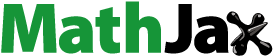
ABSTRACT
The photocatalyst Ag/Ag2O-modified ZnO, fabricated by a simple one-step calcination method, was applied into the degradation of organic pollutant dibutyl phthalate (DBP). Ag/ZnO and Ag2O/ZnO were prepared as a reference comparison. The prepared catalysts were evaluated by scanning electron microscope (SEM), transmission electron microscope (TEM), X-ray diffraction (XRD), X-ray photoelectron spectroscopy (XPS), BET surface area measurement, and photoluminescence spectra (PL) and UV–Vis diffuse reflectance spectroscopy (DRS) and electrochemical measurements. After the irradiation with ultraviolet light (352 nm), the solution was sampled and subjected to HPLC to evaluate the degradation efficiency of DBP. Ag/Ag2O/ZnO showed the best results with the excellent degradation of DBP. Ag/Ag2O/ZnO was four times more efficient, relative to zinc oxide alone. According to photocatalyst characterization, the total pore volume of photocatalyst was improved by loading Ag and Ag2O, suggesting an increase in the active sites. Also, the efficient electron transfer of Ag/Ag2O/ZnO was mainly responsible for the enhanced activity. The reaction mechanism for Ag/Ag2O/ZnO was determined to become a Z-scheme. From the radical scavenger tests, the main active species was identified as superoxide radicals. The stability of Ag/Ag2O/ZnO could be confirmed after five cycling reutilization. It was found from the radical scavenger test that •O2 – play an important role as the main reactive species in the photocatalytic degradation of DBP. Consequently, Ag/Ag2O/ZnO with a simple fabrication method seems to become one of the powerful photocatalyst for the photocatalytic degradation of organic pollutant in water.
Implications: This study discusses the usefulness of Ag/Ag2O/ZnO composites. This photocatalyst could be an approach to solve the environmental pollution caused by organic pollutants, which is a growing problem all over the world. In addition, the highly efficient photocatalyst Ag/Ag2O/ZnO is an inexpensive and reusable catalyst with great practical potential.
Furthermore, to the best of our knowledge, there are very few reports that have examined the combination of Ag, Ag2O and ZnO. In addition, the photocatalytic mechanism has not been understood. Here, we introduce Ag into Ag2O/ZnO to improve the photocatalytic performance and photostability, enhance the activity, and elucidate the mechanism of Ag/Ag2O/ZnO.
Introduction
The worldwide pollution is a global issue concerning water, air or soil quality. There are many cases of the contamination of drinking water, especially in developing countries, and there is an urgent need to develop inexpensive water purification technology. The photocatalytic reactions using artificial lamp devices (ultraviolet light) may become one of the inexpensive and powerful purification technologies. Moreover, in the future, it can be expected to be applied to visible light response.
As one of the toxic anthropogenic compounds, phthalate esters (PAEs), have been mainly used as plasticizers and additives to enhance the transparency, durability and flexibility of plastic materials since the 1930s (Cao Citation2009; Farahani et al. Citation2008). The European Union and the United States Environment Protection Agency classified phthalate esters as a top priority pollutant for risk assessment, mandating the reduction and control of phthalate pollution (Petrović et al. Citation2001).
Recently, semiconductors have been applied into the remediation of organic substances, such as dyes and pesticides, in water (Chong et al. Citation2010; Khavar et al. Citation2019), soil (Zhao et al. Citation2007) and air (Mo et al. Citation2009). Various chemical pollutants can be completely removed by the redox process that occurs at the irradiated semiconductor surface. However, few reports have been reported on the use of Zn-based semiconductor photocatalysts to degrade DBP in aqueous solution.
The research works on new treatment methods to remediate the recalcitrant compound have been actively investigated. Nowadays, advanced oxidation processes (AOP) are very appealing, due to the generation of highly oxidizing hydroxyl radicals. Among these processes, the photocatalytic treatment is especially suitable, since it cannot require the handling of expensive chemicals such as H2O2. Therefore, the photocatalytic degradation can be easily operated. In order to maximize photocatalytic performance, various semiconductor photocatalysts (ZnO (Khavar et al. Citation2019), CdS (Lei et al. Citation2020), CeO2 (Montini et al. Citation2016) and TiO2 (Fulekar et al. Citation2018; Katal et al. Citation2019; Shayegan, Lee, and Haghighat Citation2018)) have been widely explored in recent decades. Here, the present study has focused on the photocatalytic degradation of DBP with Zn-based photocatalyst and the mechanism of increased activity.
However, pure ZnO has some drawbacks, including rapid recombination of photogenerated electron hole pairs, photo corrosion and deactivation (Tsang et al. Citation2019). So as to overcome these shortcomings, the semiconductors (e.g., Bi2O3 (You et al. Citation2018), AgBr (Sun et al. Citation2021), TiO2 (Wang et al. Citation2019), and SrTiO3 (Jamil et al. Citation2017)) and precious metals (Au (Liang et al. Citation2020), Ag (Chong et al. Citation2010; Dutta, Singh, and Tyagia Citation2014; Dutta and Tyagi Citation2016; Weiwei et al. Citation2022), and Pt (Weon, Kim, and Choi Citation2018)) were used as co-catalysts for the improvement of the photocatalytic performance. Interestingly, it has been presorted that Ag/AgCl (Dutta, Singh, and Tyagia Citation2014), Ag/AgBr (Bhatt and Patel Citation2019), and Ag/AgI (Liu et al. Citation2019b) exhibited efficient and stable photochemical properties. This may be due to the Ag/Ag+ system. Contrary, Ag2O can effectively protect from photo corrosion (Chu et al. Citation2016). Liu et al. described the self-stabilizing mechanism of the Ag/Ag2O system (Liu et al. Citation2015). Gou et al. reported the Ag2O/TiO2-Zeolite composite and its enhanced solar light photocatalytic performance of norfloxacin (Gou et al. Citation2017).
To the best of our knowledge, there are very few reports on the photocatalytic remediation of DBP with Ag/Ag2O/ZnO in water. The present work has dealt with the usefulness of Ag/Ag2O/ZnO composite. In the present study, the introduction of Ag into Ag2O/ZnO to improve the photocatalytic performance and photostability has been evaluated, and the reaction mechanism of Ag/Ag2O/ZnO has been postulated.
Material and methods
Chemicals
Zinc oxide (ZnO) and acetonitrile (C2H3N) were purchased from Sigma-Aldrich, Inc. Silver acetate (CH3COOAg), 2-propanol ((CH3)2CHOH) and p-benzoquinone (C6H4O2) were prepared from FUJIFILM Wako Pure Chemical Corporation. Oxalic acid ((COOH)2) and methanol (CH3OH) were obtained from Kanto Chemical Co., Inc. Di-n-butyl phthalate (C6H4(COO(CH2)3CH3)2), ethylene diamine tetraacetic acid (C10H16N2O8), boric acid (B(OH)3) and sodium hydroxide (NaOH) were obtained from Nacalai tesque Inc. Deionized (DI) water was used for the entire synthesis process. All the chemical reagents were used without extra purification.
Synthesis of Ag/ZnO
In order to fabricate Ag-modified photocatalyst nanocomposite, 0.725 g of CH3COOAg, 0.500 g of (COOH)2 and 0.025 g of B(OH)3 were grinded for 10 minutes, and were sintered at 300°C. It has been reported that the addition of B(OH)3 reduced the Ag particle size (Khairy et al. Citation2019). The Ag was ground and mixed with commercial nanosized ZnO (Sigma-Aldrich, 50 ~ 70 nm) to obtain 4 wt% Ag/ZnO.
Synthesis of Ag2O/ZnO
Ag2O was synthesized by precipitation method. First, 0.295 g of sodium hydroxide was added to 50 mL of deionized water. Then, 0.716 g of CH3COOAg was added to sodium hydroxide aqueous solution and stirred for 10 minutes. Ag2O was formed as a black precipitate. The obtained Ag2O was collected after washing with deionized water for several times and dried at 60°C in a vacuum oven for overnight. The Ag2O was ground and mixed with commercial nanosized ZnO (Sigma-Aldrich) to obtain 4 wt% Ag2O/ZnO.
Synthesis of Ag/Ag2O/ZnO
In order to fabricate Ag/Ag2O photocatalyst nanocomposite, 0.725 g of CH3COOAg, 0.500 g of (COOH)2 and 0.025 g of B(OH)3 were grinded for 10 minutes, and were sintered to fabricate the composite for Ag and Ag2O. The sintering temperatures at 140, 170 and 200°C were evaluated. The Ag/Ag2O composite (4 wt%) was grinded and mixed with commercial nanosized ZnO (Sigma-Aldrich) to obtain Ag/Ag2O/ZnO.
Characterization of synthesized photocatalysts
The structures of photocatalysts were examined by X-ray diffraction (XRD) (RIGAKU Ultima IV) with Cu Kα radiation in the 2θ range of 10–80°. To determine the chemical composition of the photocatalysts, X-ray photoelectron spectroscopy (XPS) was performed on an X-ray photoelectron spectrometer (PHI Quantera SXM, Ulvac-Phi), equipped with an Al-Ka radiation source. Scanning electron microscopy (SEM) (S-4300, Hitachi) and transmission electron microscopy (TEM) (JEM-1011, JEOL Ltd.) were performed to observe the morphologies of the photocatalysts. Energy dispersive X-ray spectrometry (EDX) mapping images were collected using an JSM-5600LV (JEOL Ltd.). The surface properties of the photocatalysts were investigated by means of nitrogen adsorption–desorption experiments (BEL-SORP-miniII, Bel). Diffuse reflectance spectroscopy (UV–Vis DRS) was performed in a Jasco V-750 spectrometer. The excitation wavelength was set at 325 nm, and the emission was measured in the 345 ~ 600 nm range. The photoluminescence spectra (PL) were acquired using Jasco FP-8500 spectrofluorometer. The concentration of Ag in the solution after the treatment was measured by atomic absorption spectrophotometer (AA-7000, SIMADZU).
Electrochemical analysis was conducted on a Princeton/V3 electrochemical workstation using a typical setup of a three-electrode electrochemical system, where the Pt wire, the Ag/AgCl sat. KCl electrode and the photocatalyst were served as the counter electrode, the reference electrode, and the working electrode, respectively. Na2SO4 solution (0.2 mol L−1) was used as the electrolyte, and ultraviolet light was irradiated with a UV lamp (352 nm). Electrochemical impedance spectroscopy (EIS) measurements were conducted between 0.1 and 100 kHz with an amplitude of ± 100 mV and a perturbation voltage of 1.1 V. Chrono-amperometry (i-t) measurement was carried out at 1.2 V (vs. Ag/AgCl sat. KCl) over continuous light on-off cycles under illumination with 20 s light and dark with 10 s. The flat band potential obtained from Mott–Schottky analysis was obtained in the frequency range of 1000 Hz to 500 Hz, with the bias potential ranging from 0.5 V to −0.6 V (vs. Ag/AgCl sat. KCl).
Photocatalytic degradation of DBP
Thirty mg of the composite photocatalysts was added to 30 mL of 20 mg L–1 DBP in a Pyrex reactor, and the suspensions were stirred for 30 minutes in a dark place to attain adsorption–desorption equilibrium. After the irradiation with ultraviolet light (EFD15BLB-T, TOSHIBA CORP., λ = 352 nm, 0.25 mW/cm2), the solution was sampled at regular intervals, and the solution was subjected to high-performance liquid chromatography (HPLC) (PU7710, GL Sciences Inc.) with a reverse phase column (TSKgel ODS-100 V, 4.6 mm I.D. × 15 cm, 5 µm, Tosoh) and a UV detector (UV7750, GL Sciences Inc.) in order to determine the degradation efficiency. The chromatographic conditions were as follows: follow rate, 1.0 mL min–1; detection wavelength, 276 nm; mobile phase, 20:80 (v/v) water/methanol. The reproducibility of the photocatalytic degradation test (relative standard deviation) was within RSD 10% for more than 3 of run numbers.
Ag/Ag2O/ZnO photocatalytic activity was evaluated by degradation of model organic pollutants DBP. For the radical scavenging test, various scavengers were added to the reaction system under the same experimental conditions as those of photocatalytic degradation. A 1.20 mM ethylene diamine tetra acetic acid (EDTA) solution, 0.17 M 2-propanol solution and 3.15 mM p-benzoquinone (BQ) solution were used as scavengers for detecting the generation of h+, HO• and •O2–, respectively. All of the treatments were normally conducted at ambient temperature and initial solution pH 7.
Results and discussion
Characterization of photocatalysts
SEM
In order to confirm the surface morphology of the photocatalysts prepared in the present work, the prepared photocatalysts were observed by SEM (). As the sintering temperature was increased, it was found that the surface roughness increased. It has been reported that silver oxide changes to Ag metal upon heating at 250°C or higher (Gomathisankar et al. Citation2013). The reason for the surface roughness may be responsible to it that the surface is first replaced from silver oxide to silver. In (c), not only round particles but also rectangular ones were observed. In (d), the photocatalyst consisted only of silver and of fine particles with several hundreds of nanometers.
Figure 1. SEM images of Ag/Ag2O (sintering temperature 140°C (a), 170°C (b), 200°C (c)), Ag (d), Ag2O (e), Ag/Ag2O/ZnO (f) and ZnO (g).
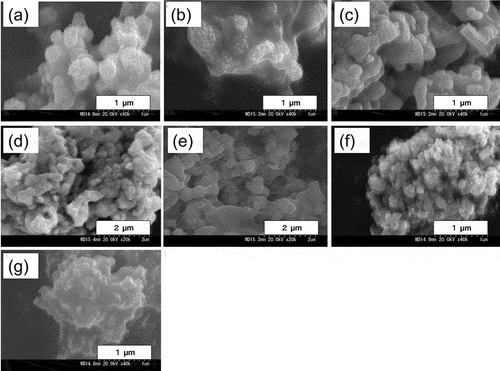
(e) shows a sheet-like surface morphology as well as particles. Silver oxide has a small surface area compared to the others, which is expected to be worse to its activity. (f) shows Ag/Ag2O mixed with ZnO calcined at 170°C. Hereafter, unless otherwise specified, Ag/Ag2O/ZnO refers to 4 wt% Ag/Ag2O/ZnO (4 wt% as total silver) with sintering temperature 170°C and time 3 hours. The surfaces of photocatalysts were rougher than that of ZnO alone in (g). When Ag and Ag2O were loaded on ZnO, the surface morphology became rougher, proving an increase in the active sites.
TEM
In order to confirm the microstructure of the photocatalysts, the photocatalysts were observed by TEM. (a) ~ (c) shows the morphological change of Ag/Ag2O with the various sintering temperatures. The spherical morphology of Ag/Ag2O was observed, due to the aggregation of uniform microspheres. These observations were consistent with the results of SEM images. As the sintering temperature increased, the particle size increased to ~10 nm, ~25 nm and ~30 nm at 140, 170 and 200°C, respectively. The long thin lines in (c) correspond to the rectangles in the SEM images. It was found in (d) that silver particles were agglomerated. On the other hand, very fine particles were also distributed. In (e) silver oxide, there was a mixture of particles and sheets. This tendency was clearly different from the others, which may be due to the preparation method by precipitation. (f) is a mixture of Ag/Ag2O (sintering temperature 170°C) and ZnO. Since the ZnO is 50 ~ 70 nm and Ag/Ag2O is 25 nm, the fine round particles on ZnO could be formed as Ag/Ag2O. Therefore, from the TEM image, Ag/Ag2O could be well modified and distributed on the surface of ZnO.
XRD
XRD patterns of prepared photocatalysts are shown in . (a) shows the XRD diffraction patterns of Ag/Ag2O at different sintering temperatures. The diffraction patterns obtained for all Ag/Ag2O samples show Ag and Ag2O. Each XRD diffraction pattern showed a characteristic peak at around 38°, which corresponded to (200) of Ag2O and (111) of Ag. It was found that the higher the sintering temperature, the amount of Ag increased and one of Ag2O decreased. The phenomena are consistent with the fact that silver oxide decomposes into silver and oxygen at temperatures above 250°C. The results that Ag was observed even at 140°C may be owing to the long sintering time of 3 hours, which accelerates the reaction. As the optimum condition, Ag/Ag2O was calcined at 170°C for 3 hours, unless otherwise noted.
Figure 3. XRD patterns of (a) Ag/Ag2O prepared at various sintering temperatures and (b) Ag, Ag2O and Ag/Ag2O.
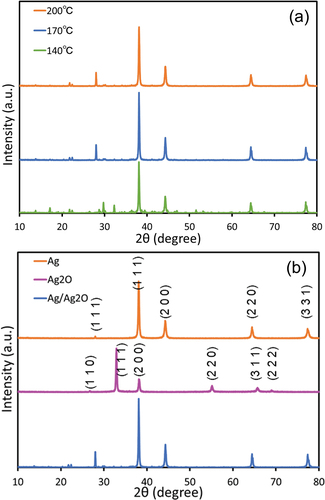
(b) shows the XRD patterns for the comparison of only Ag and Ag2O with Ag/Ag2O composite. For Ag, the patterns showed the (111), (200), (220) and (311) facets at 38.0°, 44.3°, 64.4° and 77.4°, respectively. The results were consistent with the standard data (JCPDS 04–0783). For Ag2O, the patterns showed the (110), (111), (200), (220), (311) and (222) facets at 26.7°, 32.3°, 38.0°, 55.1°, 65.7° and 68.9°, respectively. The results were consistent with the standard data (JCPDS 65–3289). Ag/Ag2O shows both peaks. Since the peak of silver appeared prominently, it is considered that the content ratio of silver was larger. The existence of boron could be confirmed by EDX analysis, as shown in Fig. S1.
XPS
X-ray photoelectron spectroscopy (XPS) enables the analysis of the composition and chemical bonding state of solid surfaces. Therefore, the chemical states of photocatalysts were identified by XPS. The results of Ag/Ag2O/ZnO are shown in . From (a), the peaks derived from O, Zn and Ag are observed, indicating that the photocatalyst was prepared correctly. From (b), the O 1s spectrum can be separated into three peaks. The peak located at 530 eV in O 1s means metal-oxygen bonds, suggesting the presence of Zn-O and Ag-O. The peak located at 532 eV might be hydroxyls, chemisorbed oxygen, or other oxygen groups (Zhao et al. Citation2019). The peak near 533 eV corresponds to chemisorbed water. In (c), two peaks at 1045 eV of Zn 2p3/2 and 1022 eV of Zn 2p1/2 are from two spin-orbit of Zn2+ (Deng et al. Citation2021). It shows peaks consistent with commercial zinc oxide, indicating that there was no change in structure. In (d), the Ag 3d spectrum illustrates that the primary peaks at 373.5 eV and 367.5 eV correspond to Ag 3d3/2 and 3d5/2, respectively. It suggests that Ag and Ag2O exist. Two peaks at 373.9 eV of Ag 3d3/2 and 367.9 eV of Ag 3d5/2 are from two spin-orbit of Ag0, and the other two peaks at 372.9 eV and 366.9 eV are signed as Ag+ (Zhao et al. Citation2019). Based on the data of Ag 3d spectra to calculate the peak area (4:1) of Ag0 and Ag+, the mole ratio of Ag:Ag2O was determined to be 8:1. It clearly demonstrated that a small portion of Ag2O was in the Ag/Ag2O/ZnO nanoparticles, which are the same results as the one with XRD.
BET
The specific surface area of photocatalysts was determined from the Brunauer–Emmett–Teller (BET) method. The adsorption volume, specific surface area, total pore volume and average pore size of Ag/Ag2O/ZnO, Ag/ZnO and Ag2O/ZnO are summarized in Table S1. The BET specific surface areas of Ag/ZnO (35.3 m2/g), Ag2O/ZnO (11.0 m2/g) and Ag/Ag2O/ZnO (12.2 m2/g) were larger, compared with pure ZnO. The total pore volumes for Ag/ZnO, Ag2O/ZnO and Ag/Ag2O/ZnO increased with the modification of silver, relative to pure ZnO, which was consistent with the SEM results. In general, it is known that the photocatalytic activity is better when the BET specific surface area and total pure volume is larger, because it facilitates the adsorption of reactants and desorption of products. In Fig. S2 (a), the pore size distribution (BJH) curve of Ag/Ag2O/ZnO shows the dominant size range of 2 ~ 20 nm. Fig. S2 (b) shows the adsorption/desorption isotherms of Ag/Ag2O/ZnO. The photocatalyst has a typical type IV isotherm, indicating the presence of mesopore pores (Al-Musawi et al. Citation2021). Also, both surfaces of Ag/ZnO and Ag2O/ZnO have mesopore pore (Fig. S3).
Wettability
It is known that the wettability of photocatalysts can be improved by the loading of metal (Vodyankin et al. Citation2021). A drop casting method for ZnO and Ag/Ag2O/ZnO photocatalyst was applied into the glass surface. One drop of 20 mg L–1 DBP was placed on the glass surface. The wettability of ZnO and Ag/Ag2O/ZnO is shown in (a) and (b), respectively. The wettability (angle) of ZnO was 128°, and on the other hand, that of Ag/Ag2O/ZnO was 53°. Because the wettability was greatly improved by the addition of Ag/Ag2O, the degradation of DBP may be enhanced by the affinity between the catalyst surface and DBP with Ag/Ag2O support.
PL
The activity for the photocatalytic reactions is greatly influenced by the recombination of photoexcited electrons and holes. The recombination of electron-hole pairs is known to release energy in the form of fluorescence luminescence (photoluminescence). The weak photoluminescence (PL) intensity implies the low electron-hole recombination rate and high photocatalytic activity. Therefore, in order to evaluate the photocatalyst activities, the PL of photocatalyst was investigated, as shown in . The pristine ZnO photocatalyst gave a strong emission peak at 513 nm, which arose from the high recombination of excited electron-hole pairs in the ZnO photocatalyst. However, the loading of Ag and Ag2O significantly reduced the emission peaks, suggesting the efficient separation and fast transfer of photogenerated electron into Ag and Ag2O. Because the band potentials of ZnO and Ag2O for Ag2O/ZnO may be close, the electrons can easily transfer between them. The situations of photocatalysts decreased the recombination rate of electrons and holes, resulting in improved photocatalytic activity. Therefore, it was shown that the combinations of different semiconductors were very effective in suppressing the recombination of electrons and holes. Furthermore, the depression of the recombination of electron-hole pairs may be a significant contribution to the DBP degradation. Ag2O/ZnO shows the lowest PL intensity, which was not consistent with the results of DBP degradation (described later). The results may be probably because the electrons produced by Ag2O are used for the reduction of silver oxide to metal silver, not for the reduction of oxygen.
DRS
Fig. S4 shows the UV-visible absorption spectra of ZnO, Ag/ZnO, Ag2O/ZnO and Ag/Ag2O/ZnO. The UV-visible absorption spectra of photocatalysts were evaluated by the Kubelka-Munk transformation of the diffuse reflection spectra obtained from the DRS measurements. In Fig. S4, the absorption edge of ZnO was about 390 nm, and significant change in the absorption edge was scarcely observed by loading silver and silver oxide. The band gap energy was calculated from the diffuse reflection spectra, according to the following equation (Dhanasankar, Purushothaman, and Muralidharan Citation2010; Xie et al. Citation2018).
α is the absorption coefficient, h Planck’s constant, ν the frequency and Eg the band gap energy. n is the value determined by the interband excitation of electrons; n = 1/2 for the direct bandgap and n = 2 for the indirect bandgap. n = 1/2 is used for ZnO and Ag2O since the interband excitation is direct. The bandgap energies of the photocatalysts are plotted in . The band gap energies of ZnO, Ag/ZnO, Ag2O/ZnO and Ag/Ag2O/ZnO were estimated to be 3.274 eV, 3.268 eV, 3.262 eV and 3.267 eV, respectively. The significant decrease in the bandgap energy could hardly be observed. This may be due to the lack of interface charge transfer from the valence band of ZnO to Ag and Ag2O. The bandgap could have little significant effect on the enhancement of photocatalytic activity.
Mott-schottly
Fig. S5 shows the Mott-Schottky of ZnO, Ag/ZnO, Ag2O/ZnO and Ag/Ag2O/ZnO. From the results, the flat band potentials of the ZnO, Ag/ZnO, Ag2O/ZnO and Ag/Ag2O/ZnO were –0.09 V, –0.04 V, –0.09 V and –0.03 V, respectively. Since the flat-band potentials of the prepared photocatalysts were more positive than redox potential for the superoxide radicals, the •O2 – radicals could not be directly produced by the conduction band electrons of the prepared photocatalysts.
Nyquist plots
shows the Nyquist plots of ZnO and Ag/ZnO, Ag2O/ZnO and Ag/Ag2O/ZnO. The arc radius of the Nyquist plot represents the resistance of the interfacial layer on the surface of the electrode. The smaller the arc of radius, the greater the mobility and separation of the charge carriers at the interface (Liu et al. Citation2019a). The radii were smaller in the order of Ag/Ag2O/ZnO, Ag2O/ZnO, Ag/ZnO and ZnO. Therefore, Ag/Ag2O/ZnO had the smallest arc radius in the impedance plot, causing the fast charge transfer for the photocatalytic degradation of DBP. Therefore, the electrons may be easily available for the radical generation and the photocatalytic activity may be improved by the addition of Ag/Ag2O.
Transient photocurrent responses
The transient photocurrent responses were studied, so as to further clarify the transport efficiency of charge carriers in ZnO, Ag/ZnO, Ag2O/ZnO and Ag/Ag2O/ZnO, with on/off cycles of UV light irradiation, as shown in Fig. S6. The transient photocurrent of ZnO was the lowest. Because contrary the photocurrent of Ag/Ag2O/ZnO being the largest, Ag/Ag2O/ZnO promoted the interfacial transfer of photogenerated charge carriers and increased the separation efficiency of electron–hole pairs. These results agreed with the results of Nyquist plots. The better charge carrier transfer efficiency may contribute to the excellent photocatalytic degradation of DBP.
DBP decomposition
In order to investigate the optimum calcination temperature of Ag2O, the photocatalytic DBP degradation was carried out with Ag/Ag2O/ZnO which was fabricated at various sintering temperatures. Four wt% of Ag/Ag2O was loaded on ZnO at different calcination temperatures of 140, 170 and 200°C for 3 h. (a) shows that Ag/Ag2O/ZnO at sintering temperature 170°C was the most efficient, and the photocatalytic degradation of DBP could be completely conducted after 60 min of light irradiation. Therefore, the optimal sintering temperature of Ag/Ag2O was determined to be 170°C. (b) shows the pseudo-first order kinetic for the photocatalytic DBP degradation with Ag/Ag2O/ZnO at various sintering temperatures. The results indicate it that the ratio of Ag to Ag2O is an important indicator of the photocatalytic activity. Ag2O can start to decompose into Ag and O2 (2Ag2O → 4Ag + O2) at 250°C. Therefore, Ag/Ag2O/ZnO at sintering temperature 200°C contained relatively larger Ag amount and had a lower degradation efficiency, due to the reduction of the formation of superoxide anion radicals (•O2–) during the photocatalytic degradation. On the other hand, in the case of Ag/Ag2O/ZnO at sintering temperature 140°C, the ratio of Ag to Ag2O may be unsuitable for the efficient photocatalytic degradation of DBP.
Figure 9. (a) Effect of sintering temperatures on the photocatalytic degradation of DBP with Ag/Ag2O/ZnO. (b) Pseudo-first order kinetic over Ag/Ag2O/ZnO at various sintering temperatures.
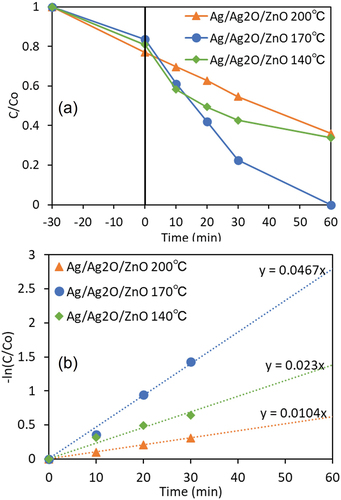
The dark adsorption/desorption equilibrium of DBP in the photocatalysts was completely reached after 30 minutes (Fig. S7). After the stirring in the dark for 30 minutes, the DBP solutions were irradiated with UV for 60 minutes. shows the photocatalytic activity of various photocatalysts. The photocatalytic degradation efficiency of pure ZnO was 0.57 (43%), while the efficiencies 0.34 (66%), 0.33 (67%) and 0 (100%) with Ag/ZnO, Ag2O/ZnO and Ag/Ag2O/ZnO, respectively. Hence, the reaction–catalytic effect of Ag and the suppression of electron-hole pair recombination by Ag2O were very effective for enhancing the photocatalytic activity of ZnO. The rate constant (kapp) for the pseudo-first order kinetic for the photocatalytic DBP degradation with Ag/Ag2O/ZnO was 0.047 min−1, which was better compared with that of TiO2 (0.033 min−1 (Kaneco et al. Citation2006)) and of graphene/TiO2 (0.040 min−1 (Wang et al. Citation2019)).
Figure 10. (a) Effect of silver addition on photocatalytic degradation of DBP with ZnO. (b) Pseudo-first order kinetic with photocatalysts.
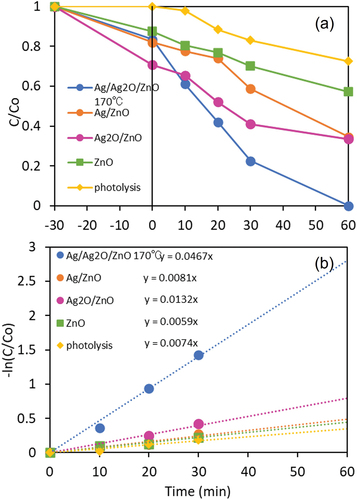
The photo corrosion of Ag/Ag2O/ZnO was investigated for the photocatalyst stability. First, the leaching amount of Ag in the sample solution was measured by the atomic absorption spectrometric analysis (Table S2). Because the concentration of Ag was 0.73 μg L–1 in the sample solution, the eluted Ag amount was 22 ng, which was only 0.0018% of the photocatalyst Ag/Ag2O/ZnO. Although Ag2O is an unstable substance, the coexistence of Ag could prevent the leaching (Zhao et al. Citation2019). Next, the leaching amount of Zn in the sample solution was measured by the colorimetric method (Fig. S8 (a)). The eluted Zn amount was 9 µg, which is only 0.03% of the catalyst Ag/Ag2O/ZnO. The photocatalyst Ag/Ag2O/ZnO was measured by XRD after 60 min of irradiation, as illustrated in Fig. S8 (b). The change in the peaks could be seldom observed, indicating the good stability of the photocatalyst.
The recyclability for the photocatalytic DBP degradation using Ag/Ag2O/ZnO photocatalysts was studied. The degradation efficiencies of DBP could keep more than 80% or more after 5 cycles, meaning a high stability of Ag/Ag2O/ZnO (Fig. S9). The effect of initial pH on the photocatalytic treatment of DBP is shown in Fig. S10. The pH adjustment was performed with HNO3 (1 M) and NaOH (1 M) solutions. Since zinc oxide is photodissolved below pH 4, the initial pH of sample solution was studied in the range of 5 to 11. The maximum photocatalytic degradation was observed at pH 7. The fact can become cost-effective, because of the unnecessary of chemical treatment including neutralization process. The degradation efficiency dropped significantly under basic conditions.
In order to investigate the main reactive species involved in the photocatalytic degradation of DBP over Ag/Ag2O/ZnO, the radical scavenging tests were conducted under the same conditions as the degradation. EDTA, 2-propanol, and benzoquinone (BQ) were added to the reaction system for detecting the generation of h+, HO• and •O2–, respectively. shows that the addition of BQ led to the suppression of the photocatalytic degradation. In addition, the values of rate constant (kapp) for the pseudo-first order kinetic for the photocatalytic DBP degradation were calculated from , and the rate constants were 0.015 min−1 for benzoquinone toward •O2–, 0.031 min−1 for EDTA toward h+, 0.035 min−1 for 2-propanol toward HO• and 0.047 min−1 without scavenger. These results indicate it that •O2– plays an important role as the main reactive species in the photocatalytic degradation of DBP in aqueous solution.
Figure 11. (a) Effect of radical scavengers on the photocatalytic degradation of DBP with Ag/Ag2O/ZnO and (b) their pseudo-first order kinetic over Ag/Ag2O/ZnO.
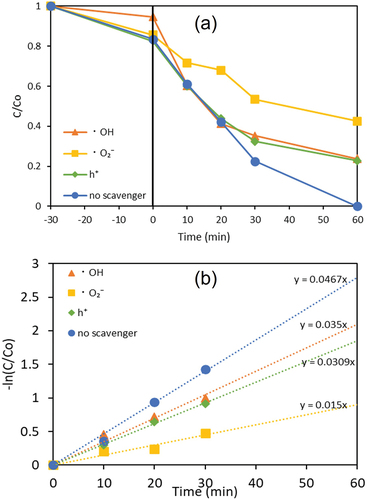
The most photocatalytic activity in the degradation of DBP was observed with a ternary composite photocatalyst composed of Ag2O, Ag and ZnO. The valence band (VB) and conduction band (CB) positions of ZnO, relative to the standard hydrogen electrode (NHE) were determined from the characterization as follows.
The VB and CB positions of Ag2O relative to the standard hydrogen electrode (NHE) were set to the following values. The CB potential (–0.27 V) of Ag2O was estimated from the Mott-schottky plot in Fig. S11, the VB potential of Ag2O was calculated to be 1.38 V based on the band gap value of 1.65 V (Fig. S12).
The excitation of electrons in ZnO and Ag2O by light irradiation generates the photogenerated electrons and holes. The photogenerated electrons can transfer to Ag on ZnO and promote the formation of •O2– by the one-electron reduction reaction of dissolved O2, causing the degradation reaction of DBP. Because the redox potential of O2 is – 0.33 eV (Wang et al. Citation2011), it was impossible to produce •O2– anion radicals with the CB electrons in ZnO and Ag2O. Therefore, the photogenerated electrons in Ag2O and ZnO may transfer to the silver metal, where they promote the formation of •O2– anion radicals, resulting in the decomposition reaction of DBP. The CB electrons in ZnO can combine with VB holes in Ag2O for the excellent separation of electrons and holes for Z–scheme. Ag and Ag2O acts as the inhibitor of recombination of electron-hole pairs in the catalyst.
Moreover, the holes in the valence band of ZnO can produce HO• hydroxy radicals by the oxidation reaction with H₂O, resulting in the photocatalytic DBP decomposition, since the oxidation potential of H2O is 2.3 eV (Wang et al. Citation2011; Yu et al. Citation2019). Thus, the enhanced photocatalytic degradation may be owing to 1) suppression of the recombination efficiency of electron-hole pairs by adding Ag/Ag2O and 2) the promotion of the generation of •O2– superoxide anion radical by modifying Ag onto the surface. Therefore, the application of Ag/Ag2O/ZnO onto the photocatalytic degradation of DBP was very effective, as shown in .
Figure 12. Postulated mechanism for the photocatalytic degradation of DBP with Ag/Ag2O/ZnO in water.
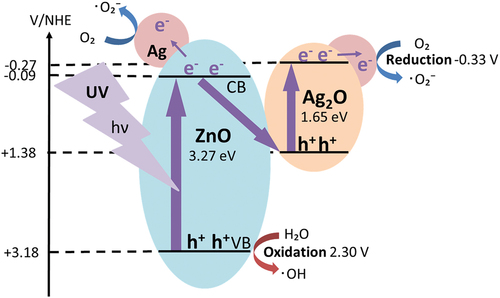
The expected degradation pathway is shown in Fig. S13 (Kaneco et al. Citation2006). Two possible attack sites for the HO• are the aliphatic chain and the aromatic ring in the structure of DBP. When the HO• attacks the aliphatic chain, many intermediate products (a)-(l) may be generated during the photocatalytic degradation of DBP. These single aromatic intermediate products are presumably further oxidized with ring breaking reactions to aliphatic compounds containing formic acid, acetic acid, and acetaldehyde (Horikoshi et al. Citation2004; Watanabe et al. Citation2003). The attack of HO• radicals to the aromatic ring leads to the formation of hydroxy and dihydroxy photoproducts (hydroxylated on the aromatic ring) for DBP. Further attack of HO• will be given to the aliphatic chain of the hydroxylated product. After the attack of the HO• on the two carbon atoms at the α-position with respect to the carboxyl group, the positions would be cleaved, forming intermediates (m) and (n) with long-chain structure. If intermediates with long-chain structures are generated, their photocatalytic degradation may be more difficult than that of the ring moiety. If such long-chain intermediates are generated, the mineralization of CO2 will be slower in the photocatalytic degradation of DBP.
Conclusion
In the present study, the photocatalytic activity based on ZnO for the degradation of organic pollutant dibutyl phthalate could be improved. The photocatalysts Ag/Ag2O–modified ZnO were fabricated by a simple one-step calcination method. A dramatic improvement in photocatalytic activity was observed by the modification of Ag/Ag2O on ZnO. The presence of both Ag and Ag2O was very important, and Ag/Ag2O/ZnO could completely decompose 20 mg L–1 of DBP during 60 minutes under UV irradiation. The efficiency with Ag/Ag2O/ZnO was about four times better than that of ZnO photocatalyst. The addition of Ag/Ag2O was very effective for the suppression of photo corrosion for the photocatalysts. According to the characterization, it is possible the suppression of electron-hole pair recombination by Ag and Ag2O, and the promotion of generation of •O2– superoxide anion radical may be due to the modifying Ag onto the surface. The developed photocatalyst has become one of the promising materials in the field of organic pollutant decomposition.
Supplementary_data_latest.docx
Download MS Word (1.1 MB)Data availability statement
The authors confirm that the data supporting the findings of this study are available within the article and its supplementary materials.
Disclosure statement
No potential conflict of interest was reported by the author(s).
Supplementary material
Supplemental data for this paper can be accessed on the publisher’s website
Additional information
Funding
Notes on contributors
Erisa Sugiura
Erisa Sugiura received a Master of Engineering degree in 2022, and her degree was associated with the environmental chemistry.
Mai Furukawa
Mai Furukawa received a Doctor of Engineering degree in 2020, and is research associate at Department of Chemistry, Graduate School of Engineering, Mie University.
Ikki Tateishi
Ikki Tateishi received a Doctor of Engineering degree in 2020, and is assistant professor for Global Environment Center for Education & Research, Mie University.
Hideyuki Katsumata
Hideyuki Katsumata received a Doctor of Science degree in 1998, and is Associate Professor at Department of Chemistry, Graduate School of Engineering, Mie University.
Satoshi Kaneco
Satoshi Kaneco received a Doctor of Engineering degree in 2000, and is Full Professor at Department of Chemistry, Graduate School of Engineering, Mie University.
References
- Al-Musawi, T., P. Rajiv, N. Mengelizadeh, F. S. Arghavan, and D. Balarak. 2021. Photocatalytic efficiency of CuNiFe2O4 nanoparticles loaded on multi-walled carbon nanotubes as a novel photocatalyst for ampicillin degradatio. J. Mol. Liq 337:116470. doi:10.1016/j.molliq.2021.116470.
- Bhatt, D. K., and U. D. Patel. 2019. Mechanism underlying visible-light photocatalytic activity of Ag/AgBr: Experimental and theoretical approaches. J. Phys. Chem. Solids 135:109118. doi:10.1016/j.jpcs.2019.109118.
- Cao, X. 2009. Phthalate Esters in foods: sources, occurrence, and analytical methods. Food Sci. And Food Safety 9:21–43. doi:10.1111/j.1541-4337.2009.00093.x. [Web of Science], [Pub Med], [Google schoolar].
- Chong, M. N., B. Jin, C. W. K. Chow, and C. Saint. 2010. Recent developments in photocatalytic water treatment technology: A review. Water Res. 44:2997–3027. [Google schoolar], [Pub Med]. doi:10.1016/j.watres.2010.02.039.
- Chu, H., X. Liu, J. Liu, J. Li, T. Wu, H. Li, W. Lei, Y. Xu, and L. Pan. 2016. Synergetic effect of Ag2O as co-catalyst for enhanced photocatalytic degradation of phenol on N-TiO2. Mater. Sci. Eng 211:128–34. doi:10.1016/j.mseb.2016.06.010.
- Deng, H., X. Fei, Y. Yang, J. Fan, J. Yu, B. Cheng, and L. Zhang. 2021. S-scheme heterojunction based on p-type ZnMn2O4 and n-type ZnO with improved photocatalytic CO2 reduction activity. Chem. Eng. Journal 409:127377. doi:10.1016/j.cej.2020.127377.
- Dhanasankar, M., K. K. Purushothaman, and G. Muralidharan. 2010. Optical, structural and electrochromic studies of molybdenum oxide thin films with nanorod structure. Solid State Sci 12:246–51. doi:10.1016/j.solidstatesciences.2009.10.021.
- Dutta, D. P., A. Singh, and A. K. Tyagia. 2014. Ag doped and Ag dispersed nano ZnTiO3: Improved photocatalytic organic pollutant degradation under solar irradiation and antibacterial activity. J. Environ. Chem. Eng 2:2177–87. doi:10.1016/j.jece.2014.09.015.
- Dutta, D. P., and A. K. Tyagi. 2016. Facile sonochemical synthesis of Ag modified Bi4Ti3O12 nanoparticles with enhanced photocatalytic activity under visible light. Mater. Res. Bull 74:397–407. doi:10.1016/j.materresbull.2015.11.005.
- Farahani, H., M. R. Ganjali, R. Dinarvand, and P. Norouzi. 2008. Screening method for phthalate esters in water using liquid-phase microextraction based on the solidification of a floating organic microdrop combined with gas chromatography–mass spectrometry. Talanta 76:718–23. doi:10.1016/j.talanta.2008.03.002.
- Fulekar, J., D. P. Dutta, B. Pathaka, and M. H. Fulekar. 2018. Novel microbial and root mediated green synthesis of TiO2 nanoparticles and its application in wastewater remediation. J. Chem. Technol. Biotechnol 93:736–43. doi:10.1002/jctb.5423.
- Gomathisankar, P., K. Hachisuka, H. Katsumata, T. Suzuki, K. Funasaka, and S. Kaneco. 2013. Photocatalytic Hydrogen production from Aqueous Na2S + Na2SO3 solution with B-doped ZnO. ACS Sustain. Chem. Eng 1:982–88. doi:10.1021/sc400061w.
- Gou, J., Q. Ma, X. Deng, Y. Cui, H. Zhang, X. Cheng, X. Li, M. Xie, and Q. Cheng. 2017. Fabrication of Ag2O/TiO2-Zeolite composite and its enhanced solar light photocatalytic performance and mechanism for degradation of norfloxacin. Chem. Eng. J 308:818–26. doi:10.1016/j.cej.2016.09.089.
- Horikoshi, S., A. Tokunaga, H. Hidaka, and N. Serpone. 2004. Environmental remediation by an integrated microwave/UV illumination method: VII. Thermal/non-thermal effects in the microwave-assisted photocatalyzed mineralization of bisphenol-A. J. Photochem. Photobiol. A: Chem 162:33–40. doi:10.1016/S1010-6030(03)00312-5.
- Jamil, T. S., H. A. Abbas, A. M. Youssief, E. S. Mansor, and F. F. Hammad. 2017. The synthesis of nano-sized undoped, Bi doped and Bi, Cu co-doped SrTiO3 using two sol–gel methods to enhance the photocatalytic performance for the degradation of dibutyl phthalate under visible light. C R Chim 20:97–106. doi:10.1016/j.crci.2016.05.022.
- Kaneco, S., H. Katsumata, T. Suzuki, and K. Ohta. 2006. Titanium dioxide mediated photocatalytic degradation of dibutyl phthalate in aqueous solution—kinetics, mineralization and reaction mechanism. Chem. Eng. J 125:59–66. doi:10.1016/j.cej.2006.08.004.
- Katal, R., S. Masudy-Panah, M. Tanhaei, M. H. D. A. Farahani, and H. Jiangyong. 2019. A review on the synthesis of the various types of anatase TiO2 facets and their applications for photocatalysis. Chem. Eng. J 384:123384. doi:10.1016/j.cej.2019.123384.
- Khairy, M., M. M. Mohamed, S. M. Reda, and A. Ibrahem. 2019. Effect of annealing temperature and Ag contents on the catalytic activity and supercapacitor performances of Ag@Ag2O/RGO nanocomposites. Mater. Sci. Eng. B 242:90–103. doi:10.1016/j.mseb.2019.03.007.
- Khavar, A. H. C., G. Moussavi, A. R. Mahjoub, R. Luque, D. Rodríguez-Padrón, and M. Sattari. 2019. Enhanced visible light photocatalytic degradation of Acetaminophen with Ag2S-ZnO@rGO core-shell microsphere as a novel catalyst: Catalyst preparation and characterization and mechanistic catalytic experiments, Sep. Purif. Technol 229:115803. [Pub Med], [Google schoolar]. doi:10.1016/j.seppur.2019.115803.
- Lei, X., T. Xu, W. Yao, Q. Wu, and R. Zou. 2020. Hollow hydroxyapatite microspheres modified by CdS nanoparticles for efficiently photocatalytic degradation of tetracycline. J. Taiwan Inst. Chem. E 106:148–58. doi:10.1016/j.jtice.2019.10.023.
- Liang, X., P. Wang, Y. Gao, H. Huang, F. Tong, Q. Zhang, Z. Wang, Y. Liu, Z. Zheng, Y. Dai, et al. 2020. Design and synthesis of porous M-ZnO/CeO2 microspheres as efficient plasmonic photocatalysts for nonpolar gaseous molecules oxidation: Insight into the role of oxygen vacancy defects and M=Ag, Au nanoparticles. Appl. Catal. B: Environ 358:118151. doi:10.1016/j.apcatb.2019.118151.
- Liu, C., C. Cao, X. Luo, and S. Luo. 2015. Ag-bridged Ag2O nanowire network/TiO2 nanotube array p-n heterojunction as a highly efficient and stable visible light photocatalyst. J. Hazard Mater 285:319–24. doi:10.1016/j.jhazmat.2014.12.020.
- Liu, J., P. Wang, W. Qu, H. Li, L. Shi, and D. Zhang. 2019a. Nanodiamond-decorated ZnO catalysts with enhanced photocorrosion-resistance for photocatalytic degradation of gaseous toluene. Appl. Catal. B: Environ 257:117880. doi:10.1016/j.apcatb.2019.117880.
- Liu, W., C. Wei, G. Wang, X. Cao, Y. Tan, and S. Hu. 2019b. In situ synthesis of plasmonic Ag@AgI/TiO2 nanocomposites with enhanced visible photocatalytic performance. Ceram. Int 45:17884–89. doi:10.1016/j.ceramint.2019.06.004.
- Mo, J., Y. Zhang, Q. Xu, J. J. Lamson, and R. Zhao. 2009. Photocatalytic purification of volatile organic compounds in indoor air: A literature review. Atmospheric Environ 43:2229–46. doi:10.1016/j.atmosenv.2009.01.034.
- Montini, T., M. Melchionna, M. Monai, and P. Fornasiero. 2016. Fundamentals and catalytic applications of CeO2-based materials. Chem. Rev 116:5987–6041. doi:10.1021/acs.chemrev.5b00603.
- Petrović, M., E. Eljarrat, M. J. L. Alda, and D. Barceló. 2001. Analysis and environmental levels of endocrine-disrupting compounds in freshwater sediments. Trends in Analyt. Chem 20 (Google scoolar):637–48. doi:10.1016/S0165-9936(01)00118-2.
- Shayegan, Z., C. Lee, and F. Haghighat. 2018. TiO2 photocatalyst for removal of volatile organic compounds in gas phase - A review Chem. Eng. J 334:2408–39. doi:10.1016/j.cej.2017.09.153.
- Sun, J., Y. Hou, Z. Yun, L. Tu, Y. Yan, S. Qin, S. Chen, D. Lan, H. Zhu, and S. Wang. 2021. Visible-light-driven Z-scheme Zn3In2S6/AgBr photocatalyst for boosting simultaneous Cr(VI) reduction and metronidazole oxidation: Kinetics, degradation pathways and mechanism. J. Hazard. Mater 419:126543. doi:10.1016/j.jhazmat.2021.126543.
- Tsang, C. H. A., K. Li, Y. Zeng, W. Zhao, T. Zhang, Y. Zhan, R. Xie, D. Y. C. Leung, and H. Huang. 2019. Titanium oxide based photocatalytic materials development and their role of in the air pollutants degradation: Overview and forecast. Environ Int 125:200–28. doi:10.1016/j.envint.2019.01.015.
- Vodyankin, A. A., Y. A. Belik, V. I. Zaikovskii, and O. V. Vodyankina. 2021. Investigating the influence of silver state on electronic properties of Ag/Ag2O/TiO2 heterojunctions prepared by photodeposition. J. Photochem. Photobiol. A: Chem 408:113091. doi:10.1016/j.jphotochem.2020.113091.
- Wang, X., S. Li, H. Yu, J. Yu, and S. Liu. 2011. Ag2O as a New Visible-Light Photocatalyst: Self-Stability and High Photocatalytic Activity. Chem. A Europ. J 17:7777. doi:10.1002/chem.201101032.
- Wang, G., Q. Zhang, Q. Chen, X. Ma, Y. Xin, X. Zhu, D. Ma, C. Cui, J. Zhang, and Z. Xiao. 2019. Photocatalytic degradation performance and mechanism of dibutyl phthalate by graphene/TiO2 nanotube array photoelectrodes. Chem. Eng. J 358:1083–90. doi:10.1016/j.cej.2018.10.039.
- Watanabe, N., S. Horikoshi, H. Kawabe, Y. Sugie, J. Zhao, and H. Hidaka. 2003. Photodegradation mechanism for biphenol A at the TiO2/H2O interfaces. Chemosphere 52:851–59. doi:10.1016/S0045-6535(02)00837-8.
- Weiwei, L. Z., L. P. Xu, H. Wang, X. Wei, K. Yao, and S. Peng. 2022. Plasmon-mediated activation of persulfate for efficient photodegradation of ionic liquids over Ag@Pd core–shell nanocubes. Appl. Catal. B: Environ 301:120751. doi:10.1016/j.apcatb.2021.120751.
- Weon, S., J. Kim, and W. Choi. 2018. Dual-components modified TiO2 with Pt and fluoride as deactivation-resistant photocatalyst for the degradation of volatile organic compound. Appl. Catal. B: Environ 220:1–8. doi:10.1016/j.apcatb.2017.08.036.
- Xie, Z., Y. Feng, F. Wang, D. Chen, Q. Zhang, Y. Zeng, W. Lv, and G. Liu. 2018. Construction of carbon dots modified MoO3/g-C3N4 Z-scheme photocatalyst with enhanced visible-light photocatalytic activity for the degradation of tetracycline. Appl. Catal. B: Environ 229:96–104. doi:10.1016/j.apcatb.2018.02.011.
- You, S., Y. Hu, X. Liu, and C. Wei. 2018. Synergetic removal of Pb(II) and dibutyl phthalate mixed pollutants on Bi2O3-TiO2 composite photocatalyst under visible light, Appl. Catal. B: Environ 232:288–98. doi:10.1016/j.apcatb.2018.03.025.
- Yu, N., H. Peng, L. Qiu, R. Wang, C. Jiang, T. Cai, Y. Sun, Y. Li, and H. Xiong. 2019. New pectin-induced green fabrication of Ag@AgCl/ZnO nanocomposites for visible light triggered antibacterial activity. Int. J. Biol. Macromol 141:207–17. doi:10.1016/j.ijbiomac.2019.08.257.
- ZHAO, X., X. QUAN, S. CHEN, H. ZHAO, and Y. LIU. 2007. Photocatalytic remediation of γ-hexachlorocyclohexane contaminated soils using TiO2 and montmorillonite composite photocatalyst. J. Environ. Sci 19:358–61. doi:10.1016/S1001-0742(07)60059-X.
- Zhao, W., J. Zhang, F. Zhu, F. Mu, L. Zhang, B. Dai, J. Xu, A. Zhua, C. Sun, and D. Y. C. Leung. 2019. Study the photocatalytic mechanism of the novel Ag/p-Ag2O/n-BiVO4 plasmonic photocatalyst for the simultaneous removal of BPA and chromium (VI). Chem. Eng. Journal 361:1352–62. doi:10.1016/j.cej.2018.12.181.