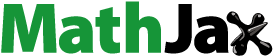
ABSTRACT
Bioaerosols play a momentous role in the transmission of human infectious diseases, so there has been increasing concern over their exposure in recent years. Bioaerosol monitor is crucial in environmental fields. Based on the universal existence of Adenosine triphosphate (ATP) in bioaerosols, ATP bioluminescence can be used as a powerful technique to detect bioaerosols without interference from non-bioaerosols. When ATP is released from bioaerosols, they can quantify microbial biomass by ATP bioluminescence. In this review, we provide the latest methodological improvements that enable more reliable quantification of bioaerosols in complex environmental samples, especially the use of ATP bioluminescence in this era of technological advancement via the following routes: lower sample content for the trace existence of bioaerosols in the atmosphere, higher sensitivity of ATP bioluminescence reaction system and shorter process times. We also highlight the new techniques in improving the efficiencies of these monitoring processes. The purpose of this paper is to make more people realize the great potential of the ATP bioluminescence system for monitoring airborne microorganisms. Additionally, the present work intends to increase people’s awareness of developing novel technology combined with ATP bioluminescence reaction system to realize rapid, real-time, and sensitive sensing of bioaerosols.
Implications: The ATP bioluminescence methodology can not only eliminate the interference of co-existing nonbiological (fluorescent or PM) but also significantly improve the efficiency of bioaerosol. Recent progresses, such as the application of ATP fluorescence technology in bioaerosol monitoring, indicating that the efficiency and sensitivity are possible to be further improved. Nevertheless, there is no reviews address these advances and deeply analyze the application of ATP fluorescence technology in this field. his contribution will attract wide attention from both academic and industrial communities of this field, as well as researchers engaging in environmental monitoring. Furthermore, the strategies and techniques of studying the ATP bioluminescence reviewed here is instructive for environment monitoring in various fields. Therefore, in view of significance and broad interest, we feel strongly that our critical review is very essential to the field of public health security, pharmaceutics, anti-bioterrorism, etc., and would like it to be published in Journal of the Air & Waste Management Association.
Introduction
Biological aerosols (airborne microorganisms) account for about 10% of aerosols in urban and rural areas (Monn Citation2001) and can be found in almost any indoor or outdoor air environment (Okten and Asan Citation2012; Prussin, Garcia, and Marr Citation2015). A considerable number of experts and scholars have conducted many studies in the field of bioaerosol (i.e., particulate matter of biological origin in the air) detection over the past decades (Choi et al. Citation2017). They include fragments of living or dead microorganisms and biological materials: bacteria, viruses, pollen grains, and mycelium fragments, fungal spores, plant fragments, and so on. The air contains a large number of bioaerosols of different sizes, mainly the inhalable and breathable parts: the diameter of viruses is 0.01–0.1 mm, the diameter of bacteria is 0.25–20 μm, the diameter of fungi is 1–30 mm, and the diameter of pollen grains, plant fragments, and animal fragments is 10 ≥ 100mm4. Due to their small size and lightweight, bioaerosols are easily transferred from various environments (Van Leuken et al. Citation2016).
Recently, biological aerosols have become a significant problem that may threaten human health, including acute toxic reactions, infectious diseases, and allergies. While the link between bioaerosols and inflammation is well established and the health risks associated with bioaerosols have been identified, the role of these particles in triggering or exacerbating disease remains unresolved, and the exposure-response relationship identified (Camatini et al. Citation2012). Since the concentration of target microbes is low and the sample matrix has potential complexity, detecting bioaerosol is challenging. Human activities make detection of target microorganisms complex or even impossible because detection can be interfered with by industrial products released into the atmosphere. In contrast to chemical agents, biological agents are well suited for military or bioterrorism purposes because of their delayed effects, infectivity, and potential mass casualties. For these reasons, there have been no specific guidelines for bioaerosol concentration levels, and bioaerosol standards based on health risk assessments have not yet been developed (Kim, Kabir, and Jahan Citation2018). Hence, it is necessary to assess the concentration of bioaerosols in contaminated indoor/outdoor or occupational environments.
At present, there are many commonly used biological aerosol detection methods (). Colony counting technology based on culture is the most widely used bioaerosol detection technology at present. It is widely used in indoor and outdoor air monitoring of bacteria and fungi (Byeon et al. Citation2008; Górny, and Krysińska-Traczyk Citation1999; Meklin et al. Citation2002). Nevertheless, the traditional culture-based approach always takes several days, which may be one of the most serious disadvantages of this approach.
Table 1. Summary of common biological aerosol detection technology (Choi et al. Citation2017; Marcovecchio and Perrino Citation2021; Monn Citation2001; Okten and Asan Citation2012; Van Leuken et al. Citation2016).
In view of the shortcomings of traditional culture-based methods (Shah and Naseby Citation2015), methods for quick detection of biological aerosols have been proposed, such as laser-induced fluorescence (LIF) (Choi et al. Citation2017; Zhang et al. Citation2013), field-effect transistor (Kim et al. Citation2016), or quartz crystal microbalance (QCM; Recognition of biological signals in dust by antibody functionalized surfaces) devices (Morris et al. Citation2014). Among them, LIF is the most widely used, because it can achieve real-time bioaerosol detection. Microorganisms under ultraviolet radiation, organic substances in the body (Fennelly et al. Citation2017). However, the LIF method requires a high concentration of laser irradiation in order to obtain a readable and reliable bioaerosol signal. QCM-based bioaerosol detection, interference from co-existing abiotic matter (fluorescence or non-particulate matter (PM)) may lead to an underestimate or overestimate of bioaerosol concentration, so extra calibration is needed to achieve reasonable data reliability (i.e., distinguish biological and non-biological particles)(Morris et al. Citation2014).
A lot of different biosensing technologies have emerged to identify bioaerosols in the atmosphere in recent years, and typical immune sensors include immune sensing components and sensors. The principle of immunosensor is that antigen or antibody is fixed on some materials, and then combined with the object to be detected, and the signal generated by the combination will be converted into electrical signal, which will be detected by the sensor (Marco, Gee, and Hammock Citation1995). In addition to immunosensing, a number of non-immunosensing methods have flourished in recent years, including electrochemical biosensors (Yoo et al. Citation2017), label-free bioaerosol sensing devices (Wu et al. Citation2018a), biosensor based on particle aggregation (Wu et al. Citation2018b), ATP-based bioluminescence sensors (Han et al. Citation2015a), fluorescence sensors (Ryškevič et al. Citation2010), a field-effect transistor and electro-aerodynamic deposition (Park et al. Citation2015b), and micro-aerosol sensor (Zhang, Zhu, and Yang Citation2016). These nonimmune sensing methods have been applied to detect biological aerosols in various fields.
Using Klarite as a substrate, Surface-enhanced Raman spectroscopy (SERS) can be an ultra-sensitive and rapid detection technique for atmospheric bioaerosols. Tahir (Tahir et al. Citation2019) found that live Escherichia coli could generate strong SERS signal, while dead airborne bacteria did not. The Klarite interface based on SERS can not only detect the bacteria in the air qualitatively, but also quantitatively distinguish the dead bacteria in the air with different proportions. This method made it possible to count single living and dead bacteria using SERS mapping, which represented the rapid, culture-free and tag-free detection of bacteria in the air in the real environment.
To solve the misreading in bioaerosol detection, a device which can analyze small biomolecules based on amplification of specific DeoxyriboNucleic Acid(DNA) region was developed and capable of providing results within 90 minutes. Indirect prediction based on PM detection data is also considered to be an inexpensive, simple and fast solution (Tovena Pecault et al. Citation2017). However, Polymerase Chain Reaction(PCR) method is still too slow to complete the rapid detection of biological aerosol, and the prediction method may lead to significant uncertainty, which does not support bioanalysis. In addition, both these strategies and LIF analysis require trained operators to perform detection or data processing (Choi et al. Citation2017; Swanson Citation2018). Mbareche et al. (Citation2018). summarized the potential of molecular-based methods (such as detecting biogenetic substances in a given sample) for sampling biological aerosols, and reached the conclusion that technical details (such as cell separation or concentration) should be considered. Therefore, in order to measure culturable rapidly and reliably and non-culturable bioaerosols while avoiding overestimation or underestimation, an achievable and easy-to-use detection platform is urgently needed.
Although several studies have described the technology of tracking bioaerosol particles released from different sources in the environment, they are short of accuracy, rapidity and simplicity (Xie et al. Citation2021). Under this background, this paper summarizes the latest progress in the research of ATP bioluminescence, which is a method for quantifying bioaerosols by measuring ATP content through bioluminescence.
Fundamentals of ATP-based bioluminescence in bioaerosol detection
Lyon’ s Dubva thought bioluminescence was the result of chemical reaction, and proposed the concept of fluorescein and luciferase. In 1914, Harvey of Princeton University published his first paper ‘ Chemical Properties of Firefly Luminescent Materials” in the journal Science (Harvey Citation1914). In 1916, he began to use the word “bioluminescence”. Harvey is considered the father of modern bioluminescence. In fact, many important discoveries in the field of bioluminescence in the 20th century were contributed by Harvey, Harvey’ s students or Harvey’ s collaborators. In 1928, Harvey and Stevens studied the bioluminescence of western Indian tortoise shells (Harvey and Stevens Citation1928). In 1947, ATP bioluminescence was pioneered by W. D. McElroy and colleagues in attempt to detect microorganisms (McElroy Citation1963). All bioluminescence processes include six basic stages: fluorescein oxidation to peroxide, peroxide decomposition to excitation product, luminescence, fluorescein regeneration, fluorescein storage and fluorescein release. For luminescent protein bioluminescence, fluorescein oxidation first forms a stable fluorescein-O-O-luciferase complex, followed by peroxide, which is usually initiated by metal cations. Notably, these six phases depend heavily on specific bioluminescent systems (Li, Chang, and Wu Citation2010).
In the past 20 years of the 21st century, we have clearly seen the great development of bioluminescence. The discovery and application of green fluorescent protein were awarded the 2008 Nobel Prize in Chemistry. Scientists have synthesized volatile firefly oxyluciferin(oxyLH2) and studied the crystal structures of several important luciferase/luminescent proteins. They tried to understand the factors affecting the luminescence wavelength and luminescence efficiency and modified the molecular structures of luciferase and mutant luciferase to regulate the color of bioluminescence. These studies focus not only on the spectra previously studied, but also on the mechanisms and applications of bioluminescence.
In the analysis of bioaerosol samples, ATP in bioaerosol is released after cleavage, which reacts with appropriate enzymes to produce bioluminescence, which is directly proportional to the concentration of bioaerosol (Karl Citation1980a). Lin et al. (Citation2013) has developed an ATP bioluminescence method for indoor bioaerosol sensing. They concluded that ATP bioluminescence was highly correlated with the overall CFU count (r = 0.963, p value < .001). Therefore, this method can be used as an index of the total load of biological aerosol, and can be effectively applied in field or field research (Han et al. Citation2015b; Lin et al. Citation2013), while many previous studies (Han et al. Citation2015a; Lin et al. Citation2013; Park et al. Citation2014; Yoon et al. Citation2010) have verified the feasibility of ATP bioluminescence for rapid bioaerosol detection.
At present, ATP bioluminescence technology has become a rapid quantitative method for biological aerosol widely applied to various atmospheric environments. The experiment used ATP, which acts as a carrier of energy and plays a central role in microbial cells, linking catabolism and biosynthesis (Cordeiro et al. Citation2017). In ATP test, under the catalysis of firefly luciferase, fluorescein was excited, and then emitted photons and returned to the ground state (Marques and Silva Citation2008). The peak intensity of the photons was within 500 nm (Karl Citation1980b). Microbial biomass can be quantified by measuring the ATP content using bioluminescence for the intensity of the generated ATP bioluminescence is proportional to the ATP content within the bioaerosol (Okanojo et al. Citation2017). Since the introduction of ATP bioluminescence in 196329, most ATP bioluminescence technologies have continued to be used for rapid detection of microorganisms and possibly for the warning and detection of biological warfare attacks. There are many quantitative methods for bioaerosol, but ATP bioluminescence has become the preferred detection tool due to its simplicity, rapidity and convenience (Cordeiro et al. Citation2017). In addition, since most viable microorganisms use ATP molecules as a necessary source of energy, it can be used as a basis for effectively quantifying microorganisms. The production of ATP bioluminescence is as follows:
For several reasons, ATP bioluminescence is very suitable for the quantification of bioaerosols. First, all biologically active organisms require ATP for maintaining life activities. The ATP assay detects all metabolically active cells, indicating microbial viability. Although this method cannot distinguish between specific species of microorganisms, but it can represent the biological aerosol physiological conditions. Second, direct detection of ATP can avoid abiotic disturbance, for ATP is generally not exist in abiotic aerosols (Lomakina, Modestova, and Ugarova Citation2015). Hence, at concentrations normally present in bioaerosols in the atmosphere, there is no interference with ATP measurements results from abiotic aerosols, which ensured the reliability and the accuracy of detecting the bioaerosols. Third, using ATP bioluminescence to detect biological aerosol can be combined with many modern new technologies to realize rapid and reliable quantification of bioaerosols. Basic steps of bioaerosols detection using ATP bioluminescence are as follows: sampling and enriching artificial or atmospheric bioaerosols, followed by the microorganisms lysis with lyase so that ATP can be released, and then ATP fluorescence assay is performed. Hence, bioaerosols can be detected by ATP bioluminescence assay, which also allows districting bioaerosols from non-biological aerosols.
If the signal interference from co-existing non-biological particles is properly eliminated, failure to detect bioaerosols by ATP bioluminescence is most often caused by low sample content based on the trace existence of bioaerosols in the atmosphere, high detection limit or long process times. In this review, we will discuss the progress made in recent years in these three areas.
Effect of sampling method on detection efficiency
The effects of different bioaerosol sampling methods on bioaerosol culture ability and cell wall integrity were different (Zhen et al. Citation2013). The selection of sampling methods will also affect the intensity of ATP bioluminescence signal and the accuracy of bioaerosol analysis, because the metabolic activity of microorganisms affects the intensity of ATP fluorescence. Sampling prior to testing is essential for the quantification of ATP bioluminescence.
At present, the impact method of solid medium is recognized as the most ideal sampling method for air microorganisms (Kuo Citation2015). Six-stage Andersen cascade impactor is a common method to measure the concentration of bioaerosol (Kim et al. Citation2011). In this method, microorganisms can be captured with high precision by multi-stage impact sampler. Each stage uses multi-nozzle plate and a culture dish filled with an agar culture medium as impact plates, and bacteria can be collected directly on the agar plates. The microorganisms in the air are classified according to particle size, which has achieved good results in the detection of air microorganisms (Lindsley et al. Citation2017). However, some inherent problems of Andersen impactor may lead to inaccurate counting: First, sensitive microorganisms may produce significant mechanical stress due to inertial impact, thus underestimating biological aerosol (Lindsley et al. Citation2017). Secondly, the flow rate of 28.3 L/min in a high concentration environment may lead to overlapping of bacteria on the agar plate, resulting in inaccurate counting. Generally, it is only used for sampling culture, and it is difficult to combine with other liquid detection methods such as ATP fluorescence in actual detection. However, this method can be used as a “gold standard” for comparison with other detection methods.
For low costs and easy handling, impingement are economically practicable samplers. The fundamentals behind impingement is the collection of atmosphere microorganism and particles into a liquid medium. The inlet of the sampler will have a certain influence on the efficiency of sampling and recovery, especially the wind speed at the inlet and the direction of the sampler (Li Citation2010). At present, the commonly used sampler is “the “Bio sampler” liquid impactor (SKC, 8, four, PA, USA) (). The biological sampler is an all-glass rotating aerosol collector, which consists of an inlet hole, three nozzles and a collection container (Li Citation2010). The sampler also includes a pump, which can suck air at the same speed as AGI-30, but the sampling time is up to 0.5 ~ 4 hours. The strength of this sampler is that it can be used in highly polluted environment. The sampling time can reach 30 minutes in aqueous sampling medium and several hours in viscous sampling medium.
There are many new bioaerosol samplers such as NIOSH one-stage Bioaerosol Cyclone, CIP 10-M, NIOSH two-stage cyclone, Coriolis®, WWC, and PAS-5 by RCT & HRB were developed. In a cyclone sampler, microorganisms are captured into liquids (from aerosols to pure sols) using rotating air and centrifugal force (Willeke, Lin, and Grinshpun Citation1998). The sterilization of these samplers are relatively easy, which is especially important when multiple samples need to be collected (Cartwright and Kirton Citation2009). Since the sampling medium of both cyclones and impactors is water, the efficiency of the cyclones is studied. The results show that the recovery rate of gram-negative bacteria in cyclones is 100 ± 10% relative to AGI-30 impactors (Han et al. Citation2011).
Filter-based samplers are a indeed effective way to collect bioaerosols, which are not only simple but also cheap. Filtration is the collection of airborne microorganisms through porous membrane filters made from fiberglass, polyvinyl chloride (PVC), polycarbonate, or cellulose acetate (cultured by transferring to the surface of AGAR culture medium for growth), or gelatin (Ghosh, Lal, and Srivastava Citation2015). However, as with the impact sampler, the count of bioaerosols becomes difficult when the sampler in the filter is used in a highly contaminated environment because the filter with microorganisms is overloaded (Ghosh, Lal, and Srivastava Citation2015). In fact, the main factors determining the loss caused by drying are sampling time and relative humidity. When the filter rotates, it helps the bacterial cells recover (Robinson Citation2012).
Because the pressure becomes smaller, electrostatic sampling is a good alternative to bioaerosol sampling, with much less morphological damage than using impactor samples. Kim’s lab has made an electrostatic rod-type sampler (Kim et al. Citation2018). The concentration of bacteria cultured with a laboratory – made electrostatic rod sampler was 5.2 times higher than that cultured with a single-stage viable impactor (Park et al. Citation2015a).
Inspissation when sampling air for bacteria improves detection of low concentrations of fungi in air in various environments. Han et al. (Citation2011) developed a super hydrophobic surface electrostatic precipitator (EPSS), which can concentrate fungi in the air in small amounts of liquid (10 or 40 μl) (). At lower spore loads, the collection efficiency is significantly improved, and the concentration of spores in the air typically encountered in the environment is >60% i.e., 104 ~ 105 spores/m3, allowing very high concentrations to be achieved.
Subsequently, Han T. developed and tested an ATP-based bioluminescence protocol for rapid characterization and collection of bacterial aerosols in liquids (Han et al. Citation2015a). The Bacillus atrophaeus and Pseudomonas fluorescens were nebulized with a Collison nebulizer (BGI Inc., Waltham, MA) in a glass or polycarbonate canister and collected for 15 and 60 minutes: (1) push-button aerosol sampler(SKC Inc., Eighty F, PA) with polycarbonate, PTFE, and nitrocellulose filters, (2) biological sampler (SKC Inc.), with 5 and 20 ml of collection solution, (3) newly developed Electrostatic Precipitator with EPSS (Han et al. Citation2011). The total number of bacteria observed under fluorescence microscope was directly counted by acridine orange, and the relative luminous unit (RLU) of total ATP content was plotted. The collection efficiencies of the two bacteria determined by ATP at all flow rates were consistent (within 5%) with the acridine orange direct count. Therefore, ATP-based techniques can reliably serve as a tool for rapid and detailed evaluation of bioaerosol sampling devices. The total number of bacteria observed by fluorescence microscope was directly counted by acridine orange, and the relative luminous unit (RLUs) of total ATP content was plotted. The collection efficiency of the two bacteria measured by ATP method at all flow rates is in good agreement with the direct counting of acridine orange (within 5%). Therefore, ATP-based technology can be reliably used as a tool for rapid and detailed evaluation of biological aerosol sampling devices.
Recently, details of a bioaerosol sampler that incorporates ATP bioluminescence have been unveiled to allow for faster and easier measurement of bioaerosol concentrations (Liao, Byeon, and Park Citation2021). They developed ATP bioaerosol samplers and made three jet impactors, which were tested with NaCl particles. The experimentally measured d50 is 0.44 μm, because most bioaerosols are larger than d50. The concentration of nebulized E. coli collected using the sampler was highly correlated to the concentration collected using the Anderson impactor in laboratory tests (R2 = 0.85). These findings provide a small-scale device for measuring bioaerosol exposure and an easy-to-use methodological concept for effective air quality management. The schematic diagram of detection is shown in the .
Improving the sensitivity of ATP bioluminescence
In order to improve the sensitivity, a variety of methods (Karl Citation1980b; Satoh et al. Citation2014) have been developed to increase ATP bioluminescence by converting ADP or AMP into ATP enzyme (), which significantly improves the sensitivity of ATP bioluminescence.
The use of adenylate kinase (ADK) and pyruvate kinase for ATP amplification has the potential to detect very low levels of ATP without the use of photon detectors. Lee et al. (Citation2017) designed ATP amplification using (i) ADK as the first enzyme that converts AMP+ATP to two ADP molecules, and (ii) polyP kinase (PPK) as the second enzyme that converts ADP to ATP using polyP. In this reaction, excess AMP and polyP are added to the reaction mixture, which drives the ADK and PPK equilibriums to ADP and ATP formation, respectively. The amplified ATP is subjected to bioluminescence detection in a firefly luciferase reaction. The sensitivity of this method to ATP was about 10,000 times that of the bioluminescence method without ATP amplification. The ATP is amplified before the bioluminescence detection, and the luminescence amount can be improved without signal integration, thereby greatly improving the sensitivity of the bioluminescence detection to ATP.
To measure attomol‐level ATP in microliter-sequenced samples, ATP contamination by processing reagents must be removed and the ATP detection limit increased. An ATP automatic bioluminescence device was developed, and the detection limit was 0.8 amol (Okanojo et al. Citation2017). The ATP bioluminescence instrument is capable of measuring multiple highly sensitive samples by automatically minimizing the amount of carryover on the nozzle (Shah and Naseby Citation2015). A 3.3 SD value for background signal intensity corresponds to 0.7 amol of ATP bioluminescence. Since the developed ATP bioluminescence instrument can automatically distribute samples and measure ATP bioluminescence, which intensity peak is easy to detect and free from contamination and carrying. The ATP content of E. coli and S. aureus was determined by ATP bioluminescence instrument. These microorganisms were detectable at ATP levels >0.8 amol.
An increasingly popular alternative to increasing the bioluminescence sensitivity of ATP is the thermal treatment of bacterial cells to increase the release of ATP. For even greater sensitivity, (Bakke and Suzuki Citation2018) describes a method of increasing the amount of ATP released from bacterial cells by heat treating a sample to improve sensitivity. The RLU was increased several times when the samples were treated with different concentrations of phosphate buffered saline (PBS) containing E. coli O157:H7, Salmonella enteritidis, Bacillus cereus etc. at 25°C-95°C for 10 min. Due to signal enhancement, the limit of detection (LOD) of ATP bioluminescence in milk-containing microorganisms was increased by approximately one order of magnitude. The results showed that a simple heating step of the food sample before measurement helped to increase the sensitivity of the ATP bioluminescence assay for bacteria. This method requires only a few minutes of heat treatment at a moderate temperature of about 60°C.The sensitivity of the ATP bioluminescence assay was increased by a factor of 10 without a significant increase in the time required for detection.However, since some microorganisms were easily killed (such as psychrophilic bacteria) at 60°C, the method still required careful optimization and cross-checking in each experiment.
In recent years, an advanced sensing system that uses air ions to destroy cell membranes has been coupled with ATP bioluminescence (Park et al. Citation2014). Firstly, in bacterial cells, ATP is extracted by a carbon fiber ionizer and produces ATP bioluminescence in the sensor. Then, the experimental tests of Staphylococcus epidermidis and Escherichia coli were carried out by atomizing atomizer, and similarly the presence of indoor biological aerosol was checked. The method also provides a solution for rapid monitoring of bioaerosols (e.g., cell lysis time shorter than 4 minutes plus bioluminescence detection)(Park, Kim, and Hwang Citation2016).
Real-time and continuous bioaerosol-sensing system
In all of the above studies, ATP bioluminescence detection takes 4 min~3 h, which is a significant improvement on the traditional colony counting method. However, these studies also inevitably require time for manual operations such as sample preparation. In order to avoid the need of manual intervention, (Park, Kim, and Hwang Citation2016) studied a continuous and real-time bioaerosol detection method using an integrated aerosol-pure sol sampler and a bioluminescent detector. He developed an bioaerosol-pure sampler, which is used to capture bacterial particles in the air. The flowing liquid contains cell lysis buffer and ATP bioluminescent reagent and is connected to a bioluminescent detector (). The technology has been successfully proved by bioaerosol-pure joint sampling and ATP bioluminescence test: the device can realize that real-time detection of the concentration of the bioaerosols and provide reliable data. However, environmental conditions such as indoor temperature, biological aerosol species and humidity may affect the correlation between biological aerosol RLU and CFU concentration. Further experiments are required under different environmental conditions.
Improving detection speed and reliability is the key to bioaerosol detection. Recently, a fast and reliable integrated bioaerosol detection platform has been developed, which combines the supply of dissolved droplets with PM sampling cotton swabs (Kim et al. Citation2019). The Arduino-Bluetooth smart phone controller is connected to the user interface program, and the dissolved swab sampler is used for convenient testing and data analysis (). The analysis provides a compact digital bioaerosol detection platform and an easy-to-use method for effective air quality monitoring. Real-time monitoring of bioaerosol concentration and composition can be accomplished.
Figure 6. Schematic of simultaneous lysis droplet supply (for the last 30s of sampling) via an automatic vibrating nozzle under the control of an Arduino–Bluetooth smartphone system during air sampling onto a swab.
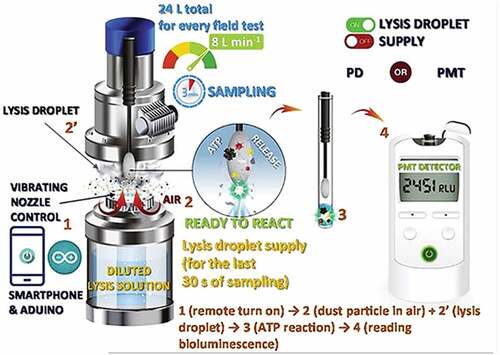
With the decrease of enzyme activity in field operation, the reaction stability of enzyme itself becomes worse (Nguyen and Kim Citation2017). The dosage of luciferase and other reagents needs to be controlled by additional devices. Therefore, (Cho et al. Citation2020) creatively uses immobilized luciferase containing basic components such as fluorescein and magnesium, so that efficient bioluminescence reaction can be carried out without injecting liquid into the reaction group in the field system. And he developed a field automated bioaerosol-detecting system (ABMS). After all the units are integrated, ABMS realizes the detection of E. coli bioaerosol, which is detected continuously every 5 min, with the lowest detection limit of ~130 CFU/m3. This is the first attempt to use the immobilized components such as luciferase and fluorescein in ATP bioluminescence reaction for real-time bioaerosol monitoring.
In summary, we believe that the detection of bioaerosols using ATP bioluminescence technology is still an imperfect technology. Known limitations of current detection must be taken into account, and attempts must be made to increase the sensitivity of ATP bioluminescence. On the other hand, to realize the actual application of the system, more experiments and statistical research are needed under different detection conditions.
Applications of the bioaerosols detection by ATP bioluminescence
The applications of ATP bioluminescence are numerous, so in the next section we will only mention a few recent applications.
ATP bioluminescence technology has been widely used in biological medicine, food, environmental system. At present, ATP bioluminescence technology has been used to measure the biological removal efficiency in air, such as air quality control in composting facilities and production places (Brooks and Gerba Citation2014; Kováts and Horváth Citation2016; Park et al. Citation2011; Watanabe et al. Citation2018). At the refuse dump, odor, air particles and bioaerosols are released from the waste decomposition process; Therefore, air quality control of the composting facility is important for the health of the composting workers and the efficient operation of the composting facility. To determine the biological removal efficiency of airborne particles, Park used a laboratory-made inertial impactor sampler and a commercial portable ATP bioluminescence detector.
In hospitals, especially during dental treatment, sprays and splashes are potential sources of infection. ATP bioluminescence analysis was used to investigate the pollution of masks, goggles and chest of operators before and after treatment. The contamination of each surface increased after the dental treatment and patients’ goggles are the most polluted. Therefore, ATP bioluminescence is an effective tool for detecting surface contamination generated during dental treatment to avoid disease transmission.
In summary, as pathogens in the air may attack people’ s bodies without their knowledge, the new, more sensitive ATP bioluminescence assays to detect bioaerosols have the greatest impact on environmental detection.
Combining ATP bioluminescence with new techniques
In recent years, ATP bioluminescence technology has been combined with a variety of other methods to conduct quantitative research on biological aerosol according to different requirements under different conditions.
The ATP bioluminescence detection system can be combined with a carbon fiber ionizer, so that the concentration of the biological aerosol can be rapidly detected (Park et al. Citation2014), which has short detection time, and is an effective, economical and feasible solution for rapid detection of biological aerosols.
A novel biosensor extracts ATP from bacterial cells through a microfluidic channel and using an aerosol condensation system to quantitatively determines ATP in bioaerosols. Santangelo et al. (Citation2018) reports the design, fabrication and testing of 3D printing microfluidic chips coupled with silicon photomultiplier tubes (SiPMs) for high sensitivity real-time ATP detection. The 3D microfluidic chip reduces the consumption of reactants, facilitates the delivery of solutions close to SiPM, and improves the detection efficiency. The LOD of ATP detected by their system is 8 nm, the analysis dynamic range is between 15 nm and 1 μ m, the stability error is 3%, and the repeatability error is less than 20%. And (Zhang et al. Citation2017) proposed a new biochemical system prototype, which uses nano probes related to ATP technology to count E. coli colonies. Its novelty lies in that magnetic nanoparticle beads are modified with specific antibodies and used as probes to capture and enrich Escherichia coli through the specific connection between antigen and antibody. Within 20 minutes, the detectable concentration of bacteria ranged from 102 CFU/ml to 108 CFU/ml. Under the best test conditions, LOD is only 3.0 × 102 CFU/mL. Independent experimental data show that the maximum fluctuation of signal-to-noise ratio of the prototype is less than 6.98%, which has good reliability and repeatability.
The combination of these new techniques with ATP fluorescence for the detection of aerosols in the atmosphere will greatly improve the detection efficiency of bioaerosols in the atmosphere and the sensitivity of ATP fluorescence technique.
Conclusion
Biological aerosols are ubiquitous in closed environments. In addition, due to the diversity of potential health effects of bioaerosols, quantification of bioaerosols is important. In the absence of detailed sampling and enumeration techniques, assessment of exposure levels is very hard. ATP-based bioluminescence has the potential to provide a rapid and economical method for bioaerosol sample quantification. With the new development of ATP bioluminescence technology, bioaerosols can be quantified in almost all natural environments, which not only improve the efficiency of bioaerosol detection, but also make it possible that many emerging technologies can be combined with bioaerosol detection. In addition, it can be seen from this review that different methods have different limitations, so different technologies can also be combined for research to overcome their limitations.
At present, in view of the low sensitivity level of ATP bioluminescence detection method, this method still needs a large amount of microorganisms to obtain sufficient detection signals. Therefore, for some specific environments with relatively low background levels of air microorganisms, the combination of this method with large flow sampling technology with high sample enrichment ability is mainly considered in the follow-up to better realize the detection of low concentration bioaerosol.
In addition, we can also combine different enrichment methods with ATP fluorescence to optimize the enrichment method which is more suitable for broad-spectrum enrichment of bioaerosols from the aspects of capture efficiency and separation speed, and realize the effective integration of sampling, enrichment and detection by combining sampling, microfluidic circuit and magnetic separation technology to further improve the detection efficiency. For the detection of specific biological aerosols, specific recognition elements can be used to modify magnetic particles to achieve specific enrichment and ATP fluorescence detection.
Disclosure statement
No potential conflict of interest was reported by the author(s).
Data availability statement
The data that support the findings of this study are available from the corresponding author upon reasonable request.
Additional information
Funding
Notes on contributors
Yueqi Zhang
Yueqi Zhang is a master's student in the State Key Laboratory of NBC Protection for Civilian. Academy of Military Sciences. She began her research in 2019. Her current research focuses on bioaerosol monitoring.
Bing Liu
Bing Liu (corresponding author) is an associate professor in the State Key Laboratory of NBC Protection for Civilian. His Ph.D. degree received in applied chemistry in 2013. His current research focuses on the areas of chemical sensing, biosensing and bioaerosol monitoring.
Zhaoyang Tong
Zhaoyang Tong is a professor in the State Key Laboratory of NBC Protection for Civilian. His Ph.D. degree received in applied chemistry in 2000. His current research focused on biochemistry, nanotechnology, chemical and biological sensing, applications of optical, electrochemical and biochemical sensors for environmental, medical, biological and chemical monitoring.
References
- Bakke, M., and S. Suzuki. 2018. Development of a novel hygiene monitoring system based on the detection of total adenylate (ATP+ADP+AMP). J. Food Prot. 81 (5):729–37. doi:10.4315/0362-028X.JFP-17-432.
- Brooks, J. P., and C. Gerba. 2014. Bioaerosol contamination of produce: Potential issues from an unexplored contaminant route. In The produce contamination problem: Causes and solutions: Second edition, 107–21. Academic Press.
- Byeon, J. H., C. W. Park, K. Y. Yoon, J. H. Park, and J. Hwang. 2008. Size distributions of total airborne particles and bioaerosols in a municipal composting facility. Bioresour. Technol. 99 (11):5150–54. doi:10.1016/j.biortech.2007.09.014.
- Camatini, M., V. Corvaja, E. Pezzolato, P. Mantecca, and M. Gualtieri. 2012. PM10-biogenic fraction drives the seasonal variation of proinflammatory response in A549 cells. Environ. Toxicol. 27 (2):63–73. doi:10.1002/tox.20611.
- Cartwright, C. H. S., and J. Kirton. 2009. Review of methods to measure bioaerosols from composting sites. Environ. Agency. SC040021/SR3:20–8.
- Cho, Y. S., H. R. Kim, H. S. Ko, S. B. Jeong, B. Chan Kim, and J. H. Jung. 2020. Continuous surveillance of bioaerosols on-site using an automated bioaerosol-monitoring system. ACS Sens. 5 (2):395–403. doi:10.1021/acssensors.9b02001.
- Choi, J., J. S. Kang, S. C. Hong, G.-N. Bae, and J. H. Jung. 2017. A new method for the real-time quantification of airborne biological particles using a coupled inertial aerosol system with in situ fluorescence imaging. Sens. Actuators B Chem. 244:635–41. doi:10.1016/j.snb.2017.01.055.
- Cordeiro, A. C., J. L. Fabris, G. H. Couto, H. J. Kalinowski, and E. Bertogna. 2017. Water assessment using ultra-weak bioluminescence. J. Photochem. Photobiol. B, Biol. 177:39–43. doi:10.1016/j.jphotobiol.2017.10.014.
- Fennelly, M., G. Sewell, M. Prentice, D. O’Connor, and J. Sodeau. 2017. Review: The use of real-time fluorescence instrumentation to monitor ambient primary biological aerosol particles (PBAP). Atmos. 9 (1):1. doi:10.3390/atmos9010001.
- Ghosh, B., H. Lal, and A. Srivastava. 2015. Review of bioaerosols in indoor environment with special reference to sampling, analysis and control mechanisms. Environ. Int. 85:254–72. doi:10.1016/j.envint.2015.09.018.
- Górny, R, D. J., and E. Krysińska-Traczyk. 1999. Size distribution of bacterial and fungal bioaerosols in indoor air. Ann. Agric. Environ. Med. 6 (2):105–13.
- Han, T., Y. Nazarenko, P. J. Lioy, and G. Mainelis. 2011. Collection efficiencies of an electrostatic sampler with superhydrophobic surface for fungal bioaerosols. Indoor Air 21 (2):110–20. doi:10.1111/j.1600-0668.2010.00685.x.
- Han, T., M. Wren, K. DuBois, J. Therkorn, and G. Mainelis. 2015a. Application of ATP-based bioluminescence for bioaerosol quantification: Effect of sampling method. J. Aerosol. Sci. 90:114–23. doi:10.1016/j.jaerosci.2015.08.003.
- Han, T., H. Zhen, D. E. Fennell, and G. Mainelis. 2015b. Design and Evaluation Of The Field-Deployable Electrostatic Precipitator With Superhydrophobic Surface (FDEPSS) with high concentration rate. Aerosol Air Qual. Res. 15 (6):2397–408.
- Harvey, E. N. 1914. On the chemical nature of the luminous material of the firefly. Sci. (New York, N.Y.) 40 (1018):33–34. doi:10.1126/science.40.1018.33.
- Harvey, E. N., and K. P. Stevens. 1928. The brightness of the light of the west Indian elaterid beetle, pyrophorus. J. Gen. Physiol. 12 (2):269–72. doi:10.1085/jgp.12.2.269.
- Karl, D. M. 1980a. Cellular nucleotide measurements and applications in microbial ecology. Microbiol. Rev. 44 (4):739.
- Karl, D. M. 1980b. Cellular nucleotide measurements and applications in microbial ecology. Microbiol. Rev. 44 (4):739–96. doi:10.1128/mr.44.4.739-796.1980.
- Kim, H. R., S. An, J. Hwang, J. H. Park, and J. H. Byeon. 2019. In situ lysis droplet supply to efficiently extract ATP from dust particles for near-real-time bioaerosol monitoring. J. Hazard. Mater. 369:684–90. doi:10.1016/j.jhazmat.2019.02.088.
- Kim, J., J. H. Jin, H. S. Kim, W. Song, S. K. Shin, H. Yi, D. H. Jang, S. Shin, and B. Y. Lee. 2016. Fully automated field-deployable bioaerosol monitoring system using carbon nanotube-based biosensors. Environ. Sci. Technol. 50 (10):5163–71. doi:10.1021/acs.est.5b06361.
- Kim, K. H., E. Kabir, and S. A. Jahan. 2018. Airborne bioaerosols and their impact on human health. J. Environ. Sci. (China) 67:23–35. doi:10.1016/j.jes.2017.08.027.
- Kim, S. Y., Z. Y. Kim, S. Lee, and G. Ko. 2011. Comparison of molecular and total ATP-based analytical methods with culture for the analysis of bioaerosols. Sci. Total Environ. 409 (9):1732–37. doi:10.1016/j.scitotenv.2011.01.035.
- Kim, H. R., J.-W. Park, H. S. Kim, D. Yong, and J. Hwang. 2018. Comparison of lab-made electrostatic rod-type sampler with single stage viable impactor for identification of indoor airborne bacteria. J. Aerosol. Sci. 115:190–97. doi:10.1016/j.jaerosci.2017.11.002.
- Kováts, N., and E. Horváth. 2016. Bioluminescence-based assays for assessing eco- and genotoxicity of airborne emissions. Lumin. 31 (4):918–23. doi:10.1002/bio.3102.
- Kuo, Y.-M. 2015. Field evaluation of sampling bias with plastic petri dishes for size-fractionated bioaerosol sampling. Aerosol Sci. Technol. 49 (3):127–33. doi:10.1080/02786826.2015.1009530.
- Lee, J., C. Park, Y. Kim, and S. Park. 2017. Signal enhancement in ATP bioluminescence to detect bacterial pathogens via heat treatment. BioChip J. 11 (4):287–93. doi:10.1007/s13206-017-1404-8.
- Li, C.-S. 2010. Evaluation of microbial samplers for bacterial microorganisms. Aerosol Sci. Technol. 30 (2):100–08. doi:10.1080/027868299304705.
- Li, L., C. Chang, and J. Wu. 2010. Research progress in the ATP bioluminescence assay and its applications. Sci. Technol. Food Ind. 31 (9):394–97.
- Liao, L., J. H. Byeon, and J. H. Park. 2021. Development of a size-selective sampler combined with an adenosine triphosphate bioluminescence assay for the rapid measurement of bioaerosols. Environ. Res. 194:110615. doi:10.1016/j.envres.2020.110615.
- Lin, C.-J., Y.-T. Wang, K.-J. Hsien, Y. I. Tsai, P.-Y. Kung, and J.-M. Chyan. 2013. In situ rapid evaluation of indoor bioaerosols using an ATP bioluminescence assay. Aerosol. Air. Qual. Res. 13 (3):922–31. doi:10.4209/aaqr.2013.01.0009.
- Lindsley, W. G., B. J. Green, F. M. Blachere, S. B. Martin, and M. P. Schafer. 2017. Sampling and characterization of bioaerosols. In NIOSH manual of analytical method. 5th ed., BA1-115.
- Lomakina, G. Y., Y. A. Modestova, and N. N. Ugarova. 2015. Bioluminescence assay for cell viability. Biochem. Mosc. 80 (6):701–13. doi:10.1134/S0006297915060061.
- Marco, M. P., S. Gee, and B. Hammock. 1995. Immunochemical techniques for environmental analysis II. Antibody production and immunoassay development. TrAC. Trends. Anal. Chem. 14 (9):415–25. doi:10.1016/0165-9936(95)90920-I.
- Marcovecchio, F., and C. Perrino. 2021. Contribution of primary biological aerosol particles to airborne particulate matter in indoor and outdoor environments. Chemosphere 264 (Pt 2):128510. doi:10.1016/j.chemosphere.2020.128510.
- Marques, S., and J. Silva. 2008. Firefly bioluminescence: A mechanistic approach of luciferase catalyzed reactions. IUBMB Life 61 (1):6–17. doi:10.1002/iub.134.
- Mbareche, H., M. Veillette, G. J. Bilodeau, and C. Duchaine. 2018. Bioaerosol sampler choice should consider efficiency and ability of samplers to cover microbial diversity. Appl. Environ. Microbiol. 84 (23). doi:10.1128/AEM.01589-18.
- McElroy, W. D. 1963. Crystalline firefly luciferase: LH2 + ATP ⇄ LH2-AMP + PP LH-2-AMP + O2 → L-AMP + light + H2O. Meth. Enzymol. 6:445–48. Academic Press.
- Meklin, T., T. Reponen, M. Toivola, V. Koponen, T. Husman, A. Hyvrinen, and A. Nevalainen. 2002. Size distributions of airborne microbes in moisture-damaged and reference school buildings of two construction types. Atmos. Environ. 36 (39):6031–39. doi:10.1016/S1352-2310(02)00769-0.
- Monn, C. 2001. Exposure assessment of air pollutants: A review on spatial heterogeneity and indoor/outdoor/personal exposure to suspended particulate matter, nitrogen dioxide and ozone. Atmos. Environ. 35 (1):1–32. doi:10.1016/S1352-2310(00)00330-7.
- Morris, D. R. P., J. Fatisson, A. L. J. Olsson, N. Tufenkji, and A. R. Ferro. 2014. Real-time monitoring of airborne cat allergen using a QCM-based immunosensor. Sens. Actuators B Chem. 190:851–57. doi:10.1016/j.snb.2013.09.061.
- Nguyen, H. H., and M. Kim. 2017. An overview of techniques in enzyme immobilization. Appl. Sci. Convergence Technol. 26 (6):157–63. doi:10.5757/ASCT.2017.26.6.157.
- Okanojo, M., N. Miyashita, A. Tazaki, H. Tada, F. Hamazoto, M. Hisamatsu, and H. Noda. 2017. Attomol-level ATP bioluminometer for detecting single bacterium. Lumin. 32 (5):751–56. doi:10.1002/bio.3246.
- Okten, S., and A. Asan. 2012. Airborne fungi and bacteria in indoor and outdoor environment of the pediatric unit of Edirne government hospital. Environ. Monit. Assess. 184 (3):1739–51. doi:10.1007/s10661-011-2075-x.
- Park, C. W., J. H. Byeon, K. Y. Yoon, J. H. Park, and J. Hwang. 2011. Simultaneous removal of odors, airborne particles, and bioaerosols in a municipal composting facility by dielectric barrier discharge. Sep. Purif. Technol. 77 (1):87–93. doi:10.1016/j.seppur.2010.11.024.
- Park, K. T., D. G. Cho, J. W. Park, S. Hong, and J. Hwang. 2015b. Detection of airborne viruses using electro-aerodynamic deposition and a field-effect transistor. Sci. Rep. 5 (1):17462. doi:10.1038/srep17462.
- Park, J. W., H. R. Kim, and J. Hwang. 2016. Continuous and real-time bioaerosol monitoring by combined aerosol-to-hydrosol sampling and ATP bioluminescence assay. Anal. Chim. Acta 941:101–07. doi:10.1016/j.aca.2016.08.039.
- Park, C. W., J. W. Park, S. H. Lee, and J. Hwang. 2014. Real-time monitoring of bioaerosols via cell-lysis by air ion and ATP bioluminescence detection. Biosens. Bioelectron. 52:379–83. doi:10.1016/j.bios.2013.09.015.
- Park, J. W., C. W. Park, S. H. Lee, and J. Hwang. 2015a. Fast monitoring of indoor bioaerosol concentrations with ATP bioluminescence assay using an electrostatic rod-type sampler. PLoS One 10 (5):e0125251. doi:10.1371/journal.pone.0125251.
- Prussin, A. J., 2nd;, E. B.;. Garcia, and L. C. Marr. 2015. Total virus and bacteria concentrations in indoor and outdoor air. Environ. Sci. Technol. Lett. 2 (4):84–88. doi:10.1021/acs.estlett.5b00050.
- Robinson, D. L. 2012. Evaluation of bacterial sampling methods for use with the bacterial tag-encoded flexible (FLX) amplicon pyrosequencing (bTEFAP) technique. Colorado State University. Master of Science (M.S.).
- Ryškevič, N., S. Juršėnas, P. Vitta, E. Bakienė, R. Gaska, and A. Žukauskas. 2010. Concept design of a UV light-emitting diode based fluorescence sensor for real-time bioparticle detection. Sens. Actuators B Chem. 148 (2):371–78. doi:10.1016/j.snb.2010.05.042.
- Santangelo, M. F., S. Libertino, A. P. F. Turner, D. Filippini, and W. C. Mak. 2018. Integrating printed microfluidics with silicon photomultipliers for miniaturised and highly sensitive ATP bioluminescence detection. Biosens. Bioelectron. 99:464–70. doi:10.1016/j.bios.2017.07.055.
- Satoh, T., J. Kato, N. Takiguchi, H. Ohtake, and A. Kuroda. 2014. ATP amplification for ultrasensitive bioluminescence assay: Detection of a single bacterial cell. Biosci. Biotechnol. Biochem. 68 (6):1216–20.
- Shah, N., and D. C. Naseby. 2015. Validation of constitutively expressed bioluminescent Pseudomonas aeruginosa as a rapid microbiological quantification tool. Biosens. Bioelectron. 68:447–53. doi:10.1016/j.bios.2015.01.008.
- Swanson, B. 2018. Development and characterization of an inexpensive single-particle fluorescence spectrometer for bioaerosol monitoring. Opt. Express 26 (3):3646–60. doi:10.1364/OE.26.003646.
- Tahir, M. A., X. Zhang, H. Cheng, D. Xu, Y. Feng, G. Sui, H. Fu, V. K. Valev, L. Zhang, and J. Chen. 2019. Klarite as a label-free SERS-based assay: A promising approach for atmospheric bioaerosol detection. Analyst. 145 (1):277–85. doi:10.1039/C9AN01715A.
- Tovena Pecault, I., M. Thibaudon, J. Clertant, and P. Godefroy. 2017. Macroparticles monitoring for biocontamination prevention. Part. Sci.Technol. 36 (7):908–12. doi:10.1080/02726351.2017.1346021.
- Van Leuken, J. P. G., A. N. Swart, A. H. Havelaar, A. Van Pul, W. Van der Hoek, and D. Heederik. 2016. Atmospheric dispersion modelling of bioaerosols that are pathogenic to humans and livestock - A review to inform risk assessment studies. Microb. Risk Anal. 1:19–39. doi:10.1016/j.mran.2015.07.002.
- Watanabe, A., N. Tamaki, K. Yokota, M. Matsuyama, and S. Kokeguchi. 2018. Use of ATP bioluminescence to survey the spread of aerosol and splatter during dental treatments. J. Hosp. Infect. 99 (3):303–05. doi:10.1016/j.jhin.2018.03.002.
- Willeke, K., X. Lin, and S. A. Grinshpun. 1998. Improved aerosol collection by combined impaction and centrifugal motion. Aerosol Sci. Technol. 28 (5):439–56. doi:10.1080/02786829808965536.
- Wu, Y., A. Calis, Y. Luo, C. Chen, M. Lutton, Y. Rivenson, X. Lin, H. C. Koydemir, Y. Zhang, H. Wang, et al. 2018a. Label-free bioaerosol sensing using mobile microscopy and deep learning. ACS Photonics 5 (11):4617–27. doi:10.1021/acsphotonics.8b01109.
- Wu, Y., A. Ray, Q. Wei, A. Feizi, X. Tong, E. Chen, Y. Luo, and A. Ozcan. 2018b. Deep learning enables high-throughput analysis of particle-aggregation-based biosensors imaged using holography. ACS Photonics 6 (2):294–301. doi:10.1021/acsphotonics.8b01479.
- Xie, W., Y. Li, W. Bai, J. Hou, T. Ma, X. Zeng, L. Zhang, and T. An. 2021. The source and transport of bioaerosols in the air: A review. Front. Environ. Sci. Eng. 15 (3):44. doi:10.1007/s11783-020-1336-8.
- Yoon, K. Y., C. W. Park, J. H. Byeon, and J. Hwang. 2010. Design and application of an inertial impactor in combination with an ATP bioluminescence detector for in situ rapid estimation of the efficacies of air controlling devices on removal of bioaerosols. Environ. Sci. Technol. 44 (5):1742–46. doi:10.1021/es903437z.
- Yoo, M. S., M. Shin, Y. Kim, M. Jang, Y. E. Choi, S. J. Park, J. Choi, J. Lee, and C. Park. 2017. Development of electrochemical biosensor for detection of pathogenic microorganism in Asian dust events. Chemosphere 175:269–74. doi:10.1016/j.chemosphere.2017.02.060.
- Zhang, C., R. Zhu, and W. Yang. 2016. A micro aerosol sensor for the measurement of airborne ultrafine particles. Sens. (Basel) 16 (3):399.
- Zhang, P., Y. Zhao, X. Liao, W. Yang, Y. Zhu, and H. Huang. 2013. Development and calibration of a single UV LED based bioaerosol monitor. Opt. Express 21 (22):26303–10. doi:10.1364/OE.21.026303.
- Zhang, Z., C. Wang, L. Zhang, Q. Meng, Y. Zhang, F. Sun, and Y. Xu. 2017. Fast detection of Escherichia coli in food using nanoprobe and ATP bioluminescence technology. Anal. Methods 9 (36):5378–87. doi:10.1039/C7AY01607G.
- Zhen, H., T. Han, D. E. Fennell, and G. Mainelis. 2013. Release of free DNA by membrane-impaired bacterial aerosols due to aerosolization and air sampling. Appl. Environ. Microbiol. 79 (24):7780–89. doi:10.1128/AEM.02859-13.