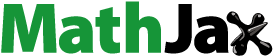
ABSTRACT
Current treatment processes for the cold rolling emulsion wastewater were in the dilemma that the cost was so high while the efficiency was not satisfied in China. In this work, a novel material with high-performance demulsification was obtained and used to treat the emulsion wastewater efficiently by modifying blast furnace dust (BFD), a steel industry waste. Firstly, the BFD was characterized by various analytical techniques and the results suggested that hydrophobic functional groups, positively charged iron oxide, and polysilicon in the BFD were contributed to removing stable oil droplets. Therefore, the BFD modification was conducted accordingly by optimizing the proportion and reaction conditions of these three components. The study demonstrated that the removal rate of oil and CODcr reached 75.21% and 81.23% under the conditions of the carbon type of GWF-HAC-R90, the carbon content of 14.86%, and the n(Fe)/n(Si) of 1.55, respectively. Based on this, the effects of pH, demulsifier dosage, and average agitation rate were investigated. The emulsion components before and after demulsification were analyzed by GC-MS, and the demulsification mechanism was expounded combined with kinetics. Results showed that the Fe2O3 with positive charge played a dominant role in emulsions with mainly anionic surfactants, while hydrophobic carbon structures and polysilicate acted as the auxiliaries. Besides, comprehensive analysis and characterization results suggested that the demulsification effect was a combination of the synergic processes: 1) electrostatic interaction developed by the anionic surfactant oil droplets and the positively charged the BFD particles; 2) hydrophobic association among the oil droplet with nonionic surfactant and amphiphilic carbon-iron complexes; 3) adsorption bridging between the surfactant oil droplets and polysilicate. The results of comparative tests in treating the actual cold-rolling emulsion wastewater showed the MBFD could bring about significant technical, economic benefits and achieve utilization of metallurgical solid wastes.
Implications: Blast furnace dust (BFD) is an industrial solid waste obtained by dry de-dusting from blast furnace gas during the blast furnace ironmaking process. The main components of BFD are iron oxide and carbon, and also contain small amounts of different recoverable non-ferrous metals zinc, secret, indium and lead, which have recovery value. In this study, we enhanced the three parts of BFD and used to demulsification. In this study, the BFD was modified in three aspects (hydrophobic functional groups, positively charged iron oxide, and polysilicon) to achieve the secondary utilization of waste in emulsion wastewater treatment. And investigated the effect of reaction conditions on the demulsification effect with modified blast furnace dust (MBFD). Furthermore, the GC-MS analyzes combined with kinetics interpret the demulsification mechanism with the MBFD as a secondary resource. Finally, the comparative tests in treating the actual cold-rolling emulsion wastewater showed the MBFD could bring about significant technical and economic benefits and achieve utilization of metallurgical solid wastes.
Introduction
During the cold rolling process of steel production, the emulsion is critically required for cooling and lubrication to eliminate the hot deformation, which can prolong the service life of products and improve the quality of processing (Zhang et al. Citation2010).
In recent years, the composition of emulsion has become more and more complicated with the rapid development of industrialization, which makes it extremely difficult to degrade. The emulsion is mainly composed of 2%-10% organic phase and 90%-98% aqueous phase (Ma et al. Citation2018). Among them, the organic phase contains not only mineral oil and vegetable oil but also various additives such as anionic surfactants, nonionic surfactants, preservatives, compressive agents, bactericides, antifoaming agents, etc. (Barreto et al. Citation2011). However, the addition of various additives increased the stability of the emulsion while increasing the toxicity. Mitjavila et al. found that surfactants altered the permeability of mouse intestinal cell membranes and immobilized certain proteins to inhibit the activity of specific enzyme proteins (Mitjavila et al. Citation1975). In addition, the petroleum hydrocarbons, phenols, and polycyclic aromatic hydrocarbons contained in oily wastewater slow down the growth of plants and animals while increasing the risk of cancer in humans (Ismail et al. Citation2019). The emulsion wastewater floating on the surface of rivers, lakes, and oceans formed an oil film that blocked the sun’s rays and prevented oxygen from dissolving into the water, leading to the death of aquatic organisms and seriously polluting the local water environment (Abdullah-Al-Wadud et al. Citation2007; Hanafy and Nabih Citation2007).
Having witnessed severe environmental pollution from emulsion wastewater led to increasingly restrictive regulations for the disposal of metalworking fluids (Cheng, Phipps, and Alkhaddar Citation2005). The Water Pollutant Discharge Standard for the Iron and Steel Industry in China (GB 13456–2012) requires that the total discharge outlet wastewater CODcr emission limited in the cold rolling enterprises is 50 mg·L−1, which pose a huge challenge for the treatment of high-concentration refractory wastewater in the steel industry. Biological processes such as activated sludge and biofilms and a variety of advanced oxidation techniques are commonly used. However, they have some notable drawbacks like high cost or/and poor treatment performance. Thence, it is of considerable interest in developing new demulsification materials with multiple decontamination functions.
Yuan et al. used a variety of natural minerals as the demulsifier, such as artificial zeolite, natural zeolite, diatomite, bentonite, natural soil. The results indicated that both metal ions and silicic acid in natural minerals promoted flocculation and agglomeration, which removed over 90% of chemical oxygen demand (COD) at lower pH and higher temperature conditions. (Yuan, Man, and Wu Citation2011). Mi et.al produced HA-coated Fe3O4MNPs using a chemical co-precipitation method, followed by wrapping with PPTA through centrifugation process. The Fe3O4@HA@PDDA NPs with positively charged revealed high-quality oil-water separation performance at lower concertation emulsions, and the excellent degradability was owing to the reduction of surface potential that improved the interface activity (Mi et al. Citation2020). Therefore, it could be concluded that metal oxides and silicates could play a central role in the oil-water separation.
Blast furnace dust (BFD) is an industrial solid waste obtained from blast furnace gas by dry dedusting in the blast furnace ironmaking process. The main components of the BFD are iron oxide and carbon, possibly containing small amounts of different recoverable non-ferrous metals such as zinc (Zn), bismuth (Bi), and lead (Pb). (Balakrishnan et al. Citation2011; Wu et al. Citation2016). However, the BFD as a solid oxide cannot be treated effectively for a long time, which inevitably leads to increasing pressure on the local environment (El-Hussiny and Meh Citation2010; Zhang et al. Citation2007).
At present, the BFD as a kind of catalyst has been applied to Fenton oxidation reaction, electrocatalytic oxidation reaction, etc. Amorim, Dutra, and Le (Citation2012) prepared a type of BFD catalyst and evaluated the degradation efficiency of azo dyes in the Photo-Fenton reaction. Although the decolorization effect of the BFD catalyst was similar to the homogeneous photo-Fenton method (using FeSO4), the BFD catalyst efficiency significantly increased (more than 5 times) according to the quasi-primary kinetic model (Amorim, Dutra, and Le Citation2012). Amorim, Leao, and Moreira (Citation2009) also found that BFD was effective for decolorization of printing and dyeing wastewater under irradiation system due to the light accelerated the Fe2+/Fe3+ cycle, producing more hydroxyl radicals for homogeneous and multiphase reactions (Amorim, Leao, and Moreira Citation2009). Moreover, Lz et al. (Citation2020) prepared a composite catalytic ceramic filter (CCF) with the BFD, and the results showed that the BFD-based Fe-Ni ceramic packing (CCF) significantly improved the ciprofloxacin (CIP) removal rate (about 42%-72% CIP removal after 3 h) compared with the conventional Fe-C ceramic packing (CF) (Lz et al. Citation2020).
In addition, due to some physicochemical features such as carbon particles with hydrophobic surfaces and iron oxides with hydrophilic surfaces, the BFD has enormous potential to separate oil/water in emulsion wastewater (Amorim, Dutra, and Le Citation2012; Ma et al. Citation2020; Teixeira et al. Citation2015). In other words, the demulsification potential of BFD is mainly manifested by the electrostatic interaction between iron oxide surface and an anionic surfactant, alongside the interaction between hydrophobic carbon surface and oil droplets. However, the ties between interactions above on the demulsification effect were hardly explored thus far.
Based on these findings and researches, current research rarely focuses on the use of the BFD in industrial wastewater fields, especially in the treatment of high-concentration metallurgical emulsion wastewater. As such, a new demulsification material with high-efficiency demulsification ability is obtained by modifying the steel waste BFD in this study. The main objectives were as follows: (1) to explore the modification indicators for improving the demulsification performance and delve into optimizing the modification systematically; (2) to investigate the influence of reaction conditions on the demulsification by modified blast furnace dust (MBFD); (3) to analyze the demulsification mechanism of MBFD through the way of GC-MS and kinetics, as well as the application feasibility in practical wastewater.
Materials and methods
Preparation of emulsion
The emulsion used in the experiment was sampled from a large-scale metallurgical enterprise in central China. According to the commonly detected oil content in emulsifying effluent, the O/W emulsion was prepared by adding 1000 mg Quaker crude oil into 1000 ml distilled water (petroleum content: 872 mg, additive content: 128 mg) and stirring for 20 min with the speed of 1200rpm ().
Table 1. Water quality comparison between actual and simulated wastewater.
Preparation of the MBFD
In order to fully realize the resources closed-loop utilization, the BFD was collected from the same metallurgical enterprise in China. Firstly, large particles of impurities mixed in the collection process were screened out with a 200-mesh screen before the modification. Subsequently, main factors (including Carbon type, ω(C), and nFe/nSi) in the modification were selected to comparatively study, and the details were as follows.
(1) Carbon type: Three different materials of highly hydrophobic carbon (A: ZHF-R90, B: GWF-R90, C: MWF-R90 commercial activated carbon material) were compounded with the BFD by the Co-heating method. Firstly, a solution of isobutyltriethoxysilane (1%) was added to the mixture, and then the mixture was put into Electric Oven at 80°C for 5 h to form a precursor (Xu, Ma, and Wang Citation2018; Zhang et al. Citation2019, Citation2020). Finally, the MBFD1 was prepared by pyrolysis.
(2) ω(C): The hydrophobic carbon (It had the best demulsification effect obtained from the first experiment of BFD modification (Figure) with various ranges of concentration was compounded with the original BFD by the Co-heating method. Afterward, the MBFD2 was obtained by pyrolysis.
(3) nFe/nSi: Since silicon was constant in the content of the BFD, therefore, Fe2O3 was added to increase the Fe content in the BFD via co-mingled grinding. Then, the mixture above was put into the reflux reaction for 3 h under the temperature of 80°C. Finally, the MBFD3 was obtained after the process of pyrolyzing, filtering, washing, and drying.
Pyrolysis: The pyrolysis was conducted in a nitrogen atmosphere tube furnace under the condition of 600°C for 3 h, with a temperature-rising rate of 10°C/min. After cooling to room temperature, the mixture was ground with a tumbler ball mill until it passed through a 200-mesh filter sieve. Subsequently, the product was washed with deionized water and dried in the oven at 60°C for 8 h, and the MBFD was obtained.
Demulsification methods
The BFD demulsification experiment
The initial pH of the wastewater was adjusted to around 5; BFD dosage: 0.8 g·L−1. The mixing procedure did not impact the results largely as long as the speed gradient was within a reasonable range. Thus, the stirring pattern2 (Table S2): fast stirring (250rpm) 1 min, medium stirring (150rpm) 5 min, slow stirring (50rpm) 8 min. After sedimentation for 100 min, CODcr and oil content were measured by taking an appropriate amount of supernatant at 3 cm below the liquid surface and setting up three parallel samples to reduce the experimental error.
The MBFD demulsification experiment
In this study, the influences of modified conditions (Hydrophobic carbon types, Carbon mass fraction, and n(Fe)/n(Si) ratio) and reaction factors (the initial pH, the MBFD dosage, and the average agitation rate (G–)) on demulsification were investigated by using the control variable method.
Exploration of modification: Hydrophobic carbon types (ZHF type, GWF-HAC-R90 type, and AC-17 M-3 type commercial activated carbon materials), Carbon mass fraction (9–19%), Fe/Si ratio (1.25–1.75) were designed to evaluate their effect on demulsification. In these experiments, 0.8 g·L−1 BFD was added to the wastewater containing emulsion (Oil:1300 mg·L−1, CODcr:8667 mg·L−1) with pH = 5 under stirring.
Demulsification experiment: The initial pH, the MBFD dosage, and the G– were selected as the main influencing factors in the MBFD demulsification experiment.
Firstly, 250 mL emulsion at different initial pH (1,2,3,4,5,6,7,8,9,10) was mixed with 0.8 g·L−1 MBFD in 500 ml beakers under stirring mode 2 (Table S2). Initial pH in all experiments was adjusted by using a 0.5 M NaOH or 0.5 M HCl solution. Secondly, 250 ml O/W solution was mixed with different MBFD doses (1,2,3,4,5,6,7,8,9,10 g · L−1) in 500 ml beakers. Based on the above experiment, different G– were also set to investigate the impact of the stirring rate on demulsification. All of these tests were conducted at room temperature and repeated three times.
Zero-point charge (ZPC) measurement experiment
Several beakers were filled with 15 mL of 4 mM NaCl solution and the pH was adjusted between 3 and 11 using 1 M NaOH or 1 M HCl with the increment of 2. Subsequently, 20 mg of samples (MBFD, MBFD2, BFD) were added to the pH adjusted beaker and kept at room temperature for 2 days to measure the post-reaction pH. Finally, the graphs were plotted with the initial pH as the independent variable and the ΔpH(initial pH – post-reaction pH) as the dependent variables (Shahana, Aarti, and Raja Citation2018). The intersection of this curve (ΔpH) with the initial pH = post-reaction pH line is called pHzpc.
Material reuse experiment
After filtering to retrieve the MBFD, it was subjected to a thermal treatment to regenerate its capacity. A horizontal tube furnace was used for heating in nitrogen to 600°C and kept for 2 h, where the N2 flow rate was 25 mL·min−1 and the heating rate was 5°C·min−1. After cooling to room temperature, the MBFD was rinsed with anhydrous ethanol and distilled water 3 times respectively. The treated MBFD was then used again for demulsification to evaluate its reusability.
Analysis method
Physics-chemical analysis method
The oil content was quantitatively determined by Infrared spectrophotometry (HJ 637–2018), and the CODCr was determined by using the Dichromate method (HJ 828–2017). The concentrations of Fe, Si, Ca, Al were measured using an Inductively Coupled Plasma (ICP) Emission Spectrometer (Optima 4300DV, PerkinElmer, USA). The pH was measured using a pH analyzer, and Zeta potential and isoelectric point (IEP) were determined by Zeta Potential Analyzer (Zeta Sizer, Malvern Instruments Corporation, England). The elemental composition of the BFD was determined by X-ray fluorescence spectrometry (XRF, Shanghai, China). The morphology features of the BFD were expressed by a Scanning electron microscope (SEM, JSM-7500 F, Japan). The elements qualitative and semi-quantitative analysis of the BFD was determined by X-ray energy spectrometer (EDS, X-Max 80, China). The specific surface area before and after the BFD modification was analyzed by Brunauer Emmett Teller (BET) test method (BET, TriStar II 3020, USA). The functional group of BFDs was determined using an infrared spectrometer (FT-IR, Nexus, USA) and the composition of the emulsion was determined by Gas chromatograph-mass spectrometer (GC-MS, Agilent 6890 N, USA). Morphological changes of oil droplets were analyzed by Microphotography System (CH30, Olympus Corporation, Japan). The particle size distribution of oil droplets was measured by a laser particle sizer (LPSA, Mastersizer 2000, China).
Mathematical analysis method
(1) Water contact angle
The water contact angle(θ) is a key point that reflects the wettability of the BFD. Specifically, the solid surface with numerous nonpolar molecules performed repulsively to water molecules, and the interaction is extremely apparent as the angle between the contacted surfaces is greater than 90°. Therefore, there is the following determination relationship, that is, θ = 90° can be used as the boundary: θ < 90° is wettable while θ > 90° is non-wettable. The water contact angle was measured by a contact angle meter (Jc2000D, China), and the water contact angle of the powdered material was further verified by the transmittance height method. Only the wetting ability of the MBFD for the same liquid (water) needed to consider in this study, thus the Relative Wetting Contact Angle was used to indicate θ. (Alam, Howlader, and Deen Citation2014).
Owing to the capillary action of the liquid infiltrating into the capillary of radius (R) in time (t), the length (h) of the capillary tube can be calculated by Washburn’s equation (Sheng, Li, and Dai Citation2001).
Where r is the surface tension of the liquid; R is the effective capillary radius of the powder column; is the viscosity of the liquid and θ is the relative water contact angle.
(2) Dynamic fitting analysis
Two kinetic fits were performed for each reaction stage separately, and the fitted one with high correlation R was used as the kinetics model of this stage.
Zero-order dynamics model:
Pseudo-level kinetic model:
Where kobs is the reaction rate constant; C0 and Ct denote the CODcr of the emulsions at reaction initial time (min) and ending time (min), respectively.
Results and discussion
Optimization of demulsification performance of the BFD
Morphology and surface property of the BFD
BFD was composed of black-gray dusty fine particles, while some hard particles may be mixed occasionally (Figure S1a). As shown in , most of the BFD particles were irregularly spherical with angular edges and the average particle size was around 10 ~ 50 μm, while the rest spherical particles with rounded corners possessed the range of particle size about 0.5 ~ 3.0 μm, which not only increased the specific surface area but also improved the oil capture performance of the material (Boglaienko and Tansel Citation2017). The main elements of the BFD content of Fe, C, O, Si, and so on can be seen through EDS analysis of the spectrum (Chen et al. Citation2017; Zhao, Li, and Ruan Citation2021). In , XRD spectrum analysis explored the phase characteristics of elements in the BFD. Through the analysis of Jade V6.0 software, it could be found that the angle of 33.17°, 35.63°, 40.87°, 49.48°, 54.10°, 62.46°, 64.05° represented Fe2O3 while 24.15°, 25.88°, 26.05° represented SiO2 (Ahn and Yun Citation2019). Combined with these analyses, the main composition of the BFD particles might be carbon particles, ferric oxide particles, and silica particles.
Figure 1. SEM images of the BFD at (A) 200x, (B) 400x, and (C) 10,000x magnification and corresponding spectra obtained by EDS.
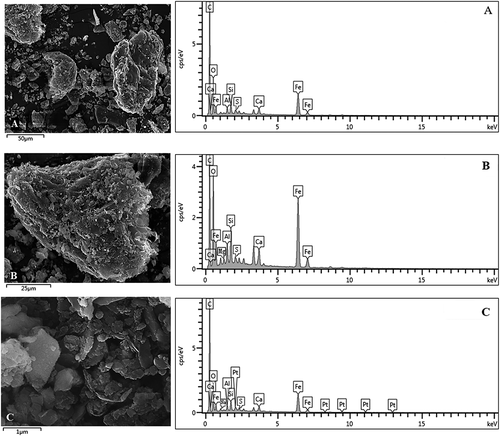
Further analysis results of CHNS/O elemental analyzer and XRF, the mass fraction of each component was calculated. gave the qualitative and semi-quantitative analysis result of the BFD before modification. It was found that the elements Fe, Si, C had relatively high content in the BFD, which played a leading role in demulsification (Bernat et al. Citation2010; Elmobarak and Almomani Citation2021; Wang, Feng, and Xu Citation2010). Among them, Ferric oxide was used extensively in oil-water separation due to its electrostatic interaction and strong interfacial activity (Mi et al. Citation2020; Soares et al. Citation2019). The combination of hydrophilic silicon and aluminum oxide surfaces with hydrophobic carbon nanostructures produced amphiphilic materials, which had a significant impact on the interaction and separation of the two-phase system (Teixeira et al. Citation2012). Therefore, the BFD was modified by exploring the elemental content variation to optimize the demulsification performance.
Modification of the BFD
The effect of adding different strong hydrophobic carbons (A: ZHF-R90, B: GWF-R90, and C: MWF-R90) on the CODcr and oil removal efficiency, and water contact angle of the MBFD1; Effect of the carbon content of the MBFD2(b), hydrophobic carbon (GWF-R90) dosage (c), and n(Fe)/n(Si) of the MBFD3 (d) on CODcr and oil removal efficiency; (b1) Comparison between the use of MBFD2 (ω(c):14.86%) and hydrophobic carbon (GWF-R90) on CODcr and oil removal efficiency; (e) Demulsification of the MBFD before and after modification; (Experimental conditions for (b1) and (c): hydrophobic carbon (GWF-R90) and MBFD2 (ω(c):14.86%): 0.8 g·L−1, pH:5, the stirring pattern 2)
Figure 3. (a) The effect of adding different strong hydrophobic carbons (A: ZHF-R90, B: GWF-R90, and C: MWF-R90) on the CODcr and oil removal efficiency, and water contact angle of the MBFD1; Effect of the carbon content of the MBFD2(b), hydrophobic carbon (GWF-R90) dosage (c), and n(Fe)/n(Si) of the MBFD3 (d) on CODcr and oil removal efficiency; (b1) Comparison between the use of MBFD2 (ω(c):14.86%) and hydrophobic carbon (GWF-R90) on CODcr and oil removal efficiency; (e) Demulsification of the MBFD before and after modification; (Experimental conditions for (b1) and (c): hydrophobic carbon (GWF-R90) and MBFD2 (ω(c):14.86%): 0.8g·L−1, pH:5, the stirring pattern 2)
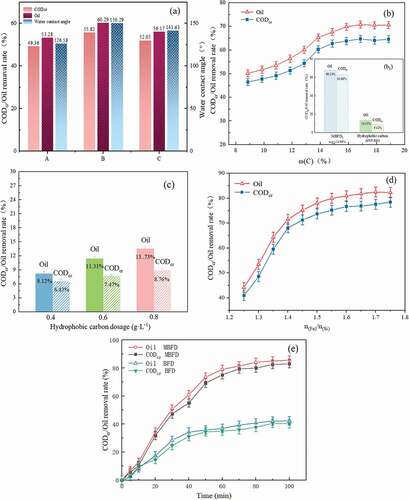
The water contact angles of these three MBFD1s and the pristine BFD were tested separately by contact angle meter and the transmittance height method to investigate the effect of different hydrophobic materials on the hydrophobicity of the MBFD1 surface (Figure S1b). Among them, the water contact angle of the MBFD1(B) reached 150.29°, approaching superhydrophobic material (Jeevahan, Chandrasekaran, and Joseph Citation2018). As illustrated in ), it was obvious that the CODcr and oil removal efficiency of sample B reached 55.82% and 60.29%, respectively. Meanwhile, the water contact angle of sample B increased from 112.63 ° to 150.29 °, indicating that the increased hydrophobicity of the carbon material enhanced the demulsification effect of MBFD1 (Xiong, Wu, and Xue Citation2012).
After identifying the carbon type as GWF-R90 (average pore size: 3 ~ 5 nm, water contact angle: >140°), the effect of demulsification was researched by changing the content of carbon in the BFD (MBFD2). Current research suggested that the combination of oxides with hydrophobic carbon structures generates amphiphilic structures, which enhanced the oil-water emulsion separation properties of materials (Jayalakshmi and Balasubramanian Citation2009; Oliveira et al. Citation2010; Teixeira et al. Citation2012). In 1, the MBFD2 had an efficient demulsification efficiency compared to a single hydrophobic carbon. It could be inferred that the iron oxide (silicon) and hydrophobic carbon were also gradually formed amphiphilic combinations to facilitate the demulsification process during the first stage (ω(C): 9–14.86%) in ) (Yang et al. Citation2018). As the content of carbon was approximately14.86%, the removal rate of CODcr and oil was almost 64.71% and 69.89%, respectively, suggesting that the addition of hydrophobic carbon promoted oil-water separation. However, in , the increase of hydrophobic carbon content in the solution had little effect on the demulsification effect as well as the removal of oil and CODcr was less than 20% at 0.8 g·L−1 dosage. It provided evidence that hydrophobic carbon did not play a single role on the MBFD2 surface and also inferred that the excessive addition of hydrophobic carbon did not improve the demulsification effect on the premise of the limited number of oxides on the MBFD2 surface. In addition, we observed that carbon powder fell off the surface of MBFD2 with the amount of carbon higher than 15%, indicating that the amount of hydrophobic carbon on the surface of the MBFD2 was over-sufficient, which made the adsorption capacity reach saturated and the demulsification effect was all but invariant.
) showed a comparison between the proportion of n(Fe)/n(Si) in the BFD on demulsification. The test was carried out with the modified carbon type that contained 14.86% C content (GWF-HAC-R90). Previous researches reported that the coagulation mechanisms of Ferric oxide particles and polysilicate were electrical neutralization, compression of double electric layers, and bridging, respectively (Shen, Liaosha, and Xingrong Citation2014; Yu, Yang, and Graham Citation2016). The O/W emulsion system was mainly composed of negatively charged surfactants, therefore, the coagulation mechanism of Ferric oxide particles with positive charge played a primary role in demulsification. In the first stage (n(Fe)/n(Si):1.25–1.4), the electrical neutralization effect was strengthened step by step along with the rise of Fe content. In the second stage (n(Fe)/n(Si):1.4–1.55), the slow increment of the demulsification effect could be attributed to the continued bridging effect of polysilicate based on the gradual peaking of the electrostatic effect. With the further increase of iron silicon ratio, the demulsification effect remained basically constant, and the oil removal rate and COD removal rate were maintained at about 83.2% and 77.6%, respectively. Besides, increasing the molar ratio made the MBFD3 unworthy in consideration of economy, thus 1.55 was determined as the optimum ratio of n(Fe)/n(Si).
Characterization of the MBFD
As shown in , the surface of the MBFD showed a significant increase in small spherical particles (0.5–3.0 μm) and fragment irregular particles (10–50 μm) compared with the BFD (). Combined with the XRF analysis results after modification (), they could be identified as iron oxide, carbon, and silica, whose mass fraction increased by 9.35%, 4.39%, and −2.02% respectively. These results suggest that the surface of the MBFD was mainly composed of grafted spherical hydrophilicity iron oxide and hydrophobic carbon fragments. In addition, the combination of hydrophobic carbon and hydrophilic iron oxide produced an amphiphilic structure, which had a remarkable effect on the separation of two phases system, e. g. oil, and water. And it should be noted that the Si content in the MBFD didn’t change remarkably although the Si mass fraction had decreased, which was demonstrated by the fact that the Si content of MBFD determined by ICP remained almost unchanged.
Table 2. XRF analysis results of elements of the BFD before and after modified.
Figure 4. SEM images for the MBFD (a) 400x, (b) 10,000x; SEM images of the MBFD1(c)1000x, the MBFD2(d)1000x and the MBFD3(e)10000x under the optimum modification condition.
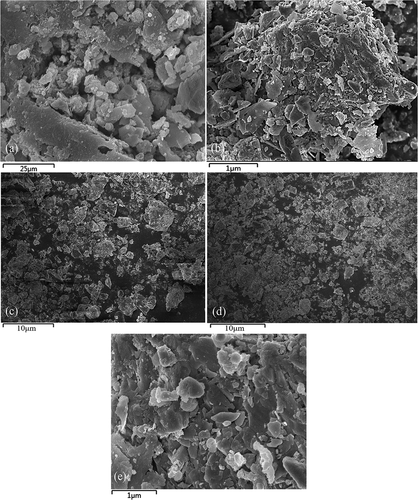
The presence of hydrophobic carbon and silica was further corroborated confirmed by FTIR spectra. As shown in , it could be seen that the absorption peaks at 1570 cm−1 and 1200 cm−1 were attributed to the C = O stretching and C-O stretching vibrations, respectively (Zhang, Hu, and Sun Citation2018). The absorption peak at approximately 1095 cm−1 in the curve could be assigned to the Si-O-Si vibration of the BFD, the characteristic functional groups of SiO2 (Liu, Zeng, and Sun Citation2020). Furthermore, the FTIR spectrum of the curve included adsorption bands at around 500 cm−1,1620 cm−1 referring to the deformation vibration of C-N-O, C = C stretching (Liu et al. Citation2010). Notably, the absorption peak less than 500 cm−1was attributed to C-C-C which had a deformation vibration (Du and Liang Citation2001). After modification, the strength of peaks at 2900 cm−1 and 2850 cm−1 were appeared, and was associated with -CH2- stretching mode (Marikhin et al. Citation2017). The signal at 722 cm−1 belonging to -(CH2) n- was obviously enhanced (Seham et al. Citation2017). These results further confirmed that the content of hydrophobic carbon on the surface of BFD modified significantly increased.
Figure 5. Fourier infrared absorption spectra of the BFD before(a) and after(b) modified; (c) MBFD after one cycle.
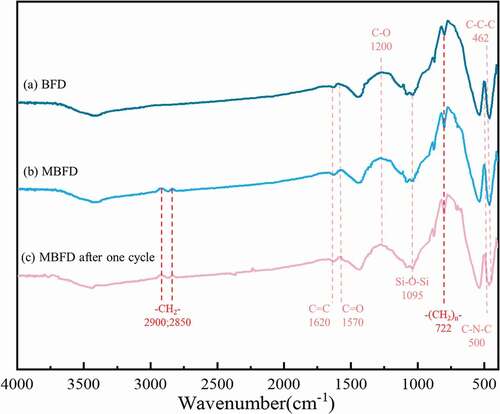
In addition, as shown in , the enhancement of the demulsification effect was revealed by some quantitative analysis, including Water contact angle, Zeta potential (pH:5), Specific surface area. The water contact angle (WCA:112.6°) for the BFD was much smaller than that in the MBFD (WCA:145.5°) mainly due to the increase of hydrophobic carbon contents on the MBFD surface. Specifically, although the wrapping of iron oxide (after composite modification) slightly reduced the water contact angle compared to that of the single hydrophobic carbon modification, the MBFD still possessed high hydrophobicity (WCA:145.5°) with a larger specific surface area (4.8 m2·g−1). The structure with a high specific surface area and appropriate hydrophobicity endowed the material with excellent adsorption performance, which reduced the diffusion resistance during the adsorption of organic pollutants. Moreover, as can be seen in ), the wrapping of iron oxide also increased the pHzpc of the MBFD. For electrostatic-dominated reactions, it not only improved the attraction to negative charges but also widened the pH range of effective demulsification to enhance the efficiency of the MBFD in removing anionic surfactant oil droplets. In summary, the demulsification effect was strengthened during the modification of adding the ferric oxide and carbon particles content on the BFD. (Amorim, Dutra, and Le Citation2012). Furthermore, it was clear that the demulsification effect of the MBFD had increased intuitively in .
Table 3. Water contact angle, Zeta potential (pH:5) and Specific surface area results of the BFD before and after modified.
Effect of reaction parameters on demulsification
pH
It had been reported that pH was one of the main factors affecting demulsification performance (Su et al. Citation2017). Thus, the removal was performed in the pH range of 1.0–10.0 to evaluate the effect of pH on the demulsification performance. As illustrated in ), in the pH range of 1.0‒5.0, CODcr and oil removal efficiency increased gradually first and then decreased with increasing pH. When pH increased from 5 to 6, the demulsification effect increased slightly. Subsequently, the demulsification decreased sharply with the increase of pH. The phenomena above could be explained as follows.
Firstly, at lower pH (1–3), the solution pH was less than the pHzpc ()) of MBFD indicating that the surface sites of iron oxide were protonated and positively charged (Kulkarni et al. Citation2016). In this way, the MBFD was more prone to attract oil droplets with anionic surfactants and formed larger oil aggregates on the surface (Choi et al. Citation2015). Meanwhile, a variety of hydrophobic organic groups on the hydrophobic carbon surface bonded with a small number of surfactant components such as nonpolar hydrocarbon chains, thus promoting the oil droplets to collide with each other (Bakhshi and Tavakol Citation2020; Kayvani et al. Citation2016). It was noteworthy that the demulsification effect of the MBFD increased during the pH 1–3, probably attributed to the hydrolysis products of iron ions promoting the demulsification effect. At pH bellowed 1, the Fe3+ existed mainly in the ionic state with relatively low coagulation capacity. As pH increased (1–3), the Fe3+ gradually bridged with hydroxyl groups to form polynuclear hydroxyl complexes, such as FeOH2+, Fe (OH)2+, etc. These complexes could not only reduce the ξ-potential of emulsions effectively but also aggregate emulsions by electrostatic action and adsorption bridging. Therefore, the demulsification effect would increase as the pH increased from 1 to 3 (Campbell, Leong, and Berndt Citation2006; Zhu Citation1993). All in all, the removal efficiency of CODcr and oil increased markedly at lower pH (1–3).
With the increase of pH from 3.0 to 6.0, the decline of the demulsification could be related to the weak electrostatic interaction due to the decrease in net charge density on the surface of iron oxide particles (Souza, Licea, and Colao Citation2021). Although the polymerization of silicic acid monomers could spatially form polysilicate with chain, ring, and mesh-like three-dimensional structures to trap the oil droplets, however, the effect above could not fully make up for the decrease in the removal performance brought by the weakening electrostatic action since the content of Si had only accounted for a relatively smaller portion in the MBFD (Song et al. Citation2012).
When pH continued to rise to 7 or above, the demulsification effect of the MBFD kept a pretty low level. The reasons could be stated as follows: firstly, the silicic acid was barely hydrolyzed under alkaline conditions and lost its ability to break emulsions (Choppin, Pathak, and Thakur Citation2008). Secondly, the pH of the solution was higher than pHzpc (6.74) of MBFD, the ferric oxide of MBFD was gradually deprotonated, and the number of negatively charged sites increased, leading to an increase in electrostatic repulsion between oil droplets with negatively charged and the MBFD. (Demangeat, Pedrot, and Dia Citation2018; Ridder and Halem Citation2018). All these reasons made the demulsification efficiency in MBFD hit the trough. Nevertheless, it was reassuring that the negatively charged iron oxide interacted electrostatically with a small number of oil droplets with non-charged and the hydrophobic organic groups could still have an association with the nonionic surfactant in a hydrophobic manner.
The MBFD dosage
). presented the dosage of MBFD (0–1 g·L−1) on the demulsification effect. The oil removal efficiency was gradually enhanced with the increasing dosage and began to decrease when the dosage was over 0.6 g·L−1. In ), at the MBFD dosage of 0.1–0.4 g·L−1, the anionic surfactant in the solution was electrically neutralized with ferric oxide, which made the zeta potential on the sliding surface of oil droplets decrease. And the oil removal efficiency reached a maximum of 91.92% at the MBFD of 0.6 g·L−1. However, the favorable situation was gradually suppressed with the increasing dosage, which could be explained from the perspective of zeta potential (Deng et al. Citation2005; Elmobarak and Almomani Citation2020). As illustrated in ), the initial value of the emulsion zeta potential was −47.2 mV. With the increase of the MBFD dosage, the zeta potential of the emulsion wastewater system increased significantly. The positively charged particles met the oil droplets with anionic surfactant by the collision, which made the Zeta potential drop, and the oil droplets gradually aggregated. When the MBFD dosage exceeded 0.7 g·L−1, the negatively charged oil beads showed an apparent sign of charge reversal, making the oil droplet particles in the water re-stable through electrostatic force.
The average G
Considering the inter-particle collisions in the liquid phase during demulsification, the average agitation rate (G–) was chosen as one of the crucial factors to explore the effect of demulsification.
The orthokinetic flocculation process is that the particles collide and agglomerate through mechanical or hydraulic stirring, in which the stirring speed gradient G and flocculation time T are intimately related to the flocculation effect (Nandy et al. Citation2003; Zhu Citation2014). Therefore, the G– associated with G and T was used as control indicators in the flocculation stage (Table S2). As shown in ), the settling performance was improved and the CODcr removal rate reached 82.03% at the G– about 36S−1. As G– increased further, the turbulence of the water flow increased. And flocs were broken apart under intense action of water flow shear, which led to a decrease in settling ability and CODcr remove rate.
Components analysis
Firstly, the main components of the emulsion were analyzed by GC-MS. As shown in Figure S2 and Table S3, the emulsion contained some organic additives, such as 1-chloroparaffin, halogenated hydrocarbons, and cycloalkanes that had anti-oxidation, anti-foaming, extreme pressure, anti-wear properties. Moreover, the main surfactant components in this crude oil were anionic surfactants such as sodium dodecyl sulfate, sodium butyl naphthalene sulfonate, etc., and fluorinated ester polymeric nonionic surfactants such as eicosyl pentafluoropropionate, nonyl heptafluorobutyrate, tetramethyl pentafluoro propionate, etc.
The obtained water samples from supernatants after demulsification were further analyzed by GC-MS. As shown in table S4, the matter mineral oil, anionic, and nonionic surfactant components were significantly decreased, especially the species of large-molecule high-carbon alkanes and anionic surfactants, indicating a decline in organic species concentration. In the light of studies above in ), the electronegativity (zeta potential) of emulsions decreased after the MBFD adding, which made the oil droplets mainly composed of anionic surfactants destabilized and adsorbed on the MBFD.
Demulsification mechanism of the MBFD
In the light of the studies above, the demulsification process was mainly composed of three aspects: electrostatic effect, flocculation, and hydrophobic association, among which the electrostatic effect played the leading role. The demulsification mechanism of the MBFD could be summarized as follows.
Firstly, the surface of MBFD was positively charged due to the protonation of ferric oxide surface sites under acidic conditions, resulting in electrostatic effects on anionic and nonionic surfactants on the surface of oil droplets. The adsorption between the oil droplets with anionic surfactants and the positively charged ferric oxide surface sites reduced the emulsion potential through electrical neutralization, which ultimately realized the coalescence of oil droplets. Otherwise, according to DLVO theory, when the repulsive energy peak (Emax) was low enough for the oil droplets to collide and accumulate in Brownian motion, the oil droplets could aggregate and then settle together with MBFD or float (Miyagawa et al. Citation2015).
Secondly, an amphiphilic complex system could be formed by a combination of the hydrophobic carbon chain groups with iron oxides hydrophilic active sites on the surface of MBFD, which had an excellent removal efficiency of oil droplets with nonionic surfactants (Israelachvili and Pashley Citation1982; Yong, Liu, and Miao Citation2019). showed a simplified scheme of these processes, where the nonpolar region (hydrophobic pocket) on the MBFD surface was surrounded by oil/water molecules. When the amphiphilic complex met the oil droplet, water molecules were drained from the region and accumulated in the polar region, providing an opportunity for the oil of binding to the MBFD alone and achieving microscopic o/w separation. In the meantime, the amphiphilic complex maintained a hydrophobic association with oil droplets by reducing free energy. Furthermore, the positively charged iron oxide played a synergistic role: the nonionic surfactants oil droplets around MBFD were polarized and approached MBFD slowly by electric field forces. At last, they could bond with the amphiphilic complex system.
Thirdly, under the acidic conditions, the SiO2 in MBFD would be hydrolyzed into three forms: H5SiO4+, H4SiO4, and H3SiO4− (table S1). In the emulsion, the silicates (H4SiO4, H3SiO4−) with coordination sites were bonded to surfactant to form organic-inorganic(surfactant-polysilicate) ion pairs and then arranged in a hexagonal stacking pattern with liquid crystal structures, contributing to form some active compounds with rod-like micelle structures (Aleisha et al. Citation2020; Huo et al. Citation1995). Subsequently, the floc structure of “oil droplet-(surfactant-polysilicate)-oil droplet” was generated by hydrogen bonding between polar heads of polysilicate and surfactants (Pevzner, Regev, and Lind Citation2003), which played an adsorption-bridging role for interconnection between oil droplets. Although hydrogen bonding was a relatively weak intermolecular force, it could still be applied as an auxiliary means to assist electrostatic interaction and hydrophobic association in demulsification.
Dynamics analysis
In order to explore the demulsification process, as shown in , the first-order reaction kinetics model was employed, and Figure S3 exhibited that the demulsification process of MBFD was divided into three stages.
Table 4. Three-stage kinetic fit results.
The demulsification effect was mainly attributed to the interaction of MBFD with the surfactants in the emulsion, which led to the emulsion instability and promoted the oil droplets to agglomerate readily. However, in stage 1, the actual reaction rate was sluggish. It was owing to that the MBFD was not mixed evenly with emulsion, which likely led to insufficient protonation of iron oxide particles on the MBFD surface and the electrostatic effect occurred locally on the MBFD only.
With a quick stir in stage 2, the reactants were mixed thoroughly. The reaction speed increased rapidly and reached the peak at 200 mg·L−1 · min (). At this point, the iron oxide particles fully were protonated with a positive charge, and the electrostatic effects began to play a dominant role in emulsions rich in anionic surfactants. As shown in Figure S4(a), there was a batch of dense “oil particles” with crystallized centers (MBFD-Oil Droplet conjugates) in the emulsion at 10 min, and the average particle size was also rising. Meanwhile, polysilicate could capture small oil droplets by adsorption bridging and the amphiphilic iron-carbon complex performed well in the process of oil-water separation on the MBFD surface, which also promoted the oil removal in the emulsion.
As the demulsification reaction proceeded, the concentration of oil in the effluent declined gradually and decreased to the lowest point. Figure S4(a) showed that the larger oil clusters centered on the MBFD and a small number of the tiny transparent oil droplets were floating around them at 20 min, which directly led to the weakening or disappearance of the electrostatic effect that dominated the demulsification at the MBFD surface. In stage 3, although the reaction rate dropped rapidly to 54.19 mg·L−1 · min, the particle size of most oil droplets in 40 min, and 80 min have reached 114.902 and 208.985 μm respectively (Figure S4(b)), which meant these oil droplets had potential to float on the water (Aleem et al. Citation2020). After 100 min, it was observed that a handful of oil droplets floated on the surface and most of the MBFD complex with oil droplets settled down at the bottom. Meanwhile, the CODcr and Oil removal rates essentially ceased to change, indicating that the demulsification reaction had been approaching its total capacity.
Reusability and practical applications
The above results proved that the MBFD had a great capacity to demulsify on cold rolling emulsions, which contained anionic surfactant types. And the MBFD had a superior demulsification effect compared to other materials with favorable adsorption properties (table S5). As shown in ), the surface functional groups of the MBFD were essentially invariant before and after the reaction. After thermal MBFD, it was possible to observe condensed oil droplets against the exhaust wall, which had similar properties to crude oil and the potential for recovery. Meanwhile, the MBFD exhibited excellent reusability with 84.58% oil removal and 80.49% CODcr removal after three reuse cycles. In addition, the number of dissolved metals remaining in the solution after demulsification was almost negligible (Table S6). Subsequently, the effect of MBFD on the actual emulsion wastewater was analyzed. In , different CODCr concentrations (mg·L−1) in the raw water, i.e., 6181.52, 8017.40, 8186.28, 8437.09, and 7750.93 were tested in the demulsification process, and the results indicated that the demulsification effect remained essentially stable at different inflow loads. In addition, in , 84.45% of CODcr could be removed at the MBFD dosage of 1.2 g·L−1, it was clear that the MBFD could improve the demulsification greatly through the way of facilitating the subsequent treatment processes. If the MBFD lost the capability of demulsification after a long term of operation, it could be separated into iron and carbon concentrates, etc. by the beneficiation process and reused as sintering raw material for iron-making. Therefore, complete waste reclamation and utilization cycle could be realized.
Table 5. CODcr removal efficiency of modified the BFD different dosage.
Table 6. Water quality comparison of five batches of wastewater; Experimental condition: MBFD: 1.2 g·L−1, pH: 5, G–:36 S−1.
Conclusion
The BFD was modified under three conditions: the carbon type of GWF-HAC-R90, the carbon content of 14.86%, and n(Fe)/n(Si) of 1.55, CODCr and oil removal efficiency reached 75.21% and 81.23% under the optimum modification, respectively. The results suggested that the MBFD could realize the efficient demulsification and oil-water separation for cold rolling wastewater. Otherwise, the demulsification was strongly affected by pH, the MBFD dosage, and the G–. Under the conditions of the initial pH of 3, the MBFD dosage amount of 0.6 g·L−1, the G– of 36S−1, the removal capability of CODcr and oil reached 82.03% and 84.59%, respectively. The experimental results and GC-MS analysis uncovered that the changes of chemical components in the emulsion were mainly reflected in hydrocarbon mineral oil, anionic and nonionic surfactants. The demulsification mechanism analysis of the MBFD in the oil removal process of cold rolling emulsion wastewater suggested that there was a synergic effect of three processes: the electrically neutralizing effect between the positively charged MBFD particles and the oil droplets with anionic surfactants; the hydrophobic association of nonionic surfactants with hydrophobic carbon-iron oxide amphiphilic structures; followed by bonding interactions between polysilicate and polar heads of surfactants. Specifically, the electrostatic effect was dominant, supplemented by hydrophobic association and adsorption bridging. In conclusion, this study provided a promising strategy for realizing efficient demulsification to promote efficient pollutants removal from emulsion wastewater.
Supplementary_file.docx
Download MS Word (1.4 MB)Data availability statement
Data available from the corresponding author on request.
Funding
This work was supported by Hainan Province Science and Technology Special Fund (ZDKJ2021024).
Disclosure statement
No potential conflict of interest was reported by the author(s).
Supplementary material
Supplemental data for this paper can be accessed on the publisher’s website
Additional information
Notes on contributors
Yibo Zhang
Yibo Zhang is a master candidate.
Meng Li
Meng Li is a professor, water pollution control and sponge City.
Wansong Huang
Wansong Huang is a senior engineer.
Kun Fan
Kun Fan is a master.
Jiawei Li
Jiawei Li is a master candidate.
Min Zhong
Min Zhong is a master candidate.
Zefeng Li
Zefeng Li is a master candidate.
Chengwei Li
Chengwei Li is a master candidate.
Qian Zhang
Qian Zhang is an associate professor, Water pollution control.
References
- Abdullah-Al-Wadud, M., M. H. Kabir, M. Dewan, O. Chae. 2007. A dynamic histogram equalization for image contrast enhancement. 25th IEEE International Conference on Consumer Electronics 53 (2):593–600. doi:https://doi.org/10.1109/TCE.2007.381734.
- Ahn, Y. Y., and E. T. Yun. 2019. Heterogeneous metals and metal-free carbon materials for oxidative degradation through persulfate activation: A review of heterogeneous catalytic activation of persulfate related to oxidation mechanism. Korean Journal of Chemical Engineering 36 (11):1767–79.
- Alam, A. U., M. Howlader, and M. J. Deen. 2014. The effects of oxygen plasma and humidity on surface roughness, water contact angle and hardness of silicon, silicon dioxide and glass. Journal of Micromechanics & Microengineering 24 (3):035010. doi:https://doi.org/10.1088/0960-1317/24/3/035010.
- Aleem, W., N. Mellon, J. A. Khan, H. H. Al-Kayiem. 2020. Experimental investigation and mathematical modeling of oil/water emulsion separation effectiveness containing alkali-surfactant-polymer. J Dispers Sci Technol 42 (9):1286–98. doi:https://doi.org/10.1080/01932691.2020.1738244.
- Aleisha, M. L., S. Kulbir, M. A. Michael, D. G. Marangoni, S. Shortall, S. D. Wettig. 2020. M-s-m cationic gemini and zwitterionic surfactants – A thermodynamic analysis of their mixed micelle formation. RSC Adv 10 (6):3221–32. doi:https://doi.org/10.1039/C9RA09432F.
- Amorim, C., M. Leao, and R. Moreira. 2009. Comparison of various advanced oxidation processes for azo dye degradation. Engenharia Sanitaria E Ambiental 14 (4):543–50. doi:https://doi.org/10.1590/S1413-41522009000400014.
- Amorim, C. C., P. R. Dutra, O. M. Le. 2012. Controlled reduction of steel waste to produce active iron phases for environmental applications. Chemical Engineering Journal 209:645–51.
- Bakhshi, P., and H. Tavakol. 2020. Oil removal from water using highly hydrophobic, ultralight carbon microsphere. International Journal of Environmental Science and Technology 17 (3):1649–56. doi:https://doi.org/10.1007/s13762-019-02495-0.
- Balakrishnan, M., V. S. Batra, J. Hargreaves, I. D. Pulford. 2011. Waste materials – catalytic opportunities: An overview of the application of large-scale waste materials as resources for catalytic applications. Green Chem. 13 (1):16–24. doi:https://doi.org/10.1039/C0GC00685H.
- Barreto, A., V. R. Santiago, S. E. Mazzetto, J. C. Denardin, R. Lavín, G. Mele, M. E. N. P. Ribeiro, I. G. P. Vieira, T. Gonçalves, N. M. P. S. Ricardo. 2011. Magnetic nanoparticles for a new drug delivery system to control quercetin releasing for cancer chemotherapy. Journal of Nanoparticle Research 13 (12):6545–53. doi:https://doi.org/10.1007/s11051-011-0559-9.
- Bernat, X., E. Piacentini, F. Bazzarelli, C. Bengoa, A. Fabregat, E. Drioli, J. Font, L. Giorno. 2010. Ferrous ion effects on the stability and properties of oil-in-water emulsions formulated by membrane emulsification. Ind Eng Chem Res 49 (8):3818–29. doi:https://doi.org/10.1021/ie901491k.
- Boglaienko, D., and B. Tansel. 2017. Wicking of light hydrophobic liquid phase from water by pulverized rubber: Theoretical and experimental analyses. J. Hazard. Mater. 325 (5):189–97. doi:https://doi.org/10.1016/j.jhazmat.2016.11.076.
- Campbell, G. R., Y. K. Leong, C. C. Berndt. 2006. Ammonium phosphate slurry rheology and particle properties—The influence of Fe (III) and Al (III) impurities, solid concentration and degree of neutralization. Chem Eng Sci 61 (17):5856–66.
- Chen, F., J. Gao, B. Qi, D. Shen. 2017. Deterioration mechanism of plain and blended cement mortars partially exposed to sulfate attack. Constr. Build. Mater. 154:849–56. doi:https://doi.org/10.1016/j.conbuildmat.2017.08.017.
- Cheng, C., D. Phipps, and R. M. Alkhaddar. 2005. Treatment of spent metalworking fluids. Water Res. 39 (17):4051–63. doi:https://doi.org/10.1016/j.watres.2005.07.012.
- Choi, Y.-W., H. Lee, Y. Song, D. Sohn. 2015. Colloidal stability of iron oxide nanoparticles with multivalent polymer surfactants. J. Colloid Interface Sci. 443:8–12. doi:https://doi.org/10.1016/j.jcis.2014.11.068.
- Choppin, G. R., P. Pathak, and P. Thakur. 2008. Polymerization and complexation behavior of silicic acid: A review. Main Group Metal Chemistry 31 (1–2). doi:https://doi.org/10.1515/MGMC.2008.31.1-2.53.
- Demangeat, E., M. Pedrot, A. Dia. 2018. Colloidal and chemical stabilities of iron oxide nanoparticles in aqueous solutions: The interplay of structural, chemical and environmental drivers. Environmental Science Nano 5:1–11.
- Deng, S., Y. Gang, Z. Jiang, R. Zhang, Y. P. Ting. 2005. Destabilization of oil droplets in produced water from ASP flooding. Colloids Surf A Physicochem Eng Asp 252 (2–3):113–19. doi:https://doi.org/10.1016/j.colsurfa.2004.09.033.
- Du, X., and Y. Liang. 2001. Molecular structure of Lead N-Octadecanoyl-l-alaninate langmuirblodgett film. Journal of Physical Chemistry B 105 (26):6092–96. doi:https://doi.org/10.1021/jp010157t.
- El-Hussiny, N. A., and S. Meh. 2010. Effect of recycling blast furnace flue dust as pellets on the sintering performance. Science of Sintering 42 (3):269–81. doi:https://doi.org/10.2298/SOS1003269E.
- Elmobarak, W. F., and F. Almomani. 2020. Application of Fe3O4 magnetite nanoparticles grafted in Silica (SiO2) for oil recovery from oil in water emulsions. Chemosphere 265:129054. doi:https://doi.org/10.1016/j.chemosphere.2020.129054.
- Elmobarak, W. F., and F. Almomani. 2021. Functionalization of silica-coated magnetic nanoparticles as powerful demulsifier to recover oil from oil-in-water emulsion. Chemosphere 279:130360. doi:https://doi.org/10.1016/j.chemosphere.2021.130360.
- Hanafy, M., and H. I. Nabih. 2007. Treatment of oily wastewater using dissolved air flotation technique. Energy Sources, Part A: Recovery, Utilization, and Environmental Effects 29 (2):143–59. doi:https://doi.org/10.1080/009083190948711.
- Huo, Q. S., R. Leon, P. M. Petroff, and G. D. Stucky. 1995. Mesostructure design with gemini surfactants: Super cage formation in three-dimensional hexagonal. Science 268 (5215):1324–27. doi:https://doi.org/10.1126/science.268.5215.1324.
- Ismail, N. H., W. Salleh, A. F. Ismail, H. Hasbullah, N. Yusof, F. Aziz, J. Jaafar. 2019. Hydrophilic polymer-based membrane for oily wastewater treatment: A review. Sep. Purif. Technol. 233:116007. doi:https://doi.org/10.1016/j.seppur.2019.116007.
- Israelachvili, J., and R. Pashley. 1982. The hydrophobic interaction is long range, decaying exponentially with distance. Nature 300 (5890):341–42.
- Jayalakshmi, M., and K. Balasubramanian. 2009. Solution combustion synthesis of Fe2O3/C, Fe2O3-SnO2/C, Fe2O3-ZnO/C composites and their electrochemical characterization in non-aqueous electrolyte for supercapacitor application. International Journal of Electrochemical Science 4 (6):878–86.
- Jeevahan, J., M. Chandrasekaran, G. B. Joseph. 2018. Superhydrophobic surfaces: A review on fundamentals, applications, and challenges. Journal of Coatings Technology & Research 15 (2):231–50.
- Kayvani, F., M. G. Ahmad, Y. Manawi, Z. Malaibari, M. A. Hussien. 2016. Outstanding adsorption performance of high aspect ratio and super-hydrophobic carbon nanotubes for oil removal. Chemosphere 164:142–55. doi:https://doi.org/10.1016/j.chemosphere.2016.08.099.
- Kulkarni, S. D., S. Kumbar, S. G. Menon, K. S. Choudhari, Santhosh C. 2016. Magnetically separable core–shell ZnFe2O4@ZnO nanoparticles for visible light photodegradation of methyl Orange. Mater Res Bull 77:70–77. doi:https://doi.org/10.1016/j.materresbull.2016.01.022.
- Liu, K., J. Zhang, G. Yang, C. Wang, -J.-J. Zhu. 2010. Direct electrochemistry and electrocatalysis of hemoglobin based on poly (diallyl dimethylammonium chloride) functionalized graphene sheets/room temperature ionic liquid composite film. Electrochem Commun 12 (3):402–05. doi:https://doi.org/10.1016/j.elecom.2010.01.004.
- Liu, Y. X., F. J. Zeng, B. L. Sun. 2020. Research on XRD and FTIR spectra of fly ash in different particle size from gujiao power plant. SPECTROSCOPY AND SPECTRAL ANALYSIS 40 (5):1452–56.
- Lz, A., G. B. Yue, A. Qy, P. Zhang, Y. Wang, B. Gao. 2020. Preparation and application of novel blast furnace dust based catalytic-ceramic-filler in electrolysis assisted catalytic micro-electrolysis system for ciprofloxacin wastewater treatment. J. Hazard. Mater. 383:121215. doi:https://doi.org/10.1016/j.jhazmat.2019.121215.
- Ma, J., J. Shi, L. Ding, H. Zhang, S. Zhou, Q. Wang, X. Fu, L. Jiang, K. Fu. 2018. Removal of emulsified oil from water using hydrophobic modified cationic polyacrylamide flocculants synthesized from low-pressure UV initiation. Sep. Purif. Technol. 197:407–17. doi:https://doi.org/10.1016/j.seppur.2018.01.036.
- Ma, J., W. Xia, R. Zhang, L. Ding, Y. Kong, H. Zhang, K. Fu. 2020. Flocculation of emulsified oily wastewater by using functional grafting modified chitosan: The effect of cationic and hydrophobic structure. J. Hazard. Mater. 403:123690. doi:https://doi.org/10.1016/j.jhazmat.2020.123690.
- Marikhin, V. A., L. P. Myasnikova, E. I. Radovanova, B. Z. Volchek, D. A. Medvedeva. 2017. Fourier transform infrared spectroscopic study of the kinetics of a first-order phase transition in tridecanoic acid CH3(CH2)11COOH. Physics of the Solid State 59 (2):331–37. doi:https://doi.org/10.1134/S1063783417020184.
- Mi, T., Y. Cai, Q. Wang, N. Habibul, X. Ma, Z. Su, W. Wu. 2020. Synthesis of Fe3O4 nanocomposites for efficient separation of ultra-small oil droplets from hexadecane–water emulsions. RSC Adv 10 (17):10309–14. doi:https://doi.org/10.1039/D0RA01044H.
- Mitjavila, M. T., S. Mitjavila, N. Gas, R. Derache. 1975. Influence of various surface-active agents on the activity of several enzymes in the brush border of enterocytes. Toxicol. Appl. Pharmacol. 34 (1):72–82. doi:https://doi.org/10.1016/0041-008X(75)90176-3.
- Miyagawa, Y., K. Katsuki, R. Matsuno, S. Adachi. 2015. Effect of oil droplet size on activation energy for coalescence of oil droplets in an O/W emulsion. Biosci. Biotechnol. Biochem. 79 (10):1–3. doi:https://doi.org/10.1080/09168451.2015.1039482.
- Nandy, T., S. Sunita, P. P. Pathe, S. N. Kaul. 2003. Pre-treatment of currency printing ink wastewater through coagulation-flocculation process. Water Air Soil Pollut 148 (1):15–30. doi:https://doi.org/10.1023/A:1025454003863.
- Oliveira, A., I. F. Teixeira, L. P. Ribeiro, J. C. Tristão, A. Dias, R. M. Lago. 2010. Magnetic amphiphilic composites based on carbon nanotubes and nanofibers grown on an inorganic matrix: Effect on water-oil interfaces. J Braz Chem Soc 21 (12):2184– 88. doi:https://doi.org/10.1590/S0103-50532010001200004.
- Pevzner, S., O. Regev, A. Lind. 2003. Evidence for vesicle formation during the synthesis of catanionic templated monoscopically ordered silica as studied by Cryo-TEM. J. Am. Chem. Soc. 125 (3):652–53.
- Ridder, D. J., and D. Halem. 2018. Influence of particle properties on iron flocculation. Water Sci. Technol.: Water Supply 18 (5–6):1617–24.
- Seham, K. A. A., K. O. Gudrun, I. Andrei, R. N. Mozhchil. 2017. Effect of organic chain length on structure, electronic composition, lattice potential energy, and optical properties of 2D hybrid perovskites [(NH3) (CH2) n (NH3)] CuCl4, n = 2–9. Applied Physics A 123 (8):531. doi:https://doi.org/10.1007/s00339-017-1150-8.
- Shahana, B., K. Aarti, and S. Raja. 2018. Facile synthesis of magnetic iron oxide nanoparticles using inedible cynometra ramiflora fruit extract waste and their photocatalytic degradation of methylene blue dye. Mater Res Bull 97:121–27. doi:https://doi.org/10.1016/j.materresbull.2017.08.040.
- Shen, X., L. I. Liaosha, W. U. Xingrong. 2014. Polymerization behavior of silicic acid with coexisting magnesium and aluminum ions. CIESC Journal 65 (3):1104–10.
- Sheng, A. D., Q. F. Li, X. M. Dai. 2001. Measurement of wetting contact angle of power by permeating height method. Physical Testing and Chemical Analysis Patra Physical Testing 3:110–12.
- Soares, S. F., T. Fernandes, T. Trindade, A. L. Daniel-da-silva. 2019. Recent advances on magnetic biosorbents and their applications for water treatment. Environ Chem Lett 18 (1):151–64. doi:https://doi.org/10.1007/s10311-019-00931-8.
- Song, Y., J. S. Peter, J. M. Grant, B. C. C. Cowie, G. I. N. Waterhouse, J. B. Metson. 2012. The influence of surface structure on H4SiO4 oligomerization on rutile and amorphous TiO2 surfaces: An ATR-IR and synchrotron XPS study. Langmuir 28 (49):16890–99. doi:https://doi.org/10.1021/la3032623.
- Souza, A., Y. E. Licea, M. V. Colao. 2021. Green iron oxides/amino-functionalized MCM-41 composites as adsorbent for anionic azo dye: Kinetic and isotherm studies. Journal of Environmental Chemical Engineering 9 (2):105062.
- Su, X. L., S. S. Li, Z. B. Liu, P. Wang, S. Pei, J. Zhang. 2017. Design of PH-responsive ‘on-off’ emulsions using CTAB/PPA emulsifiers by simulations and experiments – sciencedirect. Colloids Surf A Physicochem Eng Asp 533:140–46. doi:https://doi.org/10.1016/j.colsurfa.2017.08.027.
- Teixeira, A., A. D. Purceno, A. S. BaRros, B. R. S. Lemos, J. D. Ardisson, W. A. A. Macedo, E. C. O. Nassor, C. C. Amorim, F. C. C. Moura, M. G. Hernández-Terrones. 2012. Amphiphilic magnetic composites based on layered vermiculite and fibrous chrysotile with carbon nanostructures: Application in catalysis. Catalysis Today 190 (1):133–43. doi:https://doi.org/10.1016/j.cattod.2012.01.042.
- Teixeira, I., F. Santos, V. Sara, I. F. Teixeira, M. M. D. Leão, R. M. Lago. 2015. Efficient demulsification of wastewater by steel furnace dust. with amphiphilic and surface charge properties. Chemical Engineering Journal 271:281–86. doi:https://doi.org/10.1016/j.cej.2015.02.083.
- Wang, R., Y. Feng, H. Xu. 2010. Effective demulsification for oil–water separation through metal–organic frameworks with an amphipathic micro-domain. Materials Chemistry Frontiers 10 (4):3086–93.
- Wu, Z. J., L. C. Wang, Z. F. Gao, W.-M. Liu, X.-R. Wu. 2016. Recycling blast furnace dust into metals (Al, Zn and Ti)-doped hematite with enhanced photocatalytic activity. Journal of Environmental Chemical Engineering 4 (1):341–45. doi:https://doi.org/10.1016/j.jece.2015.11.030.
- Xiong, J., X. D. Wu, and Q. J. Xue. 2012. Surface hydrophobic modification of activated carbon. (eds) Proceedings of the Ninth National Surface Engineering Conference and the fourth national youth Surface Engineering Forum, Ningbo, Zhejiang, China, May, pp. 646–50.
- Xu, S., Q. Ma, and J. Wang. 2018. Combined effect of isobutyltriethoxysilane and silica fume on the performance of natural hydraulic lime-based mortars. Constr. Build. Mater. 162:181–91.
- Yang, F., S. Zhang, H. Li, S. Li, K. Cheng, J.-S. Li, D. C. W. Tsang. 2018. Corn straw-derived biochar impregnated with α-FeOOH nanorods for highly effective copper removal. Chemical Engineering Journal 348:191–201. doi:https://doi.org/10.1016/j.cej.2018.04.161.
- Yong, C., X. F. Liu, X. X. Miao. 2019. Targeted interfacial anchoring and wrapping of Fe3O4 nanoparticles onto graphene by PPy-derived-carbon for stable lithium-ion battery anodes – sciencedirect. Mater Res Bull 111:70–76.
- Yu, W., Y. Yang, and N. Graham. 2016. Evaluation of ferrate as a coagulant aid/oxidant pretreatment for mitigating submerged ultrafiltration membrane fouling in drinking water treatment. Chemical Engineering Journal 298:234–42. doi:https://doi.org/10.1016/j.cej.2016.03.080.
- Yuan, S., T. Man, and G. Wu. 2011. Destabilization of emulsions by natural minerals. J. Hazard. Mater. 192 (3):1882–85. doi:https://doi.org/10.1016/j.jhazmat.2011.06.047.
- Zhang, B., R. T. Hu, D. J. Sun. 2018. Fabrication of magnetite-graphene Oxide/MgAl-layered double hydroxide composites for efficient removal of emulsified oils from various oil-in-water emulsions. J Chem Eng Data 63 (12):4689–702.
- Zhang, C., J. Yu, T. Wang, S. Xu, C. Hu, W. Duan. 2020. Evaluation of ultraviolet aging resistance of bitumen modified with isobutyltriethoxysilane surface organic grafted LDH. Constr. Build. Mater. 241:118016. doi:https://doi.org/10.1016/j.conbuildmat.2020.118016.
- Zhang, J., D. Wen, J. Li, L. Qiao, J. Zheng, J. Sheng. 2007. Utilization of coal fly ash in the glass–ceramic production. J. Hazard. Mater. 149 (2):523–26. doi:https://doi.org/10.1016/j.jhazmat.2007.07.044.
- Zhang, Y., S. Li, W. Zhang, X. Chen, D. Hou, T. Zhao, X. Li. 2019. Preparation and mechanism of graphene oxide/isobutyltriethoxysilane composite emulsion and its effects on waterproof performance of concrete. Constr. Build. Mater. 208 (30):343–49. doi:https://doi.org/10.1016/j.conbuildmat.2019.03.015.
- Zhang, Y. H., F. X. Gan, L. I. Meng, J. Li, S.-Q. Li, S.-H. Wu. 2010. New integrated processes for treating cold-rolling mill emulsion wastewater. Journal of Iron and Steel Research (International) 17 (6):32–35. doi:https://doi.org/10.1016/S1006-706X(10)60110-0.
- Zhao, Z., G. Li, H. Ruan. 2021. Capturing magnesium ions via microfluidic hydrogel microspheres for promoting cancellous bone regeneration. American Chemical Society Nano (2021):34342981.
- Zhu, Z. 1993. Analysis of coagulation process behavior and prediction of optimum pH value for aluminum and ferric. Water Treatment Technology 19 (2):107–14.
- Zhu, Z. 2014. Theory on orthokinetic flocculation of cohesive sediment: A review. Journal of Geoscience and Environment Protection 12:11–11.