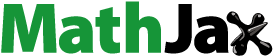
ABSTRACT
Spent drilling mud (DM), a co-product during horizontal directional drilling operations, needs proper disposal to comply with social and environmental sustainability awareness, with one option being its cost-effective reuse as a soil amendment. The possibility was investigated of DM applications to improve the physical quality of loamy sand soil. The soil was treated with increasing DM contents in a laboratory at volumetric DM-to-soil ratios of 0:100 (DM0, no DM), 25:75 (DM25), 50:50 (DM50), 75:25 (DM75), and 100:0 (DM100, no soil). The mixtures were analyzed for water retention, available water capacity, water storage capacity, S-index, soil aggregate stability (SAS), and chemical quality based on pH, effective electrical conductivity (ECe), sodium adsorption ratio (SAR), and exchangeable sodium percentage (ESP). The optimal ratio of DM and the control (DM0) in the batch experiment were selected for application to three species of forest plant seedlings in the field. The results showed that DM positively influenced soil physical qualities. The water retention increased (from 10 to 67%) with increasing DM, but the most suitable ratio was DM25, considering its water storage capacity deviated the least (1.4%) from the optimal value. DM25 also produced the highest S-index (0.081). The SAR data increased with the drilling mud application rate (1.1 to 10.9). However, the DM25 pH remained at 7.7, with an ECe of 2.5 dS m−1, a SAR of 7.0, and an ESP of 9.2, which were still favorable regarding soil structure, as indicated by no decrease in SAS with added drilling mud. In the field experiment, DM25 also decreased the water deficit of the three species of forest plant seedlings, suggesting a positive attribution to other relevant soil-plant systems. DM could be a feasible option to improve soil physical quality, but further long-term experiments are necessary before applying it as a soil amendment in real situations.
Implications: With the demand in energy-driven economics, establishing national energy security nationwide in Thailand requires the installation capacity, transmission, and distribution pipelines. Horizontal directional drilling (HDD) operations are generally used to install underground pipelines, generating large amounts of drilling mud rich in sodium bentonite as a co-product. At present, drilling mud is becoming a more serious solid waste and has been raised in proper management. The best management practices are expected to reuse as a soil amendment, a cost-effective approach, for coarse-textured soils. Before any drilling mud application can be introduced to agriculture and the environment, we must clarify the optimal rate of drilling mud. This rate has to significantly improve soil physical quality associated with air-water capacity balance while minimizing the adverse effect of residual sodium to favor soil structure and sensitive plants.
Introduction
The oil and gas industry is expanding worldwide due to increasing demand. This has resulted in increasing the installation capacity, transmission, and distribution pipelines that need horizontal directional drilling (HDD) to set up underground pipelines while minimizing open-cut trenches and surface disturbance. Generally, HDD operations use large volumes of water-based sodium-bentonite slurry as a lubricant to cool the drill bit, maintain borehole pressure, and bring the drill cuttings to the surface (Ukeles and Grinbaum Citation2004), thereby generating large amounts of spent drilling mud. In Thailand, PTT Public Company Limited (PTT) has played a vital role in establishing national energy security since 1981. According to its current operations, drilling mud is estimated to be 12,716 m3 during 3.5 years of construction. This drilling mud needs proper disposal or effective management to comply with the recognized importance of social and environmental sustainability of such projects.
Utilization of the spent bentonite mud as a soil amendment is a cost-effective means for its disposal. Yet, little has been reported about the effects of spent drilling mud on soil quality and productivity and few studies have focused on its potential to improve the pH buffering capacity of acid sulfate soils (Chittamart et al. Citation2018) and to alleviate nutrient imbalances in the paddy rice-acid sulfate soil system when used in conjunction with dolomite (Wongleecharoen et al. Citation2020). However, there is limited reporting on the effects of drilling mud that is rich in bentonite on the physical quality improvements of coarse-textured soils.
Soil physical quality is essential for sustainable agriculture and environmental protection. Vizitiu et al. (Citation2011) defined the two main structural features: (1) the soil must stable in water, and (2) the soil must have a suitable pore size distribution that will enhance the soil balance of air and water for plant use. Soil aggregate stability (SAS) is defined as the soil’s ability to stabilize the structure against disruptive forces derived from either natural or artificial sources (Amézketa, Citation1999). SAS influences the soil pore geometry and continuity, thereby controlling the fate and transport of mass and energy in a soil system (Herrick et al. Citation2001). Consequently, it is commonly used as an index determining soil structure and erosion risk (Moncada et al. Citation2013). S-index is a single indicator of soil microstructural porosity, which is the slope of the soil water retention curve (SWRC) at its inflection point (Dexter Citation2004a). It can be used in determining the soil physical quality for different soil horizons, soil types, and spatial variations (Fenton et al. Citation2017). The balance between the water holding capacity and aeration is also a good indicator of soil physical quality and is known as the water storage capacity. This indicator is defined as the soil moisture at field capacity divided by the soil porosity (FC/Pt), and it achieves the optimal balance when FC/Pt is about 0.66 (Reynolds et al. Citation2002).
Several reports showed that bentonite could increase available plant water for sandy soils because of increasing soil porosity (Soda et al. Citation2006). At a rate of 4%, bentonite increased the water content of the sandy soils in Northeast Thailand. (Masazumi et al. Citation2016). Churchman, Noble, and Chittleborough (Citation2012) also confirmed that the mud (including bentonite) could promote plant growth on degraded sandy soils. Increased water retention in coarse-textured soils is critical, especially in the rainfed area, where plants are subject to a water deficit. At a suitable ratio, sodium bentonite could alleviate the physical properties of lateritic soils (Nur et al. Citation2016). Therefore, positive benefits could be expected when using drilling mud (bentonite-based materials) to improve the physical quality associated with soil structure and hydrology, especially for the sandy soils that cover 13,760 km2 in Northeast Thailand.
Our study aimed to assess the feasibility of bentonite drilling mud to improve (i) the physical quality of loamy sand soil and (ii) the water deficit of forest plant seedlings. We expected to optimize the drilling mud-to-soil ratio that provides the water storage capacity to relieve the plant water deficit while maintaining the soil salinity and sodium adsorption ratio (SAR) below levels considered harmful to sensitive plants and soil structure.
Materials and methods
Soil and drilling mud sampling and their preparation
The soil was selected from the forest area in Kaset Wisai district, Roi Et province (15°39′24″N 103°34′48″E), in central, Northeastern Thailand. The soil was classified as a loamy, siliceous, isohyperthermic Arenic Paleustalfs, according to USDA Soil Taxonomy, namely the Ban Phai Series. The drilling mud (DM) used in this experiment was collected from the drilling mud dumping site of the PTT area located in Bang Pa-in district, Phra Nakhon Si Ayutthaya province (14°15ʹ18.2″N 100°34ʹ37.5″E). This DM had an initial bulk density of 1.11 g cm−3 and a moisture content of 50% by weight, whereas the studied soil had a bulk density of 1.51 g cm−3. The DM materials (with the aggregate size and water content as they were at collection) were mixed with the sandy soil (<2 mm aggregate size diameter) with the volumetric DM-to-soil ratios of 0:100 (only sandy soil), 25:75, 50:50, 75:25, and 100:0 (only DM) cm3 cm−3, labeled as DM0, DM25, DM50, DM75, and DM100, respectively. The total amount of each mixture treatment was 1,000 cm3. This mixture was based on a volumetric ratio to simplify field application purposes. However, for the reproducibility of the soil mixed with any inconsistent moisture content in the DM, the dry mass DM-to-soil ratio (DM0-to-DM100 ratio) was measured before the mixing step, which was equivalent to 0:100, 16:100, 49:100, 147:100, and 100:0 g g−1, respectively. All the treatments were air-dried and sieved to a diameter less than 2 mm and then analyzed for their basic physical and chemical properties according to standard methods (National Soil Survey Center Citation1996), as shown in . The exchangeable sodium percentage (ESP), was calculated based on the linear regression model ESP = 1.95 + 1.03 SAR (R2 = 0.92), as reported by Seilsepour, Rashidi, and Khabbaz (Citation2009) to estimate ESP from SAR. Parts of the soil-DM mixtures were also separately kept with minimal disturbance before further determining the physical quality.
Table 1. Some physical and chemical properties of spent drilling mud mixed with coarse-textured soil samples
Soil water retention curve and S-index
After mixing the drilling mud, the air-dried mixture samples with a diameter less than 2 mm were fully placed on a ring (height 1 cm and diameter 5 cm) with its volume equivalent to the mixture’s volume before putting on a soaked-porous ceramic plate to be fully saturated by the rising capillary. The saturated samples were placed in a pressure plate apparatus (Combo 1500F1&1600 Extractors, Soilmoisture Equipment Crop., Santa Barbara, CA, USA) to release water at given pressure heads () of −51, −102, −337, −1020, −3,059, and −15,296 cm H2O, respectively, which are equivalent to pF values of approximately 0 to 4.2. This range of pressure heads or pF is typical for full consideration of the S-index as previously studied in Vizitiu et al. (Citation2011). In this experiment, we assumed the released water at −1 cm H2O equivalent to the saturated volumetric water content, according to Landon (1991). The observed experimental data were compiled using the van Genuchten model (van Genuchten Citation1980) and maintaining the Mualem restriction (m = 1– 1/n) facilitated by the RETC program (van Genuchten, Leij, and Yates Citation1991) to generate the water retention curve using EquationEq. (1)
(1)
(1) :
Where, and
(cm3 cm−3) are the saturated volumetric water content and the residual volumetric water content, respectively, and
,
, and
are fitting-curve parameters (Radcliffe and Šimunek Citation2010).
After RETC is used to solve for
, the S-index (generally defined as the slope of the water retention curve at its inflection point) can be calculated using EquationEq. (2
(2)
(2) ), as an absolute value (Dexter Citation2004a):
The boundary between a soil with good or poor physical quality is proposed at an S-index of 0.035 and above, whereas a soil with very poor physical quality is defined by an S-index below 0.02 (Dexter Citation2004a).
Water storage capacity index
Before determining the index of water storage capacity, the associated parameters had to be quantified. These parameters consisted of: total porosity (), bulk density (
), particle density (
), field capacity (
), permanent wilting point (
), and available water capacity (
) (Oliveira et al., Citation2014). In this study, the porosity was divided into soil macropores (
) (matrix suction range of 0 to – 10 cm H2O) and soil matrix pores (
) (matrix suction range of ≤ –10 cm H2O). These porosity indices and moisture limits can be obtained from the water retention curve at the specific pressure head (cm unit) and calculated as using EquationEq. (3)
(3)
(3) to EquationEq. (8)
(8)
(8) based on the similar concept of Reynolds et al. (Citation2002):
where is the volumetric water content at the given pressure head
. With all relevant parameters above, water storage capacity can be calculated using EquationEq. (9)
(9)
(9) :
The obtained values were compared with the optimal value of 0.66, based on Reynolds et al. (Citation2002).
Soil aggregate stability
The air-dried soil aggregates that had been treated with drilling mud were sieved to an index diameter of 1–2 mm to analyze the SAS according to Kemper and Rosenau (Citation1986). Before the analysis, these soil aggregates were incubated and moisturized by vapor at the pressure equivalent to the matrix potential of water at field capacity above the salt solution in a closed chamber for 48 h, to avoid the slaking effect (Kemper and Rosenau Citation1986; Phocharoen et al. Citation2018). Then, the SAS was determined using a wet sieving apparatus (Eijkelkamp, Thai Victory, Bangkok, Thailand) with a single sieve size of 250 µm. The samples were put into the sieve container before placing them on the sample holder of a wet sieving apparatus and being vertically shaken at a working distance of 1.3 cm at a constant 35 rpm for 3 min. The aggregate samples remained on the sieve, which was resistant to wet sieving, excluding the particles larger than 250 µm (sand particles and organic materials) that were defined as the stable parts. The rest of the suspension with a diameter smaller than 250 µm were defined as the unstable parts. After that, both sample parts were oven-dried at 105°C and weighed. The SAS was determined using EquationEq. (10)(10)
(10) :
where and
are the dry weights of the stable parts and unstable parts, respectively.
Field experiment
Seedlings of Yang Na (Dipterocarpus alatus), Padauk (Pterocarpus macrocarpus), and Phayung (Dalbergia cochinchinensis) were planted on July 13th, 2020, at the same site where the studied soils were sampled. Two treatments with four replications of each plant were established. The plot size per one plant was 2 m × 4 m. One treatment was used as the control (without drilling mud applications) and the second was the most suitable drilling mud-to-soil ratio, based on the batch experiment. The selected treatment criteria were considered from the mixture treatment properties, having the least adverse effects on pH, ECe, SAR, ESP, and the most positive attributes of water retention, S-index, water storage capacity, and SAS. Based on these criteria, DM25 was chosen for the field experiment. During the growing period of 6 months, the plant height and circumference were recorded once each month on the same plant. The relative height and circumference with respect to the day of planting were used to determine the growth trend across the control and drilling mud treatments. After planting for 6 months, the water deficit (WD) in plant leaves at maturity, according to Barrs (Citation1968), as shown in EquationEq. (11)(11)
(11) .
Statistical analysis
Statistical analysis was performed using the R software package (R Core Team Citation2021), with one-way ANOVA and LSD test at the significance level of α = 0.05. The mean comparison of the relative water deficit in mature plant leaves between the control and drilling mud treatments was also evaluated using a t-test at α = 0.05.
Results
Water retention functions
demonstrates the soil water retention curves (SWRCs) as influenced by succeeding drilling mud levels. The observed data had an excellent fit to the van Genuchten model maintaining the Mualem restriction (lines), with a high coefficient of determination (R2) ranging from 0.985 to 0.999. As expected, the DM0 (loamy sand without drilling mud) had the least water retention for all the given pressure head ranges, whereas DM100 (only drilling mud) had the highest. The other curves (DM25 to DM75) fell between the upper and lower treatments, with water retention increasing for the given pressure heads with increasing drilling mud. The shapes of the SWRCs could be generally categorized into two groups. The first had an exponential decay, consisting of DM100, DM75, and DM50, where the water retention was relatively high at initial saturation but suddenly decreased with a minimal change of pressure head (). The second was a sigmoidal function (DM0 and DM25), reflecting a typical medium-textured soil as the curves in the capillary region changed over a wide range in
. The SWRCs of DM25 to DM100 retained higher water than that of DM0 at the same given
range, suggesting that drilling mud positively improved the water retention in the studied soils. Compared to the water retention curve of DM0, those of DM25, DM50, DM75, and DM100 had relative increase of 10%, 20%, 37%, and 67%, respectively.
Figure 1. Water retention curves of drilling mud-treated soil samples. Symbols represent observed experimental data, and lines indicate full function predicted using RETC with the van Genuchten model (van Genuchten Citation1980) maintaining the Mualem restriction (m = 1– 1/n)
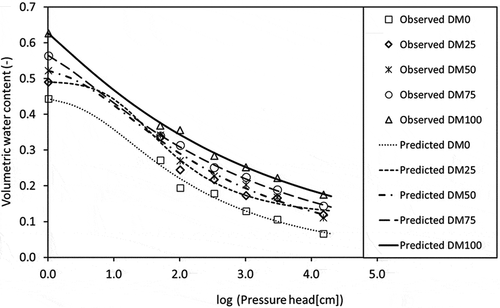
Porosity, available water capacity, and water storage capacity index
The data of porosity, moisture limits (FC and PWP), available water capacity (AWCA), and water storage capacity index are presented in . The total porosity () values were in the range 0.393–0.425 with no significant differences. The porosity was significantly (α = 0.05) distributed less in macropores (
range 0.056–0.295) and more in matrix pores (
range 0.363–0.469). The sum of the
and
values was not compensated for but was slightly higher than
because some other tiny pores were not included, such as ultrapores that were hardly saturated due to air-trapped phenomena. The moisture limit at FC was significantly different and linearly increased with increased drilling mud (0.221–0.344). Correspondingly, the moisture limit at PWP also increased with additional drilling mud (0.075–0.178). The difference between FC and PWP is AWCA; therefore, it was not significantly different because both moisture limits were lifted by the drilling mud. However, we did observe an increase in AWCA when the drilling mud was applied at DM50 and above.
Table 2. Mean values (± standard deviation, three replications) of porosity indices, field capacity (FC), permanent wilting point (PWP), available water capacity (AWCA), and water storage capacity (FC/Pt) of coarse-textured soils with added drilling mud (DM)
Water storage capacity (FC/Pt) also increased with drilling mud application rates, ranging from 0.519 to 0.833. According to Reynolds et al. (Citation2002), the critical water storage capacity is 0.66, where this number positively reflects water capacity balance with air capacity. Based on this classification, the relative deviation of water storage capacity respective to the balance capacity of 0.66 is demonstrated in . Without drilling mud (DM0), the original loamy sand had the least water storage capacity compared with the other treatments and deviated the most from the balance value. Drilling mud could have increased the water storage capacity of the studied soils (DM25 to DM100), but excess drill mud addition could have induced excessive water capacity. Based on this batch experiment, the treatment of DM25 was the optimal for field applications as indicated by the least deviation (+1.4%) from the critical value of 0.66.
Figure 2. Relative deviation of water storage capacity (field capacity/total porosity, FC/Pt) of the spent drilling mud-treated soils from optimal value of 0.66, based on Reynolds et al. (Citation2002)
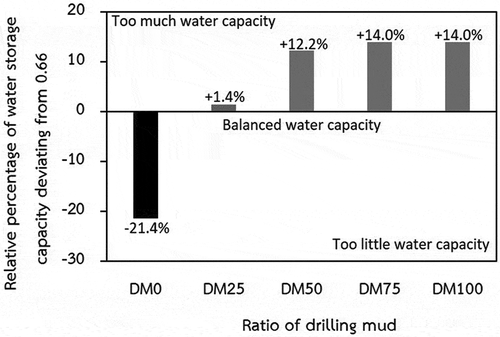
S-index
shows the hydraulic parameters, fitting curve parameters, and S-index values. Generally, all S-index values under the different drilling mud applications ranged from 0.063 to 0.081 and could all be classified as good soil physical quality based on Dexter (Citation2004a). The soil without drilling mud already had a high S-index of 0.063, suggesting no physical limitations associated with root functions. When the soils were treated with increasing drilling mud, the S index did not correspondingly increase but could result in a similar soil physical quality status. However, it should be noted that the treatment of DM25 caused the highest S-index (0.081), agreeing with the result for water storage capacity mentioned above.
Table 3. Hydraulic parameters, fitting curve parameters, and predicted S-index values of coarse-textured soils mixed with spent drilling mud. Data represent the mean ± standard deviation from three replications
Soil aggregate stability
Drilling mud also had a positive influence on the aggregate stability of the studied soils. shows that SAS increased with increased drilling mud. Noticeably, SAS significantly and sharply rose from 26.2% to 61.1% in the drilling mud treatments DM75 to DM100. However, considering the SAS classification, according to Moncada et al. (Citation2013), even the drilling mud (DM100), which had the highest SAS, still only was in the intermediate level. This result suggested that the studied soil was poor in aggregate stability and it would be challenging to improve this without other cementing agents, such as organic matter. Nonetheless, it confirmed that the selected DM25 did not further reduce SAS, indicated by similar results compared to the control treatment.
Figure 3. Soil aggregate stability (SAS) with error bars showing ± standard deviation from three replications of each treatment of studied soils with added drilling mud (DM) at DM-to-soil volumetric ratios of 0:100 (DM0), 25:75 (DM25), 50:50 (DM50), 75:25 (DM75), and 100:0 (D100). Stability classification is defined as good (SAS >70%), intermediate (50% ≤ SAS ≤70%), and poor (SAS < 50%) according to Moncada et al. (Citation2013). Values in each histogram, followed by the same letter, are not significantly different at α = 0.05 (LSD method)
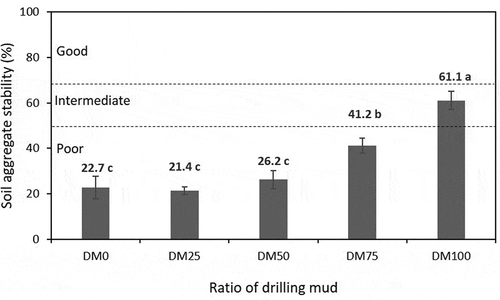
Growth and water deficit of forest plant seedlings
The DM25 treatment was selected as the most suitable treatment based on the least adverse effects of DM on the soil mixture pH, ECe, SAR, and ESP () and also on the most positive effects of water retention (), water storage capacity ( and ), S-index (), and SAS (). The DM25 and DM0 (control, only soil) treatments were prepared in the field to grow forest plant seedlings. The growth response of the forest plant seedlings with the DM25 and DM0 treatments is presented in . The overall growth with and without drilling mud was trended higher as a function of time. Compared to the control treatment, the positive response in relative growth varied among the plant species. The relative height and grith with respect to the planting day of D. alatus ( and d) had the most favorable responses to the drilling mud applications, followed by D. cochinchinensis ( and f), with the least response for P. macrocarpus ( and e).
Figure 4. Relative height (%) of D. alatus (a), P. macrocarpus (b), and D. cochinchinensis (c) and their relative girth (%), corresponding to same plant species (d), (e), and (f) for a growth period of 6 months. Solid lines and filled circles represent values from the control (no drilling mud addition), whereas dashed lines and unfilled circles indicate data from DM25 (drilling mud-to-soil ratio = 25:75 by volume). The symbols “(*)” and “ns” indicate significantly and not significantly different, respectively, at α = 0.05 (paired t-test)
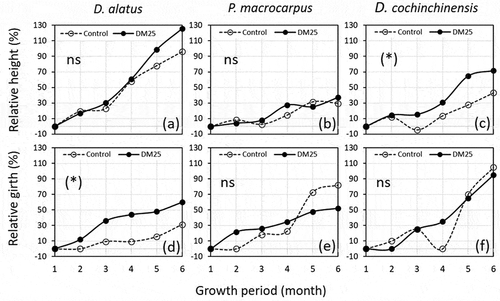
shows the water deficit (%) of plant leaves after the DM25 drilling mud application compared to the control treatment. The water deficit was the greatest for P. macrocarpus, followed by D. alatus and D. cochinchinensis. This water deficit decreased when the soil was mixed with drilling mud before planting the seedlings in the 10–20.7% to 6.9–16.9%, but there was no significant difference in water deficit between the two treatments.
Figure 5. Water deficit percentage of plant leaves after drilling mud (DM) was applied with drilling mud-to-soil ratio equivalent to 25:75 by volume (DM25) versus the control (without DM) for D. alatus, P. macrocarpus, and D. cochinchinensis. The mean comparison between the control and DM25 of each plant was not significant at α = 0.05 based on a t-test. Error bars indicate ± standard deviation based on four replications
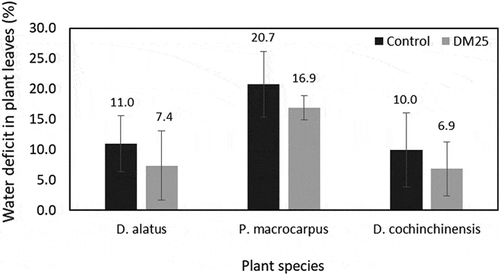
Discussion
In this experiment, we determined the physical quality indices of the drilling mud-soil mixture, consisting of water retention, available water capacity, water storage capacity, S-index, and SAS, together with the chemical quality based on the pH, effective electrical conductivity (ECe), sodium adsorption ratio (SAR) and exchangeable sodium percentage (ESP), as shown in . For the given DM treatments, DM25 had higher water retention and available water capacity, with water storage capacity approaching the balance index (0.66), the highest S-index, and no adverse impact on SAS (, to 3), suggesting positive responses regarding the soil physical quality. The DM25 treatment was the optimal rate of drilling mud to improve the physical constraints in loamy sand soil. The DM25 soil mixture had a pH of 7.7, ECe of 2.5 dS m−1, SAR of 7.0, and ESP of 9.2 (). Therefore, this chosen treatment did not result in the soil mixture being classified in any type of salt-affected soil based on Soil Survey Division Staff (Citation1993), providing another support regarding the addition of DM not adversely affecting the soil structure.
The water retention pattern of DM25 had a similar shape but higher retention than the control (). Therefore, it increased the water retention in the loamy sand soil without changing its physical characteristics. When the water retention curve changes over a wide range of pressure heads, it tends toward a broad pore-size distribution, thereby causing the water retention to be in balance with aeration (Radcliffe and Šimunek Citation2010). This result implied that the typical permeability of coarse-textured soils was less affected by adding more clay-sized particles. This observation also agreed with the water storage capacity index, which deviated the least from the critical value of 0.66 ( and ). Reynolds et al. (Citation2002) suggested that the water storage capacity of 0.66 was necessary to balance air storage capacity at 0.34. Therefore, the more positive deviation of the water storage capacity from 0.66 could also result in the soil air storage capacity to more negatively deviate from 0.34, resulting in a poor aeration condition. Thus, it would be crucial to note that applying drilling mud to improve water holding capacity must be done cautiously and at the optimal rate to avoid excess much water capacity in coarse-textured soils, especially in a wet season.
All treatments resulted in the values of the S-index over the critical value of 0.035 (), with the DM25 treatment producing the highest value of 0.081. S-index is generally used to describe the soil microstructural pore geometry derived from various abiotic and biotic factors (Dexter Citation2004a) related to plant root functions (Dexter Citation2004b). This index could also be used to determine soil physical quality status across the spatial variation of soil types (Fenton et al. Citation2017). Our results suggested that drilling mud positively influenced soil pores, and that the DM25 treatment provided the most favorable physical quality associated with plant root function.
Generally, the amount of sodium in drilling mud is one of the major concerns regarding soil structure failure. Many reports have revealed the negative impacts of sodium on soil structural behaviors (Arienzo et al. Citation2012; Quirk and Schofield Citation1955; Rengasamy and Marchuk Citation2011; Smiles Citation2006; Smiles and Smith Citation2004). In the current study, for the original drilling mud (DM100), the SAR and ESP values were 10.9 and 13.2, respectively. However, these sodium activity parameters with respect to other basic cations were reduced to 7.0 and 9.2, respectively, after mixing with the studied soils at the rate of DM25 (). The SAR values were much lower than the original sodium bentonite before use in the HDD technique, as reported by Wongleecharoen et al. (Citation2020), where the SAR value was 28.8.
If sodium in the drilling mud dispersed clay particles and demoted the soil structure, SAS would decrease with additional drilling mud. However, the current results indicated that SAS increased with increasing amounts of drilling mud, suggesting the drilling mud used in this experiment had less of a negative influence from the residual sodium. Therefore, the improvement of SAS was possibly caused by increasing the clay particle contents corresponding with the larger amounts of drilling mud (). Generally, clay-sized particles are essential for soil aggregation (Amézketa Citation1999). Furthermore, clay particles forming a clay domain are prerequisites for enhancing soil aggregation and stability (Dexter Citation1988). The positive attributes of adding drilling mud in our study agreed with several studies that used bentonite to improve the physical properties of sandy soils (Churchman, Noble, and Chittleborough Citation2012; Masazumi et al. Citation2016; Nur et al. Citation2016; Soda et al. Citation2006).
The dynamics of SAS can be related to several factors, including the clay content and electrolytes (Amézketa, Citation1999). Levy, Mamedov, and Goldstein (Citation2003) studied the effects of sodicity and water quality on the aggregate breakdown mechanism of semi-arid soils. Based on their work, the soil with low aggregate stability (clay < 25%), increasing electrolyte concentration could demote slaking at ESP < 5, whereas in the soil with clay >35%, the electrolyte concentration decreased aggregate slaking only when ESP >15. Therefore, ESP, the electrolyte concentration, and the clay content contributed to their combined effects on slaking soil aggregates, suggesting that they need to be considered simultaneously to influence SAS (Levy, Mamedov, and Goldstein Citation2003). We also found such combined effects in our experiments regarding the DM0, DM25, and DM50 treatments, where the aggregate stability values were similar and were classified as poor stability. In this case, the clay content only increased from 6.4 to 16.2% with increasing ESP of 3.1 to 13.2%. Consequently, the SAS data did not increase with increasing ECe from 0.2 to 3.6 dS m−1. We observed that the combination effects started contributing to the increase in SAS when the clay content was added in the drilling mud in the DM75 treatment, where the mixture had a clay content of 26.3%, an ECe of 4.3 dS m−1, and an ESP of 12.0.
The positive response of the forest plant seedlings to drilling mud applications compared to the control () also agreed with the positive effects of drilling mud on higher water retention, water storage capacity, and S index from the batch experiments. The response levels among plant species varied. For example, P. macrocarpus needs further investigation using a more sophisticated ratio, falling between DM0 and DM25, as the relative growth of this plant was not significantly different from the control in any given growth period ( and e). Even though there was no significant difference, the positive influence of drilling mud on the studied soils associated with plant water uptake was promising, with diverse degrees of response across plant species (). The difference between the DM25 and control treatments was somewhat lower than we expected. This result could be explained by unexpected rain events only a few days before collecting plant leaves, which may have normalized the different water deficit results across the two treatments. Subsequently, we speculated that the plant water deficit induced by drilling mud applications would produce a more pronounced decrease in a drier season. In this work, using bentonite drilling mud as a soil amendment was a highly feasible way to improve plant water deficit in loamy sand soil. However, this study was a short-term one. Indeed, long-term studies of the impact of using drilling mud mixtures on soil quality are necessary before it can be recommended as a suitable amendment.
Conclusion
The DM25 treatment (volumetric drilling mud-to-soil ratio of 25:75) was the most suitable ratio for enhancing the water storage capacity to approach the optimal value, with the highest S-index of 0.081. Although the SAR values increased with the drilling mud application rate, they remained below levels considered unfavorable to soil structure, which was supported by the results of soil aggregate stability increased with increasing amounts of drilling mud. Correspondingly, the DM25 treatment alleviated the water deficit in forest plant seedlings in the field trial. Sandy soils that are subject to water deficit cover 13,760 km2 in Northeastern Thailand, where the positive attributions of drilling mud are promising for improving the soil physical quality. Our work highlighted that bentonite drilling mud has high potential for the physical quality improvement of coarse-textured soils. However, further experiments in the long-term are needed before it can be broadly applied as a soil amendment.
Acknowledgment
The authors thank Dr.Visoot Verasan, Kasetsart University, Kamphaeng Saen Campus, Thailand, for valuable comments and suggestions regarding water retention curves and their relevant functions. Special thanks go to Miss Prapaipit Srimawong and Miss Thanyaporn Panawong for their substantial assistance with the batch experiments and data analysis.
Disclosure statement
No potential conflict of interest was reported by the author(s).
Additional information
Notes on contributors
Saowanuch Tawornpruek
Saowanuch Tawornpruek Associate Professor of Soil Science.
Surachet Aramrak
Surachet Aramrak Assistant Professor of Soil Science.
Daojarus Ketrot
Daojarus Ketrot Assistant Professor of Soil Science.
Chalermchart Wongleecharoen
Chalermchart Wongleecharoen Lecturer of Soil Science.
Worachart Wisawapipat
Worachart Wisawapipat Associate Professor of Soil Science.
Natthapol Chittamart
Natthapol Chittamart Assistant Professor of Soil Science.
Kittiphong Songrukkiat
Kittiphong Songrukkiat Manager of Project Environmental Management Department-PTT.
Suradanai Augsorntung
Suradanai Augsorntung Engineer of Project Environmental Management Department-PTT.
References
- Amézketa, E. 1999. Soil aggregate stability: A review. J. Sustain Agr. 14 (2–3):83–151. doi:https://doi.org/10.1300/J064v14n02_08.
- Arienzo, M., E. W. Christen, N. S. Jayawardane, and W. C. Quayle. 2012. The relative effects of sodium and potassium on soil hydraulic conductivity and implications for winery wastewater management. Geoderma 173-174:303–10. doi:https://doi.org/10.1016/j.geoderma.2011.12.012.
- Barrs, H. D. 1968. Determination of water deficits in plant tissue. In Water deficits and plant growth, ed. T. T. Kozlowski, Vol. 1, 235–368. New York: Academic Press.
- Chittamart, N., S. Tawornpruek, D. Ketrot, S. Aramrak, K. Chittanukul, and R. Sattapun. 2018. Utilization of nabentonite to improve pH-buffering capacity of acid sulfate soils in natural gas transmission pipeline rights-of-way, Thailand. In IOP Conference Series: Earth and Environmental Science, 12023. IOP Publishing. doi:https://doi.org/10.1088/1755-1315/151/1/012023.
- Churchman, J., A. Noble, and D. Chittleborough 2012. Addition of clay and clay minerals to enhance the sequestration of carbon in soils. Australian Regolith and Clays Conference, 117–20, Mildura, Australia.
- Dexter, A. R. 1988. Advances in characterization of soil structure. Soil Till. Res. 11 (3–4):199–238. doi:https://doi.org/10.1016/0167-1987(88)90002-5.
- Dexter, A. R. 2004a. Soil physical quality Part I. Theory, effects of soil texture, density, and organic matter, and effects on root growth. Geoderma 120 (3–4):201–14. doi:https://doi.org/10.1016/j.geoderma.2003.09.004.
- Dexter, A. R. 2004b. Soil physical quality Part II. Friability, tillage, tilth, and hard-setting. Geoderma 120 (3–4):215–25. doi:https://doi.org/10.1016/j.geoderma.2003.09.005.
- Fenton, O., S. Vero, R. P. O. Schulte, L. O’Sullivan, G. Bondi, and R. E. Creamer. 2017. Application of Dexter’s soil physical quality index: An Irish case study. Irish J. Agr. Food Res. 56 (1):45–53. doi:https://doi.org/10.1515/ijafr-2017-0005.
- Herrick, J. E., W. G. Whitford, A. G. De Soyza, J. W. Van Zee, K. M. Havstad, C. A. Seybold, and M. Walton. 2001. Field soil aggregate stability kit for soil quality and rangeland health evaluations. Catena 44 (1):27–35. doi:https://doi.org/10.1016/S0341-8162(00)00173-9.
- Kemper, W. D., and R. C. Rosenau. 1986. Aggregate stability and size distribution. In Methods of soil analysis: Part 1 physical and mineralogical methods, ed. A. Klute, 425–42. Wisconsin: Soil Science Society of America.
- Levy, G. J., A. I. Mamedov, and Goldstein. 2003. Sodicity and water quality effects on slaking of aggregates from semi-arid soils. Soil Sci. 168 (8):552–62. doi:https://doi.org/10.1097/01.ss.0000085050.25696.52.
- Masazumi, K., N. Suchat, H. Sutjaporn, Y. Reiji, W. Wilawan, H. Woraphun, V. Tosporn, and N. Iwao. 2016. Effects of bentonite, charcoal and corncob for soil improvement and growth characteristics of teak seedling planted on Acrisols in northeast Thailand. J. For. 7 (36):2–21.
- Moncada, M. P., D. Gabriels, W. Cornelis, and D. Lobo. 2013. Comparing aggregate stability tests for soil physical quality indicators. Land Degrad Dev. 26 (8):843–52. doi:https://doi.org/10.1002/ldr.2225.
- National Soil Survey Center. 1996. Soil survey laboratory methods manual: Soil survey investigation report no. 42, version 3.0. Washington: Natural Conservation Service.
- Nur, A., A. Nor, M. Mazidah, and A. Siti Nur 2016. Effect on physical properties of laterite soil with difference percentage of sodium bentonite. Penang, Malaysia: International Conference on Applied Physics and Engineering.
- Oliveira, T. C., L. F. S. da Silva, M. Cooper. 2014. Evaluation of physical quality indices of a soil under a seasonal semideciduous forest. Rev. Bras. Ciênc. Solo 38:444–453. doi:https://doi.org/10.1590/S0100-06832014000200009.
- Phocharoen, Y., S. Aramrak, N. Chittamart, and W. Wisawapipat. 2018. Potassium influence on soil aggregate stability. Commun. Soil Sci. Plan. Anal. 49 (17):2162–74. doi:https://doi.org/10.1080/00103624.2018.1499752.
- Quirk, J. P., and R. K. Schofield. 1955. The effect of electrolyte concentration on soil permeability. J. Soil Sci. 6 (2):163–78. doi:https://doi.org/10.1111/j.1365-2389.1955.tb00841.x.
- R Core Team. 2021. R: A language and environment for statistical computing. R Foundation for Statistical Computing, Vienna, Austria. http://www.R-project.org/.
- Radcliffe, D. E., and J. Šimunek. 2010. Soil physics with HYDRUS: Modeling and applications. Boca Raton: CRC Press Taylor & Francis Group.
- Rengasamy, P., and A. Marchuk. 2011. Cation ratio of soil structural stability (CROSS). Soil. Res. 49 (3):280–85. doi:https://doi.org/10.1071/SR10105.
- Reynolds, W. D., B. T. Bowman, C. F. Drury, C. S. Tan, and X. Lu. 2002. Indicators of good soil physical quality: Density and storage parameters. Geoderma. J. 110 (1–2):131–46. doi:https://doi.org/10.1016/S0016-7061(02)00228-8.
- Seilsepour, M., M. Rashidi, and B. G. Khabbaz. 2009. Prediction of soil exchangeable sodium percentage based on soil sodium adsorption ratio. Am-Euras. J. Agric. Environ. Sci. 5 (1):1–4.
- Smiles, D. E. 2006. Sodium and potassium in soils of the Murray-Darling basin: A note. Aust. J. Soil Res. 44 (7):727–30. doi:https://doi.org/10.1071/SR06057.
- Smiles, D. E., and C. J. Smith. 2004. A survey of the cation content of piggery effluents and some consequences of their use to irrigate soils. Aust. J. Soil Res. 42 (2):231–46. doi:https://doi.org/10.1071/SR03059.
- Soda, W., A. D. Noble, S. Suzuki, R. Simmons, and L. Sindhusen. 2006. Effects on soil properties and crop biomass. J. Environ. Qual. 35 (6):2293–301. doi:https://doi.org/10.2134/jeq2005.0455.
- Soil Survey Division Staff. 1993. Soil Survey Manual. USDA Handbook No. 18. U.S. Washington D.C: Government Printing Office.
- Ukeles, S. D., and B. Grinbaum. 2004. Drilling fluids. Kirk-Othmer Encyclopedia of Chemical Technology. Hoboken, NJ: John Wiley & Sons.
- van Genuchten, M. 1980. A closed-form equation for predicting the hydraulic conductivity of unsaturated soils. Soil Sci. Soc. Am. J. 44 (5):892–98. doi:https://doi.org/10.2136/sssaj1980.03615995004400050002x.
- van Genuchten, M., F. J. Leij, and S. R. Yates. 1991. The RETC code for quantifying the hydraulic functions of unsaturated soils. Oklahoma: U.S. Environmental Protection.
- Vizitiu, O., I. Calciu, I. Pănoiu, and C. Simota. 2011. Soil physical quality as quantified by S index and hidrophysical indices of some soils from Argeş hydrographic basin. Res. J. Agr. Sci. 43:249–56.
- Wongleecharoen, C., W. Wisawapipat, D. Ketrot, N. Chittamart, S. Aramrak, K. Chittanukul, R. Sattapun, and S. Tawornpruek. 2020. Elemental dynamics in porewater of an acid sulfate paddy soil as affected by sodium bentonite and dolomite amendments: Insights from field study. E3S Web Conf. 167 02003. doi:https://doi.org/10.1071/SR0305910.1051/e3sconf/202016702003.