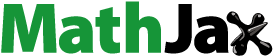
ABSTRACT
In 2019, an air emissions field sampling study was conducted by the Colorado Department of Public Health and Environment’s Air Pollution Control Division (APCD) at four commercial cannabis cultivation facilities. Measurements of ambient biogenic volatile organic compounds (VOC) concentrations were collected from various growing stages of cannabis (vegetative and flowering) and during post-harvest activities (drying and trimming). These data were then used to determine room-specific biogenic VOC emission rates for three of the facilities from the vegetative stage of the life cycle through post-harvest activities. This study shows that the magnitude of biogenic VOC emissions within a cannabis cultivation facility varies widely with the highest emission rates of up to 7.18E–1 kg/hr found during mechanical trimming and up to 2.33E–1 kg/hr in the drying rooms. These were up to an order of magnitude higher than emission rates found in the cultivation rooms. For example, Facility A vegetative room had an emissions rate of 1.46E–2 kg/hr. Normalized by the amount of biomass present, the drying rooms had the highest VOC emissions rates, with a maximum rate of 1.6E–3 kg/hr/kg biomass. The flowering room rates were found to be up to 3.25E–4 kg/hr/kg biomass and drying rooms up to 1.16E–3 kg/hr/kg biomass. When normalized by plant count, emission rates in the flower rooms ranged from 8.11E–6 to 3.62E–4 kg/hr/plant. The dominant monoterpenes from sampling were β-myrcene, terpinolene, and D-limonene. These data suggest that the variability in emission rates across cannabis production will create a challenge in establishing a generalized emission factor for all facilities. Across the industry, cannabis cultivation conditions and strategies can vary widely impacting the amount and type of VOC emissions. Minimizing uncertainties for VOC emission from cannabis facilities requires site-specific information on air exchange rates, plant counts, cannabis strains, biomass, and if hand or mechanical processing is used.
Implications: This study found that the magnitude of biogenic VOC emissions within a cannabis cultivation varies widely throughout rooms found in the facility, with the highest emissions found during post-harvest activities (i.e. trimming) and the lowest rates in the vegetative room. These data suggest that the large emission sources of VOCs are found post-harvest and emission inventories based solely on cultivation emissions will underestimate total biogenic VOC emissions from indoor cannabis cultivation facilities. The dominant measured terpenes throughout all facilities from cultivation to post harvest were: β-myrcene, terpinolene, and D-limonene.
Introduction
Colorado legalized the medical and recreational use of cannabis in 2000 and 2012, respectively. Since Colorado began implementing cannabis licensing, the cannabis industry has grown rapidly. In Colorado, large-scale commercial cannabis cultivation occurs primarily indoors, allowing for greater compliance and process control along with optimizing crop-yield and permitting year-round harvesting. Due to the infrastructure requirements of these indoor cultivation facilities, and zoning restrictions, most of these cannabis cultivation and cannabis infused product manufacturing facilities are located in dense urban and industrial areas within retrofitted warehouses, according to the Colorado Marijuana Enforcement Division.
As cannabis plants grow, they naturally emit biogenic volatile organic compounds (VOC), primarily as monoterpenes, with species found in many other plants and trees (Fischedick et al. Citation2010; Fuentes et al. Citation2000; Marchini et al. Citation2014; Ross and ElSohly Citation1996; Samburova et al. Citation2019; Sharkey, Wiberley, and Donohue Citation2008; Turner et al. Citation1979; Urso et al. Citation2022; Wang et al. Citation2019a). VOCs are an important precursor for ozone (O3) and particulate matter (PM) and could raise concerns related to worker safety when exposed to high concentrations indoors. This could be important for indoor settings, where concentrations can be many times higher than outdoor settings. Understanding the potential air quality impacts of cannabis cultivation is complicated by a lack of understanding about the types and magnitude of VOCs emitted from cannabis along with the correlation with light and temperature. Each strain of cannabis has unique biochemical processes in its metabolism that results in a unique composition of terpenes (Fischedick et al. Citation2010). Studies that have examined the emissions profile of terpenes in the cannabis bud and cannabis-derived oils find a high concentration of monoterpenes, sesquiterpenes, and cannabinoids, with monoterpenes typically dominating the terpene profile (Fischedick et al. Citation2010; Marchini et al. Citation2014; Ross and ElSohly Citation1996; Samburova et al. Citation2019; Turner et al. Citation1979; Urso et al. Citation2022). A study estimated the basal emission rate (BER) of VOCs from four strains of cannabis in an enclosure experiment with a limited number of plants (not representative of a commercial cultivation) and found a range of 4.3 to 8.6 µg C g−1 hr−1 BERs between different strains of cannabis and that the emissions are dependent on environmental conditions (Wang et al. Citation2019a). These data suggest emission profiles from cannabis cultivation could vary widely across facilities due to cultivation strategies, the cannabis strains grown, and the type of indoor environmental control methodologies that are applied. These factors present a challenge in efforts to develop industry-wide emission inventories.
Plants at indoor cannabis facilities are generally cultivated within 2–5 months, with three primary stages of plant growth (clone, vegetative, and flowering) that are segregated into different rooms of varying light and environmental conditions (e.g., temperature and humidity) to optimize plant growth. Due to the high intensity lighting and plant transpiration, cultivation facilities have high the heating, ventilation, and air conditioning (HVAC) loads to remove heat and moisture. It is important to note that one of the biggest advantages of indoor cultivation is the full plant life cycle is condensed from 4 to 7 months when grown outside down to 2–5 months of cultivation inside a building. This means the VOC emissions rate could potentially be higher for indoor plants compared to outdoor grown plants due to the condensed life cycle.
Given the limited number of observed emission factor data, and the large variety of strains of cannabis, there still exists large uncertainties for VOC emission inventories for cannabis cultivation. These cultivation emissions, however, only represent part of the total potential emissions from activities in a facility. After the plants have fully developed flowers, they are harvested, dried, and processed. In the harvesting process, excess stalks, stems, and fan leaves are cut and discarded and the flowers and sugar leaves are dried in separate areas in a controlled process before the sellable flowers are trimmed, cured, and packaged. The trimming process can vary and can be multi-stage from hand-trimming to utilizing high-speed mechanical trimming to remove the excess leaves off the flowers before sale. The excess plant material and some flowers typically go to an extraction process to make various cannabis products (e.g., concentrates, edibles, and topicals). Some extraction processes utilize VOC-based solvents like propane, butane, and ethanol in closed-loop systems (Samburova et al. Citation2019; Valizadehderakhshan et al. Citation2021). In addition to solvent VOCs, there could be wound-compound (pentene and hexane alcohols and aldehydes) VOC emissions from the harvesting, trimming and drying procedures which also add to the total VOC profile. (Ameye et al. Citation2018) After the flowers are trimmed for sale, they are dried and cured. Curing is an additional drying and aging stage where the flowers are sealed for various amounts of time before they are vented in a repeating process to slowly dry the flowers before packaging and sale; this process is similar to aging cheese.
Quantifying VOC emissions from commercial indoor cannabis cultivation facilities is critical to predict the impact on air quality (Samburova et al. Citation2019; Urso et al. Citation2022; Wang et al. Citation2019b). Further, specifically for indoor facilities, the VOC emissions from the plant cultivation process could have implications on worker health for individuals who work in the facility, but more research is needed. There is, however, a data gap on emission rates from post-harvest activities meaning that any quantification of cultivation facility emissions must include all emissions from seed to sale to reduce uncertainties. The following describes a collaborative field study with the cannabis cultivation industry and Colorado Department of Public Health and Environment’s (CDPHE) Air Pollution Control Division (APCD) to quantitatively estimate biogenic VOC emissions rates and speciation profiles for various stages of plant growth and during post-harvest activities within commercial indoor cannabis cultivation facilities. All study-related data is included within the tables (in manuscript and supplemental documents) besides the PID data are available on request from CDPHE as it is in multiple very large files.
Methods
CDPHE APCD’s staff conducted sample collection at four indoor cannabis cultivation facilities in Colorado, laboratory analysis of samples was conducted by Desert Research Institute (DRI), and indoor air quality modeling was conducted by the University of North Carolina – Chapel Hill. The laboratory analyses for samples were limited to biogenic terpenes and did not include measurements of solvent emissions.
Cultivation facility selection and details
In 2019, there were 1,167 licensed cannabis cultivation facilities in Colorado according to the Colorado Department of Revenue’s Marijuana Enforcement Division. Study participation was elicited by reaching out to cannabis industry stakeholders about the study and doing preliminary site evaluations at interested cultivation facilities to select representative facilities to sample. Facility selection criteria included diversity in strains grown, facility size, and location. As shown in , four cultivation facilities volunteered to anonymously participate in this study: two large (>100,000 harvested plants per year) facilities (Facility A and B), one medium (Facility D) (>20,000 harvested plants per year), and one small (Facility C) (<1,000 harvested plants per year).
Table 1. Facility details.
Facility A has an infrastructure that was explicitly designed for precise climate-controlled cannabis cultivation with two main exhaust points (north and south). There are currently 64 carbon filters in the north exhaust and 48 carbon filters in the south exhaust, for a total of 112 carbon filters at Facility A. The filters are changed when cannabis odor is detected at the perimeter of the parking lot which is monitored on a bi-weekly basis. Exhaust filtration reduces the likelihood that a significant fraction of released VOCs are recycled back to into the building through HVAC intakes.
Facility B has 60 20-ton rooftop HVAC units that recirculate recycled-air within the facility and only one dedicated exhaust point for air to leave the building that is located on the side of the building away from the rooftop HVAC units. This single exhaust point and other recirculation points do not have any installed filtration but are in different locations so mixing of inlet and exhaust is minimized.
Facility C has a unique HVAC system that utilizes a “lung room.” Intake air is pulled from the lung room and exhaust air is pumped into the lung room, creating a challenge for air sampling. Unfortunately, it was not possible to calculate any emission rates or an air exchange rate for Facility C due to the complex HVAC system causing unpredictable mixing of inlet, ambient, and exhaust air.
Facility D has a combination of evaporative cooling and traditional rooftop HVAC units. Additionally Facility D has at least two exhaust points per room with plant activity (growing, flowering/harvesting, drying/curing) that maintain negative pressure within the facility. Each exhaust port is equipped with a carbon filter that is changed annually. Exhaust filtration reduces the likelihood that a significant fraction of released VOCs are recycled back to into the building through HVAC intakes. Based on our evaluation of HVAC systems in facilities A, B, and D, we assume a clean background for calculations.
Supplementary Table S1 in the supplemental documents lists the cannabis strain types grown at each facility by the percent of the total number of plants harvested in 2019. Note that in supplementary Table S1, strains that individually represent less than 1% of the total harvest are grouped together at the bottom of each column to condense reporting to the more significant strains grown. Supplementary Table S2 and supplementary Table S3 in the supplemental documents show the plant count and strain type for the flowering rooms and weight of product by strain types in the drying room for facilities A, B, and D on the day of sample collection.
Sample collection methods
CDPHE APCD staff collected air samples from various rooms (vegetative, flower, drying, and trimming) inside Facilities A, B, C, and D by pulling air through porous polymer adsorbent (Tenax TA) filled glass tubes at a constant flow rate of approximately 25–40 ml/min for durations ranging from 5 to 20 minutes per sample, based on the expected concentrations determined from preliminary photoionization detector (PID) monitoring. Indoor samples were collected in areas that were generally the middle of the rooms, at points that were considered to be well mixed from the various ventilation points. Duplicate (parallel) tube samples were also taken at each facility, to compare parallel sample results to ensure accuracy in laboratory analysis. To collect the duplicate samples, a second identical tube sampling setup was added with the two tube inlets placed as close as possible to one another during the sampling. The pumps were operated in identical manners for the same amount of time to ensure comparability of the samples. After sampling, all tubes were immediately sealed with brass end-caps and placed on ice for storage, then overnight cold-pack shipped to DRI in Reno, NV for analysis. Laboratory blanks were run to determine any bias from the analytical processes. No blank cartridge samples were taken during the indoor study in an effort to efficiently make use of the small number of samples we had the budget to collect. The uncertainties in the instruments used for the study are listed in supplementary Table S4 of the supplemental documents.
Two ppbRAE 3000 PID units calibrated to 10 ppm isobutylene and set to a flow rate of 400 ml/min were used to monitor total VOC concentrations for about a week at each sample collection point at each facility to determine an optimal sampling time for subsequent Tenax tube sample collection. Periods of maximum and minimum concentrations were targeted for sampling, within the operating hours of the volunteering facilities for sampling access. PID sampling details are in supplementary Table S5 of the supplemental documents. PID data are available on request from CDPHE as the data files are very large.
As an example of how CDPHE used the PID data to determine tube sampling times representative of minimum and maximum concentrations, shows the 1-s interval PID concentrations in ppb that were monitored within the Facility B drying room for an 8-day period from 7/25/19 to 8/1/19. The Facility put a fresh batch of cannabis in the drying room the day the PID monitoring started and the downward trend in represents the overall concentration dropping as the cannabis dries out. The intermittent concentration spikes during staff operating hours are from the curing operation of venting air out of sealed containers of aging cannabis stored within the drying room, releasing additional terpenes within the drying room. The graph shows the VOC concentrations are influenced by staff operations but do not drop completely outside of operating hours in the evenings and over the weekend as the cannabis continues to dry in the room. After evaluating the data in , the Facility B Tenax tube samples were collected in the drying room on Wednesday 8/14/2019 in the morning to represent a low VOC concentration and then in the afternoon after fresh batch of cannabis was loaded into the drying room to represent a high VOC concentration. Note that sample collection times were restricted to facility operating hours for security purposes. The average total VOC concentration from the Tenax tube sample collected in the Facility B drying room was 4.59E +03 µg/m3.
Figure 1. Total VOC concentration data in ppb from the PID within Facility B Drying Room 7/25/19 to 8/1/19.
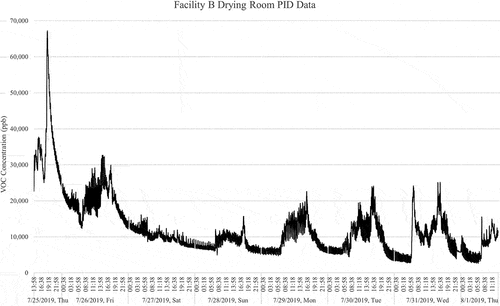
This sampling methodology was applied to analyzing all the PID data at the various sampling locations to determine optimal tube sample collection times that represent low and high VOC concentrations at each location within the constraints of sampling within operating hours. For example, only one sample was collected in a vegetative room at Facility A after the PID Data showed consistently low VOC concentrations, comparable with the findings in other cannabis cultivation emissions studies (Samburova et al. Citation2019; Wang et al. Citation2019a). With the vegetative room being a low contributor to the overall cannabis cultivation emissions profile the limited tube sampling budget was utilized to capture the larger VOC emissions contributors like the flowering, drying and trimming rooms.
shows the 1-s interval PID concentrations in ppb that were monitored within the Facility B trimming room for the same 8-day period as the drying room in ; from 7/25/19 to 8/1/19. The graph shows the VOC concentrations drop outside of operating hours in the evenings and over the weekend when staff are not actively trimming. In there are spikes in the VOC concentrations on weekday afternoons, this is when facility staff clean the trimming room with solvent-based cleaner after the mechanical trimming is done for the day. The tube sampling only captures terpenes emissions, the focus of the study, and not solvent emissions. The Facility B Tenax tube samples were collected in the trimming room on Wednesday 8/14/2019 in the morning to capture hand trimming representative of a low VOC concentration and in the afternoon during mechanical trimming to represent the high VOC concentrations seen in . The average total VOC concentration from the Tenax tube sampling in the Facility B trimming room was 3.14E +03 µg/m3.
Figure 2. Total VOC concentration data in ppb from the PID within the Facility B trimming room 7/25/19 to 8/1/19.
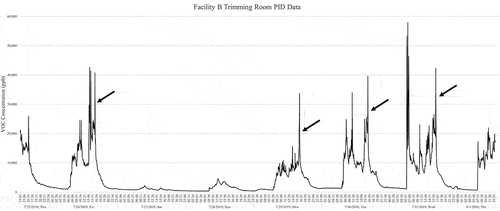
Tube sample collection times were based on the high and low concentration times captured by the PID units as discussed above. Tube samples were collected using constant flow sampling pumps made by Markes International and calibrated to an optimal sample flow rate (informed by PID results) using a reference flowmeter. Sampling times were limited to 5–15-min periods, dependent upon the concentrations seen in the data collected from the PIDs at the sampling points, to avoid saturation and/or breakthrough of the Tenax media. When room size and contents allowed, sample tubes were placed in approximately the center of the room being sampled, about halfway between the floor and the ceiling in an effort to be at a well-mixed point in the room based on the placement of exhaust vents, the entry/exit points of the room, and maintaining a safe working environment for the cannabis industry employees. Tube sampling details and results are in supplementary Tables S6 and S7, respectively, in the supplemental documents.
Sample analysis methods
After the Tenax tube samples were collected, they were sealed, kept cold, and shipped overnight to the DRI laboratory. At the DRI laboratory, biogenic VOCs (terpenes) were desorbed from the Tenax tubes using an automated thermal desorber (TurboMatrix 650), followed by gas chromatographic separation on Clarus 680 and analysis by mass spectrometry on Clarus SQ8 (all equipment by PerkinElmer, CT, USA). An Elite-5 MS (30 m × 0.25 mm I.D. × 0.25 μm) column (PerkinElmer) was used in the gas chromatograph (GC). The thermal desorber was operated such that 10% of the sample was transferred to the GC, while the remaining part was collected on a clean Tenax tube in case a reanalysis was required. VOCs were identified by their retention time and mass spectra. The target biogenic VOC compounds and sample results are listed in supplementary Table S7 of the supplemental documents. These target compounds were quantified using calibration with analytical standards [Restek Cannabis Terpenes standard (Restek Co., Bellefonte, PA)] that were transferred to Tenax tubes that were subsequently desorbed and analyzed. The average R2 value for calibration curves was 0.966 (the median value was 0.995) and the calibration range was 20 ng to 1 μg. Minimum detection limits (MDL) were measured by exposing pre-cleaned Tenax tubes to small amounts of terpenes. MDLs for individual compounds ranged between 0.6 and 1.8 ng per sample. Reproducibility of the analysis results was assessed using field-collected replicate samples, which on average was 21% (median reproducibility was 7%). The Tenax tube sampling data obtained from DRI was blank corrected by taking the average concentration for each compound in the blank samples and subtracting them from the corresponding compound concentration values reported by the laboratory. This correction did not amount to significant changes in the concentrations of the compounds detected.
Emissions rate calculation
Each room within each facility is modeled as a completely mixed flow reactor at steady state. A dynamic mass balance on BVOCs is therefore given by:
where is the mass flow rate into the room (kg/hr),
is the mass flow rate out of the room (kg/hr), R is the loss due to chemistry (kg/hr), ER is the emission rate (kg/hr), and L is the loss due to dry deposition.
EquationEquation (1)(1)
(1) is simplified by assuming that the rate mass transport into the room, Fin, is zero (i.e., outdoor concentrations are very low). For dry deposition, it was assumed that VOCs would equilibrate rapidly with room surfaces, i.e., that the rates of deposition to, and re-emission from, indoor surfaces are equal and therefore would have a negligible impact on emission rates (Won Citation2001). Thus, L was assumed to be zero. It is important to note that this assumption of rapid equilibrium is only valid if the facilities did not clean indoor surfaces frequently. Considering these assumptions, the mass balance simplifies to EquationEquation (2).
(2)
(2)
The mass flowrate out of the building is given by the measured biogenic VOC concentration, CBVOC (kg/m3), times the air exchange rate, ë, (1/h) and building volume, V (m3), both provided by the facility.
For emission rates reported in the results, we assume the biogenic VOC removal by reaction, R, is zero resulting in EquationEquation (6)(6)
(6)
The uncertainty in the emission rate is dependent on the uncertainty in the concentration values, air exchange rate, and volume. The uncertainty and detection limits for the concentration are described in the Sample Analysis Section.
The largest source of uncertainty is the air exchange rate, ë, or ventilation rate, (ë × V). These were reported by the facility but were not measured onsite. We discuss the implications of that uncertainty in the results, noting that we do not have a basis for estimating the magnitude of the uncertainty in ventilation or air exchange rates for all but one facility. The VOC signal, as measured by PID, cannot be considered a conserved species for tracer decay determination of air exchange rates (e.g. ASTM method E741–11(2017)). VOCs adsorb and desorb from surfaces, causing decay rates to change over time. However, sharp VOC peaks due to facility cleaning in the trimming room of Facility B rose well above background VOC levels and rapidly decayed in a way that may be used to roughly estimate the air exchange rate. Therefore, the PID results for Facility B during time periods indicated by the arrows in were used to roughly estimate air change rates, recognizing that these values are only approximations for comparison with the facility reported values.
Because chemistry may meaningfully reduce the apparent emission rate, we evaluated this assumption by estimating an upper bound on the losses due to chemistry. To do this, it was assumed that BVOCs react primarily with O3 and the hydroxyl radical, OH as shown in EquationEquation (6)(6)
(6)
where kOH and kO3 (m3 kg−1 s−1) are the second-order rate constants for the reaction of OH and O3 with the biogenic VOC, COH is the hydroxyl radical concentration, CO3 is the O3 concentration and V is the volume of the room in which sampling occurred.
Since OH can be generated by photolysis and by O3 chemistry inside the facility, it was assumed that a steady OH concentration develops and can be estimated from prior literature (Alvarez et al. Citation2013). The term kOHCOH can be compared directly with the air exchange rate to evaluate the relative importance of this chemistry in reducing the concentrations of biogenic VOCs. We make this comparison with air exchange for OH chemistry by assuming a high indoor value for COH from prior literature (Alvarez et al. Citation2013) and report the mass rate of chemical removal by OH as shown EquationEquation (6)(6)
(6) (kOHCOHCBVOCV).
For O3, we use a different method to determine the loss due to O3. The indoor O3 concentration may vary from a maximum of that outdoors to zero, depending on the air exchange rate and concentration of reactive monoterpenes. The monoterpenes emitted by cannabis plants are reactive with O3, and concentrations are high; given enough residence time, much of the O3 that enters the building could be titrated out by the monoterpenes present (Weschler Citation2000). Rather than use the removal rate method applied for OH, we estimate the upper-bound removal rate of biogenic VOCs, by assuming that all infiltrated O3 has been titrated by reaction with monoterpenes. For a 1:1 stoichiometric reaction between O3 and a monoterpene, the mass removal rate is simply the infiltration rate of O3 from outside, multiplied by the appropriate molecular weight conversion as shown in EquationEquation (6)(6)
(6)
where MWBVOC and MWO3 are the molecular weights of a typical monoterpene (136 g/mol) and O3 (48 g/mol).
Speciated monoterpene concentration sample results from the Tenax Tube samples are listed in supplementary Table S7 of the supplemental documents. As shown in supplementary Table S7, there were 23 compounds that were identified across all facilities and rooms. The highest VOC concentrations were in the mechanical trimming room where β-myrcene concentrations reached 2.31E4 μg/m3. In contrast, the concentration of β-myrcene in the hand trimming rooms was an order of magnitude lower. It is important to note that the mechanical method is also able to trim a larger volume of product when compared to hand trimming. The drying room also had significant concentrations of β-myrcene reaching a maximum concentration of 1.5E4 μg/m3 in Facility D. Only one vegetative room sample was available and was from Facility A’s vegetative room with the highest measured concentration, also being β-myrcene, at 4.8E2 μg/m3. The VOC concentrations in the vegetative room are an order of magnitude less than found in the other rooms in Facility A and that is why only one vegetative room sample was collected in this study with a limited sampling budget. The rest of the Tenax tube sampling budget was focused on capturing the rooms with higher emission rates (i.e., flower, drying, and trimming rooms).
As shown in supplementary Table S7 of the supplemental documents, when averaged across all rooms and facilities, β-myrcene had the largest concentration with 6.41E4 μg/m3 followed by terpinolene with 2.92E3 μg/m3 and D-Limonene with 1.72E3 μg/m3. provides the average measured concentration data for each room across all facilities for the top five biogenic VOC compounds. As shown in , β-myrcene was the top measured compound in all rooms, with the highest concentrations in the trimming rooms (average of 8.35E3 μg/m3). The top five biogenic VOC compounds are consistent among the rooms, except for a lack of terpinolene in all the trimming rooms and within the Facility A vegetative room. This sampling study also found notable amounts of ã-terpinene emissions from the drying rooms in Facility C and Facility D but only trace levels at Facility A and B, which is most likely attributed to the unique strains grown at the facility. Eucalyptol was relatively low in all samples collected in this study. The similarity in dominant monoterpene species across facilities is surprising given the large variety of unique cannabis strains present in the flower and drying rooms at each facility. Supplementary Table S1 of the supplemental documents shows the number and type of cannabis strains for each facility in 2019 and supplementary Tables S2 and S3 in the supplemental documents show the strains specifically in the flowering and drying rooms during sampling. It is evident from these supplementary tables that there are a large number of reported cannabis strains where each facility has its own unique profile of strains being processed, but all still share the dominant monoterpene species.
Table 2. Dominant terpenes sampled in rooms across all facilities and average concentrations.
All the biogenic VOC species listed in supplementary Table S7 of the supplemental documents were summed into a total biogenic VOC concentration for each room and are listed in . As described previously, the mechanical trimming rooms measured the highest concentration of total biogenic VOCs of 4.35E4 μg/m3 and the vegetative room measured the lowest average concentration of 7.26E2 μg/m3. To determine emission rates for each room sampled, the air exchange rate (AER) and volume of the room were used from three of the four facilities as shown in . It was not possible to calculate an emission rate for Facility C due to the characteristics of the HVAC system where an inconsistent mixing of inlet, ambient, and exhaust air prevented an accurate AER calculation. Facility A had the highest AER of 2-min room exchange rates. Other differences in facilities included the method by which trimming is completed, which has a direct impact on emission rates. For example, Facilities A and B utilize high-speed mechanical trimmers that resulted in higher emissions versus Facilities C and D that relied on hand-trimming as shown in . Based on this facility-specific data, a total biogenic VOC emission rate (kg/hr) for each room was calculated and is shown in (except for Facility C). The largest emission rate was 7.18E–1 kg/hr in the mechanical trimming room in Facility A. In contrast, the biogenic VOC emission rates in hand trimming rooms were an order of magnitude smaller. The flower rooms also had significant emission rates of up to 3.25E–1 kg/hr.
Table 3. Calculated cannabis cultivation VOC emission rates.
Each of the four facilities sampled had different strains of cannabis (supplementary Table S1), varied in the weight of plants at harvest (), and the 2019 annual number of plants grown by each facility (). Using these average values, the emission rates in the flowering rooms were normalized by biomass as shown in . Based on these normalized values, Facility A’s flower room had the highest rates of 3.14e-4 kg/hr per kg of biomass. It is important to note that the emission rates varied by five orders of magnitude suggesting that the large variety in strains and varying growing strategies at each facility influences the emission rates of the flowering rooms. These large variations suggest large uncertainties in any attempt to build flower room emission inventories on average or on limited strain-specific emission rate data.
The facilities also reported the amount of biomass present in the sampled drying rooms in Table S6 of the supplemental documents. Based on these values, normalized emission rates for the drying rooms are shown in . The largest emission rate of 1.16E–3 kg/hr/kg was measured in the drying room of Facility A. The emissions in the drying room were higher than those found in the flower rooms. The highest emission rate in the flower rooms was 3.14E–4 kg/hr/kg in Facility A. Since the facilities provided plant counts for the flowering and vegetative rooms, an emission rate per plant was also calculated and is shown in . As expected, the younger plants found in the vegetative room had the lowest emission rates of 8.11E–6 kg/hr/plant. The emission rates in the flowering rooms, however, were 1–2 orders of magnitude higher with the largest rate found in Facility D’s flower room with a value of 3.62E–4 kg/hr/plant.
A sensitivity analysis was completed to estimate the magnitude of loss rates due to chemical losses and uncertainties in the AER. For loss of VOCs by chemical reactions, we consider reactions with O3 and the OH radical. Many of the primary monoterpenes emitted are very reactive with O3. We assume that O3 can only enter the space by ventilation of outdoor air (i.e., there are no O3 generating devices in use in the buildings). Therefore, we can put an upper bound on the fraction of monoterpenes lost by assuming that all O3 that enters is titrated by monoterpenes and that one molecule of O3 removes one monoterpene molecule. For example, the summed concentration of monoterpenes in the flower room of Facility B was 1.05E13 molecules/cm3 and a typical background (outdoor) O3 mixing ratio is 50 ppb (1.23E12 molecules/cm3) (Pfister et al. Citation2019). Therefore, the total monoterpene concentration could have been as high as 1.17E13 molecules/cm3 (11% higher) in the absence of O3 chemistry. Across all facilities, the summed concentration of monoterpenes ranged from 1E13 to 1E14, suggesting that the upper bound loss of monoterpenes by reaction with O3 is in the range of 1–10%. An estimate of mass removal rate of biogenic VOCs by full titration of O3 by room and facility is shown in . To estimate the removal rate by reaction with OH, the median of reported values for kOH, 1.0E–10 cm3 molecule−1 s−1, was chosen (Atkinson Citation1997). A high value of the measured indoor concentration for COH of 1.8E6 molecules cm−3 was assumed (Gomez Alvarez et al. Citation2013). Therefore, the removal rate of a typical monoterpene is approximately 0.6/h. For Facility B, this is about 1/10 the air change rate (5.5/h) and therefore would be responsible for up to a ~ 10% underprediction of emission rates, compared with assuming no chemistry occurs. For Facility A, with an air change rate of 30/h, the underprediction is <0.5%. The estimated mass removal rate of total biogenic VOCs by reaction with OH (EquationEquation (6)(6)
(6) ) is provided in . To improve the estimates of removal by reaction with O3 and OH, a more detailed indoor chemistry model would need to be applied (Carslaw Citation2007).
Although the AERs were reported by each facility, they were not directly measured at the time of sampling. Further, AERs can vary widely among facilities based on their HVAC method. Given this uncertainty, AERs could be substantially higher or lower than reported. Emission rates as determined by EquationEquation (6)(6)
(6) are proportional to air exchange rates. For example, if air exchange rates are 25% higher or lower than reported, then actual emission rates will be 25% higher or lower. Although the air exchange rate is among the largest sources of uncertainty, estimates of the air exchange rate by decay of PID signals in the trimming room of Facility B (~4/h-6/h) were consistent with the reported value of 5.5/h.
Discussion and conclusion
This study found that the magnitude of biogenic VOC emissions for cannabis cultivation varies widely throughout rooms within a facility, with the highest emissions found during post-harvest activities (i.e., trimming), and the lowest rates in the vegetative room. Plant harvesting also generates green leaf volatiles (Ameye et al. Citation2018) not measured in the targeted Tenax tube analysis that will contribute to the PID signal data and increase overall biogenic VOC emission rates. The higher emissions during post-harvest activities were likely due to the physical disturbance of the harvested plants in trimming and were further enhanced with the vigorous movement caused by mechanical methods. This is consistent with findings from other studies that have found that surfaces of female flowers contain storage of resins rich in terpenoids which are likely being disturbed by these physical disturbances (Zager et al. Citation2019). These data suggest that the largest emission sources of VOCs are found post-harvest and emission inventories based solely on cultivation emissions will underestimate total biogenic VOC emissions. Biogenic VOC emission rates were also determined in a 2019 study at four cannabis growing facilities located in California and Nevada. Researchers reported the biogenic VOC emission rate for the grow room of one facility, with a value of 7.4E–4 kg/day/plant (Samburova et al. Citation2019). A 2018 leaf enclosure study in Denver found a maximum VOC emission rate of 2.4E–4 kg/day/kg biomass (Wang et al. Citation2019a). For this study, rates were found to be higher than the previous studies, but within the same order of magnitude. There are no comparable studies for emission rates found in post-harvest activities. This is significant as this study showed the highest emissions were from these post-cultivation activities.
In this study, the dominant measured monoterpenes throughout all facilities from cultivation to post harvest were: β-myrcene, terpinolene, and D-limonene. These top monoterpenes were also present at the exhaust points at each of the cannabis cultivation facilities where this study occurred (Urso et al. Citation2022). These same species were measured from a 2019 sampling of facilities in California and Nevada (Samburova et al. Citation2019). This study also reported a similar finding that eucalyptol was not a dominant species (Samburova et al. Citation2019). In 2019, an ambient field campaign completed in Denver also observed that β-myrcene and D-limonene were the dominant monoterpenes (Wang et al. Citation2020). Despite the diversity of cannabis strains grown at each facility, operational parameters, facility design, and sampling methodology, the dominant biogenic VOCs remain consistent.
Biogenic VOC emissions within indoor cannabis cultivation facilities could have potential impacts on both indoor and outdoor air quality via the chemical production of O3 and PM (Urso et al. Citation2022; Wang et al. Citation2019b). The data provided here is an important first step in building a cannabis facility emissions inventory of biogenic VOCs that could be used to inform on potential worker safety exposures and outdoor air quality impacts. Furthermore, these inventories could provide help in the design of HVAC and the sizing of mitigation technology. Our data suggest that the large variability in emission rates across facilities introduces uncertainty in current biogenic emission inventories used for modeling studies (Urso et al. Citation2022; Wang et al. Citation2019b). It is also important to note that this study had a small sample size, and given the large variability found in the industry, more sampling across different kinds of facilities is needed to understand the range of possible emission rates. This should also include the measurement of VOCs used in the extraction of concentrates with industrial solvents (Valizadehderakhshan et al. Citation2021). For example, a 2019 study found that concentrate manufacturing produced concentrations up to 43,000 μg/m3 of butane (Samburova et al. Citation2019). To minimize these uncertainties in emission inventories will require site-specific information on AER, plant counts, cannabis strains, biomass, and types of hand or mechanical processing. All these activities and industrial solvents must be considered in the development of a cannabis emission inventory.
Acronym glossary
AER | = | air exchange rate |
APCD | = | Air Pollution Control Division |
BER | = | basal emission rate |
CDPHE | = | Colorado Department of Public Health and Environment |
DRI | = | Desert Research Institute |
GC | = | gas chromatograph |
hr | = | hour |
HVAC | = | heating, ventilation, and air conditioning |
kg | = | kilogram |
lb | = | pound |
m | = | meter |
min | = | minute |
ml | = | milliliter |
MDL | = | minimum detection limit |
MST | = | Mountain Standard Time |
ng | = | nanograms |
O3 | = | ozone |
[OH]− | = | hydroxyl radical |
PID | = | photoionization detector |
PM | = | particulate matter |
ppm | = | parts per million |
ppb | = | parts per billion |
ppbv | = | parts per billion by volume |
µg | = | microgram |
µg/m3 | = | micrometers per cubic meter |
VOC | = | volatile organic compound |
Disclaimer
This document has been reviewed and approved for publication by all contributing authors.
SUPPLEMENTAL_DOCUMENTATION.docx
Download MS Word (41.2 KB)Acknowledgment
The authors want to acknowledge the time and efforts of the cooperating volunteer cannabis cultivation facilities throughout this study. The authors also thank Sara Heald, Kristen Good, and Daniel Bon of CDPHE, Chi-tsan Wang of the University of North Carolina, Chiranjivi Bhattarai, Irina Lebedeva, and Vera Samburova of Desert Research Institute, Christine Wiedinmyer of the University of Colorado in Boulder, Micheal Wolf of Washoe County Air Quality, and Joe Southwell of Spokane Regional Clean Air Agency for their scientific inputs that helped inform this study and publication. The authors also thank CDPHE management staff for their ongoing support of this study. Specifically, Garry Kaufman and Leah Martland. Thanks to the 2018 CDPHE Innovation Grant for funding for this study. Thanks to the University of North Carolina - Chapel Hill for cost-sharing the publication fees with CDPHE.
Disclosure statement
No potential conflict of interest was reported by the author(s).
Data availability statement
The authors confirm that the data supporting the findings of this study are available within the article and its supplementary materials besides the PID data that is available on request from CDPHE as it is multiple very large files. Contact the corresponding author, Kaitlin Urso, to request PID data.
Supplementary material
Supplemental data for this article can be accessed online at https://doi.org/10.1080/10962247.2023.2175741
Additional information
Funding
Notes on contributors
Kaitlin Urso
Kaitlin Urso is an environmental consultant with the Colorado Department of Public Health and Environment specializing with in helping small businesses reduce their environmental impacts and comply with regulations. Kaitlin was the lead author and project engagement manager for this study on indoor cannabis air emissions and for the “Terpene exhaust emissions and impact ozone modeling from cannabis plants at commercial indoor cultivation facilities in Colorado” study that took place simultaneously at the same cultivation facilities (Urso et al. Citation2022). She has 12 years of technical environmental experience and a mechanical engineering degree from the University of Colorado, Boulder. Kaitlin's work has been featured in Wall Street Journal, Forbes, CNN, NPR, Science Magazine, and countless other media and industry publications.
William Vizuete
William Vizuete is a Professor in the Department of Environmental Sciences and Engineering at the University of North Carolina at Chapel Hill. For the last 17 years he has been tackling the public health problems related to air pollution. In his research, he focuses on understanding how the atmosphere can change the formation processes of ozone and PM, and its connection to human health. Through his research Dr. Vizuete has increased our scientific knowledge in these areas and produced new insights through air quality models, field studies, laboratory experiments, and the development of a novel in vitro technology. Dr. Vizuete contributed to the concept of the paper, the analysis of data, writing the manuscript, producing tables, and overall editing.
Ryan Moravec
Ryan Moravec graduated from University of North Carolina at Chapel Hill in May 2022 with a Masters of Science in Environmental Engineering. His research explored the impacts of ozone induced oxidation of skin surface lipids. Ryan assisted in the modeling of this publication by calculating the emission rates of biogenic VOC and the chemical reaction analysis.
Andrey Khlystov
Andrey Khlystov is a Research Professor and the Director of the Organic Analytical Laboratory at the Desert Research Institute (DRI) with more than 30 years of experience in various aspects of air pollution research. For this study, Andrey led the laboratory analysis of collected samples. He has designed, organized, and directed several medium to large scale laboratory and field studies funded by the National Institutes of Health, the National Science Foundation, the EPA, and several other federal and non-governmental sponsors.
Alicia Frazier
Alicia Frazier is a physical scientist with the Colorado Department of Public Health and Environment’s Air Pollution Control Division. She specializes in planning and performing a variety of air toxics sampling activities. Alicia designed and implemented the sampling process for this study on indoor cannabis air emissions. She has over 20 years of environmental sampling experience, and a M.S. in Astrophysical, Planetary and Atmospheric Science from the University of Colorado.
Glenn Morrison
Glenn Morrison is a professor of Environmental Science & Engineering at the University of North Carolina and has been studying indoor and outdoor air pollution and human exposure for 30 years. He received his PhD from Berkeley, and his research has primarily been related to indoor physics and chemistry. He has a particular expertise in interfacial chemistry, having also worked as a chemical engineer on heterogeneous catalysis. This research has included ozone-surface chemistry, acid-base chemistry and its role in sorption to indoor surfaces, methamphetamine contamination in buildings, aerosol-SVOC modeling and field measurements of reactive oxygen species in homes. From 2014-2016 he was the President of the International Society of Indoor Air Quality and Climate (ISIAQ). Glenn Morrison contributed to the modeling and sensitivity analysis for this study, specifically focused on indoor chemistry and its influence on estimated emission rates.
References
- Alvarez, H. B., R. Sosa Echeverria, P. S. Alvarez, and S. Krupa. 2013. Air quality standards for particulate matter (PM) at high altitude cities. Environ. Pollut. 173:255–56. doi:10.1016/j.envpol.2012.09.025.
- Ameye, M., S. Allmann, J. Verwaeren, G. Smagghe, G. Haesaert, R. C. Schuurink, and K. Audenaert. 2018. Green leaf volatile production by plants: A meta-analysis. New Phytol. 220 (3):666–83. doi:10.1111/nph.14671.
- Atkinson, R. 1997. Gas-phase tropospheric chemistry of volatile organic compounds: 1. Alkanes and alkenes. J. Phys. Chem. Ref. Data 26 (2):215–90. doi:10.1063/1.556012.
- Carslaw, N. 2007. A new detailed chemical model for indoor air pollution. Atmos. Environ. 41 (6):1164–79. doi:10.1016/j.atmosenv.2006.09.038.
- Fischedick, J. T., A. Hazekamp, T. Erkelens, Y. Hae Choi, and R. Verpoorte. 2010. Metabolic fingerprinting of Cannabis sativa L., cannabinoids and terpenoids for chemotaxonomic and drug standardization purposes. Phytochemistry 71 (17–18):2058–73. doi:10.1016/j.phytochem.2010.10.001.
- Fuentes, J. D., M. Lerdau, R. Atkinson, D. Baldocchi, J. W. Bottenheim, P. Ciccioli, B. Lamb, C. Geron, L. Gu, and A. Guenther. 2000. Biogenic hydrocarbons in the atmospheric boundary layer: A review. Bull. Am. Meteorol. Soc. 81 (7):1537–75. doi:10.1175/1520-0477(2000)081<1537:BHITAB>2.3.CO;2.
- Gomez Alvarez, E., C. Afif, S. Gligorovski, C. Schoemaecker, C. Fittschen, J. F. Doussin, and H. Wortham. 2013. Unexpectedly high indoor hydroxyl radical concentrations associated with nitrous acid. Proc. Natl. Acad. Sci. 110 (33):13294–99. doi:10.1073/pnas.1308310110.
- Marchini, M., C. Charvoz, L. Dujourdy, N. Baldovini, and J. J. Filippi. 2014. Multidimensional analysis of cannabis volatile constituents: Identification of 5, 5-dimethyl-1-vinylbicyclo [2.1. 1] hexane as a volatile marker of hashish, the resin of Cannabis sativa L. J. Chromatogr. A 1370:200–15.
- Pfister, G., C. T. Wang, M. Barth, F. Flocke, W. Vizuete, and S. Walters. 2019. Chemical characteristics and ozone production in the Northern Colorado front range. J. Geophys. Res. 124 (23):13397–419. doi:10.1029/2019JD030544.
- Ross, S. A., and M. A. ElSohly. 1996. The volatile oil composition of fresh and air-dried buds of Cannabis sativa. J. Nat. Prod. 59 (1):49–51. doi:10.1021/np960004a.
- Samburova, V., M. McDaniel, D. Campbell, M. Wolf, W. R. Stockwell, and A. Khlystov. 2019. Dominant volatile organic compounds (VOCs) measured at four Cannabis growing facilities: Pilot study results. J. Air Waste Manage. Assoc. 69 (11):1267–76. doi:10.1080/10962247.2019.1654038.
- Sharkey, T. D., A. E. Wiberley, and A. R. Donohue. 2008. Isoprene emission from plants: Why and how. Ann. Bot. 101 (1):5–18. doi:10.1093/aob/mcm240.
- Turner, C. E., M. A. Elsohly, P. C. Cheng, and G. Lewis. 1979. Constituents of Cannabis sativa L., XIV: Intrinsic problems in classifying cannabis based on a single cannabinoid analysis. J. Nat. Prod. 42 (3):317–19. doi:10.1021/np50003a017.
- Urso, K., A. Frazier, S. Heald, and A. Khlystov. 2022. Terpene exhaust emissions and impact ozone modeling from cannabis plants at commercial indoor cultivation facilities in Colorado. J. Air Waste Manage. Assoc. 72 (8):1–21. doi:10.1080/10962247.2022.2046206.
- Valizadehderakhshan, M., A. Shahbazi, M. Kazem-Rostami, M. Scott Todd, A. Bhowmik, and L. Wang. 2021. Extraction of cannabinoids from Cannabis sativa L.(Hemp). Agriculture 11 (5):384. doi:10.3390/agriculture11050384.
- Wang, C. T., K. Ashworth, C. Wiedinmyer, J. Ortega, P. C. Harley, Q. Z. Rasool, and W. Vizuete. 2020. Ambient measurements of monoterpenes near Cannabis cultivation facilities in Denver, Colorado. Atmos. Environ. 232:117510. doi:10.1016/j.atmosenv.2020.117510.
- Wang, C. T., C. Wiedinmyer, K. Ashworth, P. C. Harley, J. Ortega, Q. Z. Rasool, and W. Vizuete. 2019b. Potential regional air quality impacts of cannabis cultivation facilities in Denver, Colorado. Atmos. Chem. Phys. 19 (22):13973–87. doi:10.5194/acp-19-13973-2019.
- Wang, C. T., C. Wiedinmyer, K. Ashworth, P. C. Harley, J. Ortega, and W. Vizuete. 2019a. Leaf enclosure measurements for determining volatile organic compound emission capacity from Cannabis spp. Atmos. Environ. 199:80–87. http://www.sciencedirect.com/science/article/pii/S1352231018307416.
- Weschler, C. J. 2000. Ozone in indoor environments: Concentration and chemistry. Indoor Air 10 (4):269–88. doi:10.1034/j.1600-0668.2000.010004269.x.
- Won, D., D. M. Sander, C. Y. Shaw, and R. L. Corsi. 2001. Validation of the surface sink model for sorptive interactions between VOCs and indoor materials. Atmos. Environ. 35:4479–88. doi:10.1016/S1352-2310(01)00223-0.
- Zager, J. J., I. Lange, N. Srividya, A. Smith, and B. M. Lange. 2019. Gene networks underlying cannabinoid and terpenoid accumulation in Cannabis. Plant Physiol. 180(4):1877–97, August. doi:10.1104/pp.18.01506.