomponent vocs in biofilters. Trends Biotechnol. 36 (7):673–85. doi:10.1016/j.tibtech.2018.02.004.
- Yang, C. P., H. Chen, G. M. Zeng, G. L. Yu, and S. L. Luo. 2010. Biomass accumulation and control strategies in gas biofiltration. Biotechnol. Adv. 28 (4):531–40. doi:10.1016/j.biotechadv.2010.04.002.
- Yang, C. P., M. T. Suidan, X. Q. Zhu, and B. J. Kim. 2003. Biomass accumulation patterns for removing volatile organic compounds in rotating drum biofilters. Water Sci. Technol. 48(8):89–96. doi:10.2166/wst.2003.0456.
- Yu, J. M., W. Liu, Z. W. Cheng, Y. F. Jiang, W. J. Cai, and J. M. Chen. 2014. Dichloromethane removal and microbial variations in a combination of uv pretreatment and biotrickling filtration. J. Hazard. Mater. 268:14–22. doi:10.1016/j.jhazmat.2013.12.068.
- Zhao, L., S. B. Huang, and Z. M. Wei. 2014. A demonstration of biofiltration for voc removal in petrochemical industries. Environ. Sci. Proc. Impacts 16 (5):1001. doi:10.1039/c3em00524k.
- Zhu, X. Q., M. T. Suidan, A. Pruden, C. P. Yang, C. Alonso, B. J. Kim, and B. R. Kim. 2004. Effect of substrate Henry’s constant on biofilter performance. J. Air Waste Manage. Assoc. 54 (4):409–18. doi:10.1080/10473289.2004.10470918.