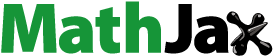
ABSTRACT
Per- and polyfluoroalkyl substances (PFAS) pose a major health and environmental problem. Methods are needed to ensure that PFAS are not released into the environment during their use or disposal. Alumina-based catalysts have been used for the abatement of small perfluorocarbons, e.g. tetrafluoromethane and perfluoropropane, emitted during the silicon etching process. Here, an alumina-based catalyst was tested to determine if these catalysts may facilitate the destruction of gas-phase PFAS. The catalyst was challenged with two nonionic surfactants with eight fluorinated carbons, 8:2 fluorotelomer alcohol and N-Ethyl-N-(2-hydroxyethyl)perfluorooctylsulfonamide. The catalyst helped decrease the temperatures needed for the destruction of the parent PFAS relative to a thermal-only treatment. Temperatures of 200°C were sufficient to destroy the parent PFAS using the catalyst, although a significant number of fluorinated products of incomplete destruction (PIDs) were observed. The PIDs were no longer observed by about 500°C with catalyst treatment. Alumina-based catalysts are a promising PFAS pollution control technology that could eliminate both perfluorocarbons and longer chain PFAS from gas streams.
Implications: The release of per- and polyfluoroalkyl substances (PFAS) into the atmosphere can cause problems for human health and the environment. It is critical to reduce and eliminate PFAS emissions from potential sources, such as manufacturers, destruction technologies, and fluoropolymer processing and application sites. Here, an alumina-based catalyst was used to eliminate the emissions of two gas-phase PFAS with eight fully fluorinated carbons. No PFAS were observed in the emissions when the catalyst was at 500°C, lowering the energy requirements for PFAS destruction. This shows that alumina-based catalysts are a promising area for research for PFAS pollution controls and the elimination of PFAS emissions into the atmosphere.
Introduction
Per- and polyfluoroalkyl substances (PFAS) are a class of man-made compounds with strong carbon-fluorine bonds and low surface energies (O’Hagan Citation2008). These unique properties make PFAS excellent for a variety of applications: inert containers, high temperature lubricants and seals, stain-resistant coatings, aqueous film forming foams (AFFF), cosmetics, artificial blood substitutes, and more (Goldwhite Citation1986; Lemal Citation2004; Okazoe Citation2009). The widespread use of PFAS and their stability have led to the accumulation of some PFAS in water (Ahrens Citation2011; McCord and Strynar Citation2019; Sunderland et al. Citation2019; Thompson et al. Citation2022), soil (Bolan et al. Citation2021; Houtz et al. Citation2013; Milley et al. Citation2018), air (Ellis et al. Citation2004; Faust Citation2022; Jahnke et al. Citation2007), plants (Freeling et al. Citation2022; Ghisi, Vamerali, and Manzetti Citation2019; Li, Sun, and Li Citation2022; Zhao et al. Citation2022), animals (Giesy and Kannan Citation2001; Haukas et al. Citation2007; Stockin et al. Citation2021), and humans (McDonough et al. Citation2022; Pan et al. Citation2017; Sunderland et al. Citation2019). The bioaccumulation of some PFAS, particularly perfluorinated surfactants over six carbons long, in organisms allows even low concentrations of exposure over time to have harmful health effects, such as low birth weight, elevated cholesterol, and cancer (McDonough et al. Citation2022; Pan et al. Citation2017; Sunderland et al. Citation2019).
The development of effective methods to treat PFAS-contaminated sources and to control the release of PFAS into the environment is a high priority (U.S. EPA Citation2021). PFAS are difficult to destroy completely, break all the carbon-fluorine bonds, using incineration (Krug et al. Citation2022; Seay et al. Citation2023; Wang et al. Citation2022) and many other PFAS treatment technologies (Berg et al. Citation2022). PFAS, and fluorinated products of incomplete destruction (PIDs), can be emitted into the atmosphere from treatment methods, their industrial use and manufacturing, and the commercial use of PFAS (Hercules et al. Citation2017; LaZerte et al. Citation1953; Tsang, Burgess, and Babushok Citation1998). Organofluorine PIDs are problematic, because they are among the most potent greenhouse gases (some global warming potentials are over 10,000 times carbon dioxide) due to their long atmospheric lifetimes and strong infrared adsorption (Ivy et al. Citation2012; LaZerte et al. Citation1953; Leedham Elvidge et al. Citation2018; Ravishankara et al. Citation1993), and fluorinated PIDs containing an oxygen or hydrogen can oxidize in the atmosphere, creating perfluorocarboxylic acids (Ellis et al. Citation2001, Citation2004). The dangers posed by PFAS and fluorinated PIDs have created the need for new control systems and destruction technologies to limit emissions of organofluorine compounds into the atmosphere.
Low temperature (<700°C) PFAS destruction technologies using reactive materials or catalysts are ideal control devices since they can mineralize PFAS with lower energy costs than incineration. PFAS can be reduced by zerovalent metals such as calcium (Billups et al. Citation1984; Klabunde, Jyf, and Key Citation1973), magnesium, or aluminum (Nie, Pisharath, and Hng Citation2020; Ou et al. Citation2021; Zhou et al. Citation2019), resulting in violent reactions that can be used in energetics and solid fuel propellants. Reactions with the oxides of calcium and aluminum, along with aluminum phosphates, have been explored as effective and controllable alternatives (Ng et al. Citation1995; Riedel et al. Citation2021; Takita et al. Citation2002; Wang et al. Citation2011, Citation2015). Alumina-based catalysts have the advantage of acting as true catalysts; the aluminum fluoride bonds can be hydrolyzed back to an aluminum hydroxyl bond, restoring reactivity (Mukhopadhyay et al. Citation2007). Alumina (Han et al. Citation2014; Ng et al. Citation1995; Padhye et al. Citation2017; Rehwoldt et al. Citation2022), metal-doped alumina (e.g., cesium or cobalt) (El-Bahy, Ohnishi, and Ichikawa Citation2003; Han et al. Citation2017; Song et al. Citation2013; Xiu-Feng et al. Citation2005), and aluminum phosphates (Takita et al. Citation1999, Citation2002) have long been studied for their catalytic destruction of perfluorocarbons emitted from silicon chip etching processes (Nakajo et al. Citation2000; Otsuka et al. Citation2005; Rossin Citation2004; Rossin and Feaver Citation2004).
Here, a catalyst developed for perfluorocarbon destruction from silicon etching machines (Brown, Rossin, and Aitchison Citation2001; Rossin Citation2004; Rossin and Feaver Citation2004; Rossin and Maurer Citation2002) was tested to determine if alumina-based catalysts can be applied to the destruction of long chain (>C7) PFAS in the gas-phase. The catalyst was challenged with two gas-phase nonionic fluorosurfactants mainly used in the production of fluoropolymer coatings, 8:2 fluorotelomer alcohol (8:2 FTOH) and N-Ethyl-N-(2-hydroxyethyl)perfluorooctylsulfonamide (NEt-FOSE), structures shown in Figure S1 in the supplementary information (SI). These PFAS were chosen to provide two different classes of PFAS with eight fully fluorinated carbons and for their relatively low boiling points to simplify the evaluation by limiting adsorption. The emissions were sampled and evaluated using thermal desorption-gas chromatography/mass spectrometry (TD-GC/MS), a technique that has been previously used to sample and identify a variety of PFAS, including FTOHs, perfluorocarboxylic acids, perfluoroalkane sulfonamides, perfluoroalkane sulfonamido ethanols, perfluoroiodides, fluorotelomer acrylates, perfluoroalkanes, and perfluoroalkenes (CitationMarkes International Ltd; Riedel et al. Citation2019; Roth et al. Citation2020; Wu and Chang Citation2012). Further development of the catalyst may lead to the production of efficient pollution control devices for PFAS emissions sources.
Materials and methods
Tube furnace setup
PFAS standards were purchased from Synquest Laboratories. Solid phase PFAS, 8:2 FTOH (CAS 678-39-7, 98% purity) and NEt-FOSE (CAS 1691-99-2, >95% purity), were vaporized by placing 50–100 mg into a 2 mL autosampler vial and warming the open vial to a temperature near the PFAS’ melting point, see Figure S2 in the SI for more details. For the lower boiling point 8:2 FTOH a VICI Dynacalibrator perm oven (Santa Clara, CA) was used, while the NEt-FOSE was vaporized in a Lindberg/Blue M Mini-Mite flameless tube furnace (Thermo Scientific, Waltham, MA). The perm oven, Figure S2 section B, was connected by a ¼” fluorinated ethylene propylene (FEP) tube heated to 85°C. Air flow through the system was controlled by a mass flow controller set at 500 mL/min and was bubbled through water before passing over the sample to add 2.3–2.5% water to the air. The moisture was measured with Fourier Transform Infrared spectroscopy (FTIR), setup shown in Figure S2 A. The non-oven portions of the setup, the union connecting the two tube furnaces for the NEt-FOSE experiment (Figure S2 C), the glass tee at the exit of the furnace, and a ¼” stainless steel tube used as a sampling probe, were held at 196°C with heat wrap and a temperature controller (Apex Instruments, Fuquay-Varina, NC). To keep HF from entering the samples, the stainless steel sample probe had about 20 mg of sodium carbonate (Na2CO3) sandwiched between two approximately 8 mg plugs of glass wool inserted into one end of the tube. The vaporized PFAS went into a flameless tube furnace set to temperatures between 200 to 800°C. Samples were collected in 100°C increments from 200 to 800°C for 8:2 FTOH experiments, with a second set of samples collected at 50°C intervals from 200 to 500°C for the catalyst tests. The NEt-FOSE experiments were sampled in 50°C increments from 200 to 500°C for both the thermal only and the catalyst treatments. The 22 mm inner diameter by 45 cm quartz tube in the destruction furnace was run empty, or with a 5.7 cm plug, 20.0 g of Guild Associates, Inc. (Dublin, OH) PFC-200 Catalyst, 8 × 12 mesh sandwiched between quartz wool at the exit end and 1.0 cm, 12 g, of 1 mm zirconia beads and quartz wool at the entrance side. The inert zirconia layer was included to help with gas heating and mixing prior to reaching the catalyst. The catalyst was placed in the last third of the quartz tube, 20 cm from the entrance of the furnace and about 8 cm from the exit of the furnace. The same portion of the catalyst was used for all the experiments. The temperature was measured in the tube furnaces using E-type thermocouples near the PFAS vial, about a third into the volatilization furnace and at the exit side of the catalyst. Blank air samples of the tube furnace with the catalyst were collected before the parent PFAS were introduced to ensure that PIDs did not originate from the catalyst, tubing, or other factors in the experimental setup. Background peaks were not fluorinated nor considered to be PIDs in this experiment.
Sampling and analysis
The sampling and analysis were performed using TD-GC/MS, as described previously (Riedel et al. Citation2021); greater detail, including the use of a Thermo GC-Q-Exactive Orbitrap mass spectrometer used for nontargeted analysis, can be found in the SI. Briefly, two samples were collected for each temperature on multi-bed Universal stainless steel sorbent tubes (Markes International, C3-AXXX-5266) after the Na2CO3 sampling tube at 100 mL/min for 5 minutes using an SKC, Inc. Model 220-5000TC personal sampling pump (Eighty Four, PA). Thermal desorption tube (TD-tube) samples were analyzed on a Markes International TD-100×R thermal desorber coupled to an Agilent 6890N/5977B GC/MSD with a Restek Rtx-VMS GC column. The TD-GC-Orbitrap-MS had similar conditions, but with a Markes International UNITY-xR TD with an ULTRA-xR autosampler and a Restek Rtx-VRX column. Samples were analyzed in electron ionization (EI) mode by TD-GC/MS and both EI and positive chemical ionization (PCI) by TD-GC-Orbitrap-MS.
Destruction efficiency
The temperature dependent destruction efficiencies for the parent molecules and fluorinated PIDs were calculated using Equation 1. The ratio of the molecule’s average peak area, the peak area ratio, in duplicate TD tube samples at the given temperature, PA(T), to the peak’s maximum average area, PAmax, was subtracted from one. For the Figures the standard deviation of the duplicate samples was propagated through the determination of the peak area ratio and the standard deviation was included on the charts as error bars.
Results and discussion
Thermal-only and catalyst treatment experiments
Experiments were conducted with thermal treatment only to determine the efficiency of mineralization for each PFAS without catalyst. Using TD-GC/MS, the 8:2 FTOH peak in EI mode was observed as a single peak at 15.460 min (Figure S3). The thermal-only destruction profile for 8:2 FTOH, , matched that as previously reported in Riedel et al. (Citation2021). Maximum destruction efficiency, ~1.0, was achieved around 700°C. When using the catalyst with 8:2 FTOH, full transformation of the parent compound was observed at all temperatures monitored. A single fluorinated PID, representing 96.7% of the total ion chromatogram area in scan mode was observed at 4.092 min in the 200°C sample from the reaction of the catalyst with the FTOH (see Figure S12 in the SI). At 250°C, the PID at 4.092 min was absent and a different PID at retention time of 4.293 min was observed as the only compound in the chromatogram, representing 100% of the total ion chromatogram area in scan mode (Figure S13). Therefore, this PID’s peak area profile relative to its area at 250°C is plotted in to show the catalyst’s effectiveness. No temperatures lower than 200°C were tested due to the tendency of the long chain PFAS to stick in the tube furnace and tubing at lower temperatures. These results demonstrate that the catalyst is very effective at destroying 8:2 FTOH PIDs even at temperatures as low as 350°C. This destruction temperature is even lower than reported for calcium oxide, a known reactive material for the low temperature destruction of PFAS (Riedel et al. Citation2021).
Figure 1. The destruction profile for 8:2 FTOH with thermal treatment only and the disappearance of a fluorinated PID observed with the catalyst. The catalyst points are based on the peak area of a PID formed by 250°C that replaces the FTOH in the chromatograms.
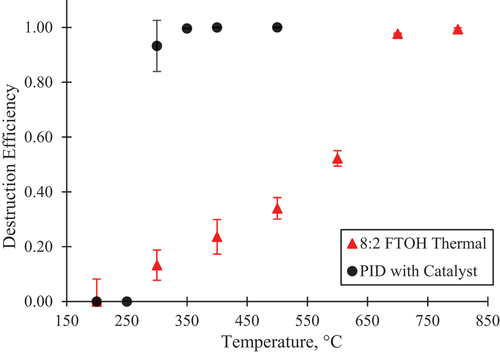
The destruction profiles were obtained for NEt-FOSE, a PFAS with a trisubstituted sulfonamido nitrogen (like many PFAS found in fire-fighting foams). With TD-GC/MS in EI mode, the NEt-FOSE standard produced three main peaks with retention times at 19.928, 20.419, and 21.122 min when loaded directly onto a TD tube. The presence of multiple peaks may indicate the presence of impurities in the purchased standard, or that the parent compound NEt-FOSE experienced some breakdown during the thermal desorption process. Comparing the EI spectra with literature (Roth et al. Citation2020) and standards, the peak at 19.928 min was spectral matched to N-ethyl-perfluorooctane sulfonamide (NEt-FOSA, CAS 4151-50-2), shown in Figure S4, the peak at 20.419 min was confirmed by spectral match and retention time with a standard to be perfluorooctane sulfonamide (PFOSA, CAS 754-91-6), shown in Figures S5 and S6, and the peak at 21.122 min was spectral matched to NEt-FOSE, see Figure S7. All three molecules were included in the destruction efficiency curves for NEt-FOSE in . The thermal-only treatment of these PFAS showed 97+% destruction at 500°C. When the catalyst was used, none of the three parent PFAS were observed in the 200–500°C range. The absence of the three fluorinated peaks in the catalyst experiment at 200°C indicate that the catalyst is reactive and effective at destroying the parent NEt-FOSE.
Figure 2. The thermal only destruction profiles for the molecules in the NEt-FOSE experiment. NEt-FOSA and PFOSA were present as adulterants in the standard and were not separately tested. The NEt-FOSA and PFOSA points at 200°C are overlaid with the NEt-FOSE data point. At 500°C, NEt-FOSE and PFOSA were fully destroyed and only NEt-FOSA was detected. With the catalyst, the parent molecules were no longer present.
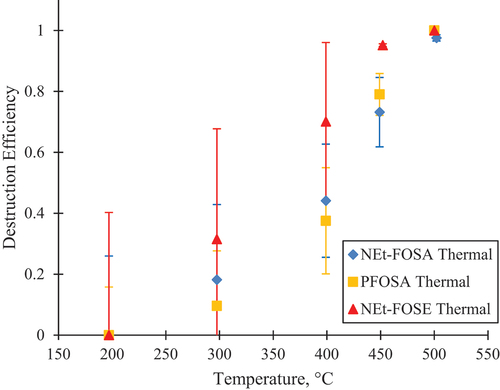
Detection of products of incomplete destruction (PIDs)
During thermal-only and catalyst experiments, fluorinated PIDs were observed. During thermal-only treatment of 8:2 FTOH, four PIDs with CxFy fragment ions such as 69 m/z (CF3), 95 m/z (CF2C2H5O), 119 m/z (CF3CF2) and 131 m/z (CF3CF2C) were detected at multiple temperatures (Figures S8-S11). These same PIDs were not seen in the 8:2 FTOH experiments with catalyst, indicating that they either did not form or were destroyed below 200°C. Two fluorinated PIDs that were not observed in thermal-only treatment were detected in the catalyst experiment at retention times of 4.076 and 4.291 min (Figures S12-S13). These PIDs were no longer observed at low temperatures and were not detected above 200°C and 350°C, respectively. The temperature-dependent disappearance of the PID at 4.291 min is included in .
Several fluorinated PIDs were observed during thermal-only and catalyst treatment of NEt-FOSE. The removal efficiency curves for a PID observed during thermal-only and catalyst treatment are shown in , while the spectra are shown in Figure S14. demonstrates that the fluorinated PID was stable during thermal-only treatment and did not start to breakdown until the temperature was greater than 400°C. With the addition of the catalyst, the PID was defluorinated at lower temperatures, although 500°C was still required to fully destroy the PID.
Figure 3. The thermal-only and catalytic profiles for the PID observed during both treatments of NEt-FOSE. The peak ratio between the largest peak area and the area at the given temperature is shown. The PID had a retention time of 18.97 min and m/z of 541.00109. With catalyst treatment, the PID’s peak area decreases at lower temperatures compared to thermal-only treatment.
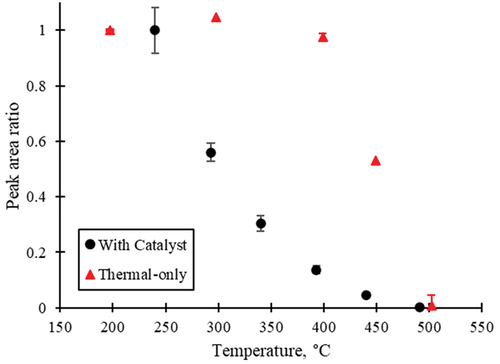
To better characterize PIDs in the NEt-FOSE experiments, the TD tubes collected during the catalyst experiments were also analyzed by TD-GC-QExactive-MS to obtain EI and PCI spectra. Many fluorinated PIDs were observed by TD-GC-QExactive-MS, although it outside the scope of this article to report all non-targeted PIDs. However, the EI spectra for the PID in was matched by retention time to the PCI spectra, and the exact mass for the PID in was determined to be 541.00109 (see Figures S15-S16). The chemical formula for the feature in is likely C11H8F17NO2S; see Figure S17 for the proposed structure. The identification of this PID demonstrates how important it is to monitor the destruction profiles of PIDs in addition to the parent PFAS in thermal destruction experiments. The absence of this PID in the NEt-FOSE standard shows that it was formed through the degradation process and not due to breakdown in the thermal desorber during analysis. With the catalyst, full destruction of PFAS at 200°C would have been assumed due to the absence of the NEt-FOSE compounds found in the standard, even though this PID was not destroyed until 500°C.
Fluorocarbon emissions
The TD-GC/MS methods cannot sample and analyze all types of PFAS. PFAS containing three carbons or less and sulfonates will not be observed with TD-tubes, Perfluorocarboxylic acids (PFCAs) can be observed on TD-tubes, but the potential decarboxylation of the PFCAs during thermal desorption makes PFCAs difficult to discern if potential fluorocarbon or alkene species observed in the chromatograms are from PIDs formed during thermal treatment or PFCAs degrading during the analysis. The 1 H-perfluoroalkanes or 1-perfluoroalkenes formed during the degradation process or from reactions and smaller byproducts down to four carbon long chains can be analyzed with the TD-tubes. The analysis of any smaller species was not done, since the destruction of three carbon and smaller fluorocarbons has been previously reported in patents and literature (Brown, Rossin, and Aitchison Citation2001; Rossin Citation2004; Rossin and Feaver Citation2004; Rossin and Maurer Citation2002), and verified with preliminary tests in this lab. The literature shows that short chain perfluorocarbons, tetrafluoromethane through perfluorobutane, can be destroyed around 650–700°C using the catalyst. Other fluorocarbons, like trifluoromethane, and fluorinated species, such as nitrogen trifluoride, can be destroyed at temperatures below 500°C (Brown, Rossin, and Aitchison Citation2001; Rossin Citation2004). Both thermal treatment and the catalyst can defluorinate fluorochemicals that contain bonds between carbon and non-fluorine atoms at lower temperatures than perfluorinated compounds (Krug et al. Citation2022; Rossin Citation2004). Alumina is known to catalyze elimination reactions and reacts with hydrogens present on a molecule (Adkins and Perkins Citation1925), so it is likely that weaker bonds can increase the PFAS’ reactivity with the alumina based catalyst. It has been shown here that at 500°C most PFAS will be destroyed and the predominant PFAS that could be in the gas stream would be perfluorocarbons (inert, nontoxic greenhouse gases). The destruction of the PFAS and greenhouse gases could occur by heating the catalyst to 700°C to remove over 99% of the perfluorocarbon emissions.
Conclusion
In this study, the link from the destruction of small fluorocarbons with the PFC catalyst and the destruction of vaporized PFAS was shown. The catalyst was effective at lowering the temperature required for destruction of both long chain PFAS with different functional groups and their PIDs. The presence of functional groups or a bond other than a carbon-fluorine bond, lowers the temperature needed to destroy PFAS. A weak non-carbon-fluorine bond provides a place to start the destruction process and an easier place for the catalyst to start to react. The PFAS tested here include carbon-oxygen bonds, carbon-sulfur, and carbon-hydrogen bonds and show that the catalyst can work well with a variety of long chain PFAS with a variety of functional groups. These results demonstrate the utility of the catalyst as a potential PFAS control technology that can eliminate PFAS emissions and show the importance of analyzing for PIDs while evaluating PFAS destruction technologies. Future experiments and development are required to investigate additional PFAS functional groups for their destruction efficiencies and to explore other possible applications in catalytic systems, such as a catalytic thermal oxidizer.
Author contributions
The manuscript was conceptualized, researched, and written through contributions of all authors. All authors have given approval to the final version of the manuscript.
Revised-SI-LowTempDestructionGasPhasePFAS.docx
Download MS Word (2.7 MB)Acknowledgment
Special thanks to Guild Associates, Inc. for providing the PFC-200 catalyst in a special 8 × 12 mesh for these tests. The views expressed in this article are those of the authors and do not necessarily represent the views or policies of the U.S. Environmental Protection Agency. Any mention of trade names, manufacturers or products does not imply an endorsement by the United States Government or the U.S. Environmental Protection Agency. EPA and its employees do not endorse any commercial products, services, or enterprises.
Disclosure statement
No potential conflict of interest was reported by the authors.
Data availability statement
The data that support the findings of this study are available at https://doi.org/10.23719/1527784.
Supplementary material
Supplemental data for this paper can be accessed online at https://doi.org/10.1080/10962247.2023.2210103
Additional information
Funding
Notes on contributors
Erin P. Shields
Erin P. Shields is a Physical Scientist in the U.S. EPA’s Office of Research and Development. His research area involves developing methods to characterize source emissions and to evaluate pollution control technologies.
M. Ariel Geer Wallace
M. Ariel Geer Wallace is a Chemist in the U.S. EPA's Office of Research and Development. Her research is focused on methods development for the sampling and analysis of volatile and semivolatile organic compounds.
References
- Adkins, H., and P.P. Perkins. 1925. Dehydration of alcohols over alumina. J. Am. Chem. Soc. 47 (4):1163–67. doi:10.1021/ja01681a036.
- Ahrens, L. 2011. Polyfluoroalkyl compounds in the aquatic environment: A review of their occurrence and fate. J. Environ. Monitor. 13 (1):20–31. doi:10.1039/C0EM00373E.
- Berg, C., B. Crone, B. Gullett, M. Higuchi, M.J. Krause, P.M. Lemieux, T. Martin, E.P. Shields, E. Struble, E. Thoma, et al. 2022. Developing innovative treatment technologies for PFAS-containing wastes. J. Air Waste Manag. Assoc. 72 (6):1–16. doi:10.1080/10962247.2021.2000903.
- Billups, W.E., J.P. Bell, J.L. Margrave, and R.H. Hauge. 1984. Activation of hexafluoroethane by calcium atoms. J. Fluor. Chem. 26 (2):165–67. doi:10.1016/S0022-1139(00)80920-3.
- Bolan, N., B. Sarkar, Y. Yan, Q. Li, H. Wijesekara, K. Kannan, D.C.W. Tsang, M. Schauerte, J. Bosch, H. Noll, et al. 2021. Remediation of poly- and perfluoroalkyl substances (PFAS) contaminated soils – to mobilize or to immobilize or to degrade? J. Hazard. Mater. 401:123892. doi:10.1016/j.jhazmat.2020.123892.
- Brown, R.S., J.A. Rossin, and K. Aitchison. 2001. Catalytic technology for PFC emissions control. Solid State Technol. 44 (7):189.
- El-Bahy, Z.M., R. Ohnishi, and M. Ichikawa. 2003. Hydrolysis of CF4 over alumina-based binary metal oxide catalysts. Appl. Catal B 40 (2):81–91. doi:10.1016/S0926-3373(02)00143-1.
- Ellis, D.A., S.A. Mabury, J.W. Martin, and D.C. Muir. 2001. Thermolysis of fluoropolymers as a potential source of halogenated organic acids in the environment. Nature 412 (6844):321–24. doi:10.1038/35085548.
- Ellis, D.A., J.W. Martin, A.O. De Silva, S.A. Mabury, M.D. Hurley, M.P. Sulbaek Andersen, and T.J. Wallington. 2004. Degradation of fluorotelomer alcohols: A likely atmospheric source of perfluorinated carboxylic acids. Environ. Sci. Technol. 38 (12):3316–21. doi:10.1021/es049860w.
- Faust, J.A. 2022. PFAS on atmospheric aerosol particles: A review. Environ. Sci. Process Impacts 25 (2):133–50. doi:10.1039/D2EM00002D.
- Freeling, F., M. Scheurer, J. Koschorreck, G. Hoffmann, T.A. Ternes, and K. Nödler. 2022. Levels and temporal trends of trifluoroacetate (TFA) in archived plants: Evidence for increasing emissions of gaseous TFA precursors over the last decades. Environ. Sci. Technol. Lett. 9 (5):400–05. doi:10.1021/acs.estlett.2c00164.
- Ghisi, R., T. Vamerali, and S. Manzetti. 2019. Accumulation of perfluorinated alkyl substances (PFAS) in agricultural plants: A review. Environ. Res. 169:326–41. doi:10.1016/j.envres.2018.10.023.
- Giesy, J.P., and K. Kannan. 2001. Global distribution of perfluorooctane sulfonate in wildlife. Environ. Sci. Technol. 35 (7):1339–42. doi:10.1021/es001834k.
- Goldwhite, H. 1986. The Manhattan project. J. Fluor. Chem. 33 (1–4):109–32. doi:10.1016/S0022-1139(00)85273-2.
- Han, W., Y. Chen, B. Jin, H. Liu, and H. Yu. 2014. Catalytic hydrolysis of trifluoromethane over alumina. Greenhouse Gases Sci. Technol. 4 (1):121–30. doi:10.1002/ghg.1376.
- Han, J.-Y., C.-H. Kim, B. Lee, S.-C. Nam, H.-Y. Jung, H. Lim, K.-Y. Lee, and S.-K. Ryi. 2017. Sorption enhanced catalytic CF4 hydrolysis with a three-stage catalyst-adsorbent reactor. Front. Chem. Sci. Eng. 11 (4):537–44. doi:10.1007/s11705-017-1651-1.
- Haukas, M., U. Berger, H. Hop, B. Gulliksen, and G.W. Gabrielsen. 2007. Bioaccumulation of per- and polyfluorinated alkyl substances (PFAS) in selected species from the barents sea food web. Environ. Pollut. 148 (1):360–71. doi:10.1016/j.envpol.2006.09.021.
- Hercules, D.A., C.A. Parrish, T.S. Sayler, K.T. Tice, S.M. Williams, L.E. Lowery, M.E. Brady, R.B. Coward, J.A. Murphy, T.A. Hey, et al. 2017. Preparation of tetrafluoroethylene from the pyrolysis of pentafluoropropionate salts. J. Fluor. Chem. 196:107–16. doi:10.1016/j.jfluchem.2016.10.004.
- Houtz, E.F., C.P. Higgins, J.A. Field, and D.L. Sedlak. 2013. Persistence of perfluoroalkyl acid precursors in AFFF-impacted groundwater and soil. Environ. Sci. Technol. 47 (15):8187–95. doi:10.1021/es4018877.
- Ivy, D.J., T. Arnold, C.M. Harth, L.P. Steele, J. Mühle, M. Rigby, P.K. Salameh, M. Leist, P.B. Krummel, P.J. Fraser, et al. 2012. Atmospheric histories and growth trends of C4F10, C5F12, C6F14, C7F16 and C8F18. Atmos. Chem. Phys. 12 (9):4313–25. doi:10.5194/acp-12-4313-2012.
- Jahnke, A., L. Ahrens, R. Ebinghaus, and C. Temme. 2007. Urban versus remote air concentrations of fluorotelomer alcohols and other polyfluorinated alkyl substances in Germany. Environ. Sci. Technol. 41 (3):745–52. doi:10.1021/es0619861.
- Klabunde, K.J., L.O.W. Jyf, and M.S. Key. 1973. Metal atom reactions with fluorocarbons. II. Defluorination by calcium atoms. J. Fluor. Chem. 2 (2):207–09. doi:10.1016/S0022-1139(00)83508-3.
- Krug, J.D., P.M. Lemieux, C.W. Lee, J.V. Ryan, P.H. Kariher, E.P. Shields, L.C. Wickersham, M.K. Denison, K.A. Davis, D.A. Swensen, et al. 2022. Combustion of C1 and C2 PFAS: Kinetic modeling and experiments. J. Air Waste Manag. Assoc. 72 (3):256–70. doi:10.1080/10962247.2021.2021317.
- LaZerte, J.D., L.J. Hals, T.S. Reid, and G.H. Smith. 1953. Pyrolyses of the salts of the perfluoro carboxylic acids1. J. Am. Chem. Soc. 75 (18):4525–28. doi:10.1021/ja01114a040.
- Leedham Elvidge, E., H. Bönisch, C.A.M. Brenninkmeijer, A. Engel, P.J. Fraser, E. Gallacher, R. Langenfelds, J. Mühle, D.E. Oram, E.A. Ray, et al. 2018. Evaluation of stratospheric age of air from CF4, C2F6, C3F8, CHF3, HFC-125, HFC-227ea and SF6; implications for the calculations of halocarbon lifetimes, fractional release factors and ozone depletion potentials. Atmos. Chem. Phys. 18(5):3369–85. doi:10.5194/acp-18-3369-2018.
- Lemal, D.M. 2004. Perspective on fluorocarbon chemistry. J. Org. Chem. 69 (1):1–11. doi:10.1021/jo0302556.
- Li, J., J. Sun, and P. Li. 2022. Exposure routes, bioaccumulation and toxic effects of per- and polyfluoroalkyl substances (PFASs) on plants: A critical review. Environ. Int. 158:106891. doi:10.1016/j.envint.2021.106891.
- Markes International Ltd. Analysis of trace per- and polyfluorinated organic vapours in air using cryogen-free thermal desorption and gas chromatography–mass spectrometry. Appl. Note 158:1–8. https://markes.com/content-hub/application-notes/application-note-1582022.
- Markes International Ltd. Untargeted screening of volatile per- and polyfluoroalkyl substances (PFAS) released during the application of aqueous film-forming foam (AFFF). Appl. Note 153:1–4. https://markes.com/content-hub/application-notes/application-note-1532021.
- McCord, J., and M. Strynar. 2019. Identification of per- and polyfluoroalkyl substances in the Cape Fear River by high resolution mass spectrometry and nontargeted screening. Environ. Sci. Technol. 53 (9):4717–27. doi:10.1021/acs.est.8b06017.
- McDonough, C.A., W. Li, H.N. Bischel, A.O. De Silva, and J.C. DeWitt. 2022. Widening the lens on PFASs: Direct human exposure to perfluoroalkyl acid precursors (pre-PFAAs). Environ. Sci. Technol. 56 (10):6004–13. doi:10.1021/acs.est.2c00254.
- Milley, S.A., I. Koch, P. Fortin, J. Archer, D. Reynolds, and K.P. Weber. 2018. Estimating the number of airports potentially contaminated with perfluoroalkyl and polyfluoroalkyl substances from aqueous film forming foam: A Canadian example. J. Environ. Manage. 222:122–31. doi:10.1016/j.jenvman.2018.05.028.
- Mukhopadhyay, S., C.L. Bailey, A. Wander, B.G. Searle, C.A. Muryn, S.L.M. Schroeder, R. Lindsay, N. Weiher, and N.M. Harrison. 2007. Stability of the AlF3 surface in H2O and HF environments: An investigation using hybrid density functional theory and atomistic thermodynamics. Surf. Sci. 601 (18):4433–37. doi:10.1016/j.susc.2007.04.231.
- Nakajo, T., H. Ohno, T. Ohi, Y. Takita, D.K.K. Showa assignee. 2000. Catalytic decomposition of perfluoro-compound. United States patent US 6023007, February 8.
- Ng, L.M., E. Lyth, M.V. Zeller, and D.L. Boyd. 1995. Surface chemistry of perfluoro ethers: An infrared study of the thermal decomposition of (C2F5)2O on Al2O3. Langmuir 11 (1):127–35. doi:10.1021/la00001a024.
- Nie, H., S. Pisharath, and H.H. Hng. 2020. Combustion of fluoropolymer coated Al and Al–Mg alloy powders. Combust. Flame 220:394–406. doi:10.1016/j.combustflame.2020.07.016.
- O’Hagan, D. 2008. Understanding organofluorine chemistry. An introduction to the C-F bond. Chem. Soc. Rev. 37 (2):308–19. doi:10.1039/B711844A.
- Okazoe, T. 2009. Overview on the history of organofluorine chemistry from the viewpoint of material industry. Proc. Jpn. Acad. Ser. B Phys. Biol. Sci. 85 (8):276–89. doi:10.2183/pjab.85.276.
- Otsuka, K., Y. Nawa, T. Ikeda, K. Ochi, inventors; Japan Pionics Co., Ltd., assignee. 2005. Decompositionally treating agent and decompositionally treating method for fluorocarbons. United States patent US 6960552, November 1.
- Ou, Y., Q. Jiao, N. Li, S. Yan, and R. Yang. 2021. Pyrolysis of ammonium perfluorooctanoate (APFO) and its interaction with nano-aluminum. Chem. Eng. J. 403:403. doi:10.1016/j.cej.2020.126367.
- Padhye, R., A.J.A. Aquino, D. Tunega, and M.L. Pantoya. 2017. Fluorination of an alumina surface: Modeling aluminum-fluorine reaction mechanisms. ACS Appl. Mater. Interfaces 9 (28):24290–97. doi:10.1021/acsami.7b05372.
- Pan, Y., H. Zhang, Q. Cui, N. Sheng, L.W.Y. Yeung, Y. Guo, Y. Sun, and J. Dai. 2017. First report on the occurrence and bioaccumulation of hexafluoropropylene oxide trimer acid: An emerging concern. Environ. Sci. Technol. 51 (17):9553–60. doi:10.1021/acs.est.7b02259.
- Ravishankara, A.R., S. Solomon, A.A. Turnipseed, and R.F. Warren. 1993. Atmospheric lifetimes of long-lived halogenated species. Science 259 (5092):194–99. doi:10.1126/science.259.5092.194.
- Rehwoldt, M.C., Y. Wang, F. Xu, P. Ghildiyal, and M.R. Zachariah. 2022. High-temperature interactions of metal oxides and a PVDF binder. ACS Appl. Mater. Interfaces 14 (7):8938–46. doi:10.1021/acsami.1c20938.
- Riedel, T.P., J.R. Lang, M.J. Strynar, A.B. Lindstrom, and J.H. Offenberg. 2019. Gas-phase detection of fluorotelomer alcohols and other oxygenated per- and polyfluoroalkyl substances by chemical ionization mass spectrometry. Environ. Sci. Technol. Lett. 6 (5):289–93. doi:10.1021/acs.estlett.9b00196.
- Riedel, T.P., M.A.G. Wallace, E.P. Shields, J.V. Ryan, C.W. Lee, and W.P. Linak. 2021. Low temperature thermal treatment of gas-phase fluorotelomer alcohols by calcium oxide. Chemosphere 272:129859. doi:10.1016/j.chemosphere.2021.129859.
- Rossin, J.A., inventor; Guild Associates, Inc., assignee. 2004. Catalyst composition and method of controlling PFC and HFC emissions. United States patent US 6676913, January 13.
- Rossin, J.A., W.B. Feaver, inventors; Guild Associates, Inc., assignee. 2004. Catalytic processes for the reduction of perfluorinated compounds and hydrofluorocarbons. United States patent US 6673326, January 6.
- Rossin, J.A., S.M. Maurer, inventors; Guild Associates, Inc., assignee. 2002. Catalytic process for the decomposition of perfluoroalkanes. United States patent US 6426443, July 30.
- Roth, J., I. Abusallout, T. Hill, C. Holton, U. Thapa, and D. Hanigan. 2020. Release of volatile per- and polyfluoroalkyl substances from aqueous film-forming foam. Environ. Sci. Technol. Lett. 7 (3):164–70. doi:10.1021/acs.estlett.0c00052.
- Seay, B.A., K. Dasu, I.C. MacGregor, M.P. Austin, R.T. Krile, A.J. Frank, G.A. Fenton, D.R. Heiss, R.J. Williamson, and S. Buehler. 2023. Per- and polyfluoroalkyl substances fate and transport at a wastewater treatment plant with a collocated sewage sludge incinerator. Sci. Total Environ. 874:162357. doi:10.1016/j.scitotenv.2023.162357.
- Song, J.-Y., S.-H. Chung, M.-S. Kim, S. M-G, Y.-H. Lee, K.-Y. Lee, and J.-S. Kim. 2013. The catalytic decomposition of CF4 over Ce/Al2O3 modified by a cerium sulfate precursor. J. Mol. Catal. A Chem. 370:50–55. doi:10.1016/j.molcata.2012.12.011.
- Stockin, K.A., S. Yi, G.L. Northcott, E.L. Betty, G.E. Machovsky-Capuska, B. Jones, M.R. Perrott, R.J. Law, A. Rumsby, M.A. Thelen, et al. 2021. Per- and polyfluoroalkyl substances (PFAS), trace elements and life history parameters of mass-stranded common dolphins (Delphinus delphis) in New Zealand. Mar. Pollut. Bull. 173 (Pt A):112896. doi:10.1016/j.marpolbul.2021.112896.
- Sunderland, E.M., X.C. Hu, C. Dassuncao, A.K. Tokranov, C.C. Wagner, and J.G. Allen. 2019. A review of the pathways of human exposure to poly- and perfluoroalkyl substances (PFASs) and present understanding of health effects. J. Exposure Sci. Environ. Epidemiol. 29 (2):131–47. doi:10.1038/s41370-018-0094-1.
- Takita, Y., M. Ninomiya, H. Miyake, H. Wakamatsu, Y. Yoshinaga, and T. Ishihara. 1999. Catalytic decomposition of perfluorocarbons Part II. Decomposition of CF4 over AlPO4-rare earth phosphate catalysts. Phys. Chem. Chem. Phys. 1 (18):4501–04. doi:10.1039/a904311j.
- Takita, Y., T. Tanabe, M. Ito, M. Ogura, T. Muraya, S. Yasuda, H. Nishiguchi, and T. Ishihara. 2002. Decomposition of CH2FCF3 (134a) over metal phosphate catalysts. Ind. Eng. Chem. Res. 41 (11):2585–90. doi:10.1021/ie0106229.
- Thompson, K.A., S. Mortazavian, D.J. Gonzalez, C. Bott, J. Hooper, C.E. Schaefer, and E.R.V. Dickenson. 2022. Poly- and perfluoroalkyl substances in municipal wastewater treatment plants in the United States: Seasonal patterns and meta-analysis of long-term trends and average concentrations. ACS ES&T Water 2 (5):690–700. doi:10.1021/acsestwater.1c00377.
- Tsang, W., D.R. Burgess, and V. Babushok. 1998. On the incinerability of highly fluorinated organic compounds. Combust. Sci. Technol. 139 (1):385–402. doi:10.1080/00102209808952095.
- U.S. EPA. 2021. PFAS strategic roadmap: EPA’s commitments to action 2021–2024. U.S. EPA document EPA-100-K-21-002. https://www.epa.gov/system/files/documents/2021-10/pfas-roadmap_final-508.pdf.
- Wang, J., Z. Lin, X. He, M. Song, P. Westerhoff, K. Doudrick, and D. Hanigan. 2022. Critical review of thermal decomposition of per- and polyfluoroalkyl substances: Mechanisms and implications for thermal treatment processes. Environ. Sci. Technol 56 (9): 5355–5370 doi:10.1021/acs.est.2c02251.
- Wang, F., X. Lu, X.Y. Li, and K. Shih. 2015. Effectiveness and mechanisms of defluorination of perfluorinated alkyl substances by calcium compounds during waste thermal treatment. Environ. Sci. Technol. 49 (9):5672–80. doi:10.1021/es506234b.
- Wang, F., X. Lu, K. Shih, and C. Liu. 2011. Influence of calcium hydroxide on the fate of perfluorooctanesulfonate under thermal conditions. J. Hazard. Mater. 192 (3):1067–71. doi:10.1016/j.jhazmat.2011.06.009.
- Wu, Y., and V.W.C. Chang. 2012. Development of analysis of volatile polyfluorinated alkyl substances in indoor air using thermal desorption-gas chromatography–mass spectrometry. J. Chromatogr. A 1238:114–20. doi:10.1016/j.chroma.2012.03.053.
- Xiu-Feng, X., J.Y. Jeon, M.H. Choi, H.Y. Kim, W.C. Choi, and Y.-K. Park. 2005. A strategy to protect Al2O3-based PFC decomposition catalyst from deactivation. Chem. Lett. 34 (3):364–65. doi:10.1246/cl.2005.364.
- Zhao, H., L. Yang, X. Yang, and S. Zhao. 2022. Behaviors of 6: 2 fluorotelomer sulfonamide alkylbetaine (6: 2 FTAB) in wheat seedlings: Bioaccumulation, biotransformation and ecotoxicity. Ecotoxicol. Environ. Safety 238:113585. doi:10.1016/j.ecoenv.2022.113585.
- Zhou, X.-Y., F. Xiao, R.-J. Yang, F.-L. Huang, and J.-M. Li. 2019. Investigation of the ignition and combustion of compressed aluminum/polytetrafluoroethylene bulk composites. J. Therm. Anal. Calorim. 139 (5):3013–21. doi:10.1007/s10973-019-08662-2.