Abstract
Cohesin is a central architectural element of chromosomes that regulates numerous DNA-based events. The complex holds sister chromatids together until anaphase onset and organizes individual chromosomal DNAs into loops and self-associating domains. Purified cohesin diffuses along DNA in an ATP-independent manner but can be propelled by transcribing RNA polymerase. In conjunction with a cofactor, the complex also extrudes DNA loops in an ATP-dependent manner. In this study we examine transcription-driven translocation of cohesin under various conditions in yeast. To this end, obstacles of increasing size were tethered to DNA to act as roadblocks to complexes mobilized by an inducible gene. The obstacles were built from a GFP-lacI core fused to one or more mCherries. A chimera with four mCherries blocked cohesin passage in late G1. During M phase, the threshold barrier depended on the state of cohesion: non-cohesive complexes were also blocked by four mCherries whereas cohesive complexes were blocked by as few as three mCherries. Furthermore cohesive complexes that were stalled at obstacles, in turn, blocked the passage of non-cohesive complexes. That synthetic barriers capture mobilized cohesin demonstrates that transcription-driven complexes translocate processively in vivo. Together, this study reveals unexplored limitations to cohesin movement on chromosomes.
INTRODUCTION
Cohesin is a central architectural element of chromosomes that functions by bringing distant DNA sites together.Citation1 The protein complex was first characterized for its role in sister chromatid cohesion. Cohesin is now known to participate in most if not all fundamental chromosomal processes, including transcription, replication and repair. Recent work has shown that the complex also organizes chromosomes into large DNA loops that contribute to the segmentation of the genome into self-associating domains. Mis-regulation of cohesin has been identified as the cause of certain developmental diseases known collectively as cohesinopathies.Citation2 Mutations in cohesin subunits have also been associated with human cancers.
The evolutionarily conserved core cohesin complex contains two SMC subunits (Smc1 and Smc3) and an α kleisin subunit Mcd1 (also known as Scc1/Rad21). The proteins assemble into a ring that can embrace DNA topologically, most notably when engaged in sister chromatid cohesion.Citation3,Citation4 In yeast, the complex accumulates densely across tens of kilobases surrounding centromeres.Citation5,Citation6 Along chromosome arms, the complex binds between convergently transcribed genes. Chromosome conformation capture (3 C) techniques have shown that such sites participate both in sister chromatid cohesion and chromosomal loop formation in yeast.Citation7–10 Cohesin performs these same architectural functions in higher eukaryotes.Citation11–13
Cohesin loading onto yeast chromosomes by Scc2 (NIPBL in higher eukaryotes) begins each cell cycle in late G1. Initially, the interaction is dynamic. The complex cycles on and off DNA because loading is balanced by an unloading factor known as Wpl1 or Rad61 (WAPL in higher eukaryotes). During S phase, Eco1 (also known as Ctf7) halts cohesin loss by acetylating the Smc3 cohesin subunit, which antagonizes Wpl1 (reviewed in UhlmannCitation14). Eco1 travels with the DNA replication fork, presumably to couple the stabilization of cohesive complexes directly with the creation of sister chromatids.Citation15
A second dynamic property of cohesin is the ability to redistribute on chromosomes. Cohesin accumulates at sites that are often great distances from where the complex initially loads.Citation16 Initially, RNA polymerase was seen as the central driver of repositioning because cohesin on dormant or weakly transcribed genes was relocated downstream upon transcriptional activation.Citation5,Citation6,Citation17 Numerous observations suggested that cohesin translocates processively: (1) at low temperature, gene induction moved the complex to intermediate positions between initial and final binding sites;Citation18 (2) cohesin arrived at new sites following transcription even in the absence of the Scc2 loader;Citation18,Citation19 (3) Transcription by an ectopic polymerase caused cohesin mobilization over the transcribed region.Citation20 Indeed, cohesin was shown to diffuse along DNA in vitro and translocating enzymes, like RNA polymerase, were shown to bias the directionality of movement.Citation20–22 More recent biochemical work showed that cohesin, in combination with NIPBL/Scc2, possesses an intrinsic motor activity that enables processive translocation.Citation23,Citation24 Importantly, these complexes extruded DNA loops, a process that is thought to form the large chromosomal loops that typify genomes.Citation25–27 In support of the loop extrusion model, the size and distribution of chromosomal loops are altered by factors that influence the residence time of cohesin on or engagement with chromatin.Citation7,Citation8,Citation28–31,Citation58 Consistent with processivity, loop expansion is blocked or delayed by CTCF and other natural DNA-binding proteins.Citation1,Citation32 RNA polymerase is a mobile barrier to loop extrusion with the ability to push loop extruding complexes during transcription (see,Citation33 and references therein).
We recently described the impact of transcription on cohesion using a model cohesin-bound gene in yeast.Citation34 Upon induction of the gene, cohesion of the locus was lost. If, however, the locus was first converted to an extrachromosomal circle, cohesion persisted. The results are most readily explained by redistribution of topologically-bound cohesin by processive translocation because such complexes cannot migrate off circularized DNA. In the present study, the properties of transcription-propelled complexes were further studied by measuring their ability to bypass engineered obstacles of increasing size. This work provides direct evidence that transcription-driven cohesin translocates processively on DNA in vivo. The data reveal that the fate of encounters with barriers depends on the cohesive state of the complex: cohesive complexes are blocked by a barrier not large enough to block non-cohesive complexes. Furthermore, the data show that stalled cohesive complexes block passage of non-cohesive complexes.
RESULTS
Nuclear membrane tethering of DNA blocks cohesin translocation
Our previous study found that DNA circularization, as well as downstream convergently oriented genes, prevented transcription-driven cohesion loss.Citation34 As stated above, we inferred that cohesin was mobile but trapped on the circles. Downstream convergent genes, on the other hand, were viewed simply as barriers to cohesin passage. Intriguingly, DNA-bound lac repressors did not block escape of cohesin (). We hypothesized that the bacterial protein (lacI fused to GFP) was smaller than the opening of the complex through which DNA passes. If cohesin truly redistributes by translocation in vivo, then DNA-bound proteins of sufficient size should block passage of the complex. To begin to explore this concept, we tethered an array of lac operators to the nuclear envelope, thereby creating an obstacle of essentially infinite size (). We used a GFP-lacI chimera linked to FFAT, a decapeptide motif that associates with proteins in the nuclear envelope and endoplasmic reticulum. shows that the fluorescent dot of DNA-bound GFP-FFAT-lacI localized to the nuclear periphery, in agreement with previous work.Citation36 In contrast, GFP-lacI formed perinuclear foci with a much lower frequency that was consistent with random nuclear localization. These data indicate that GFP-FFAT-lacI tethers our locus of interest to the nuclear envelope.
FIG 1 Anchoring DNA to the inner nuclear membrane blocks transcription-driven translocation of cohesin. (A) Schematic of cohesin translocation and blockage by membrane anchorage. Only one GFP-lacI is shown for clarity. NE = nuclear envelope. (B) Nuclear envelope tethering of DNA by GFP-FFAT-lacI. DNA circles containing an array of lac operators were generated as described in and perinuclear localization was determined for 121 G1 phase cells of strains MRG7663.1 and MRG7664, as described in Gartenberg et al.Citation35 The percentage of cells with a GFP dot in the zone closest to the nuclear envelope (the outermost of three concentric zones of equal surface area) is reported. A dashed red line identifies the expected value for random localization (33% in each zone). A representative image of MRG7664 cells is shown with SS-mCherry-HDEL tagging the NE in red. (C) Schematic of the locus used to test translocation. Binding of Mcd1-TAP was evaluated at a site labelled 5’lacO that lies immediately upstream of an array of roughly 128 lac operators. (D) Experimental flow chart. Cells were arrested in M phase by Cdc20 depletion and then URA3 transcription was induced by switching to media lacking uracil. (E) ChIP-qPCR of Mcd1-TAP. Strains MSB114 and MRG6947 were used. Values were normalized to a cohesin bound site (549.7 kb on Chr IV, see Materials and Methods). P values for pairwise Student’s t tests are presented relative to benchmark sample (designated with a dash). ns = not significant. n = number of independent biological replicates. trxn = transcription.
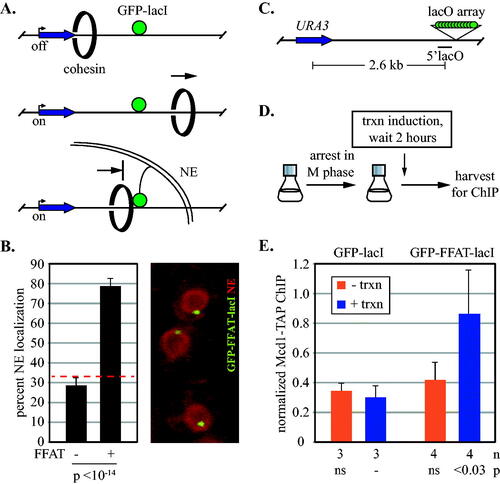
Translocation assays were performed at a chromosomal construct () that contains an array of lac operators downstream of URA3, a weakly expressed gene that can be induced 3–5 fold by withholding uracil. Previous work showed that cohesive complexes associate with the uninduced gene until transcriptional activation triggers their departure, presumably due to the increased density of transiting RNA polymerases. Cohesin levels at URA3 do not diminish, however, because new non-cohesive complexes reload during the experimental intervalCitation34 (see example in Fig. S1B). Chromatin immunoprecipitation coupled with quantitative real-time PCR (ChIP-qPCR) was used to monitor arrival of cohesin at a downstream site immediately preceding the lac operator array (hereafter referred to as the 5’lacO site). A TAP epitope affixed to Mcd1 was used for immunoprecipitations.
URA3 transcription was induced after cells were arrested in M phase by depletion of Cdc20 (). When GFP-lacI was expressed, cohesin at 5’lacO was low both before and after URA3 induction (). The result is consistent with the inability of GFP-lacI to block translocating cohesin complexes. Expression of GFP-FFAT-lacI, on the other hand, increased the level of cohesin at 5’lacO following transcription. This finding serves as a proof-of-principle that large immobile objects block translocation of cohesin complexes in yeast. In B. subtilis, tethering a DNA-bound repressor to the plasma membrane similarly blocked translocation of bacterial SMC proteins.Citation37
Large DNA-bound proteins block cohesin translocation
To determine if large proteins could similarly block cohesin passage, a series of obstacles was generated by fusing increasing numbers of mCherry to GFP-lacI (). This red fluorescent protein is ideally suited as a size standard because it has a compact monomeric structure unlike its tetrameric progenitor, DsRed.Citation39 The GFP-lacI-mCherry chimeras bind lac operator arrays, albeit with reduced efficiency compared to GFP-lacI alone, as evidenced by weaker GFP foci and higher background nuclear GFP fluorescence (Fig. S2).
FIG 2 Synthetic barriers of sufficient size block transcription-driven translocation of cohesin. (A) Schematic of cohesin translocation and blockage by GFP-lacI chimeras. (B) Organization of the GFP-lacI chimeras with mCherries. (C) Expression of GFP-lacI chimeras. Strains MSB174, MSB179, MSB180, MSB181 and MRG7102 were used. Chimeras were immunoblotted with an antibody to GFP. The lower molecular weight bands (marked with *) likely arise from partial proteolytic cleavage of mCherry.Citation38 (D) ChIP-qPCR of Mcd1-TAP. Cohesin binding at the 5’lacO site was evaluated in strains MSB114, MRG6810, MRG6812, MSB165.1 and MRG7060 according to the growth protocol shown in . (E) ChIP-qPCR of Mcd1-TAP in a strains with an array of eight lac operators. Strains PMK11 and PMK16 were used.
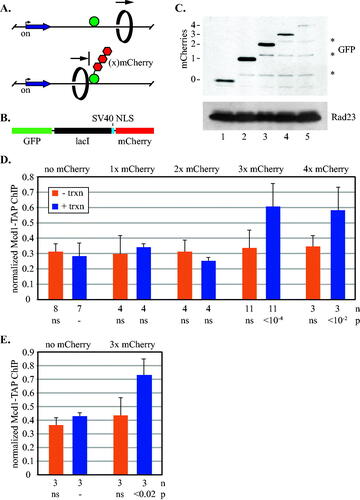
Accumulation of cohesin at 5’lacO was measured in M phase-arrested cells as in . In the absence of URA3 induction, cohesin levels at 5’lacO were low for all of the GFP-lacI chimeras (). When URA3 was induced following M phase arrest, by contrast, accumulation of cohesin at 5’lacO depended on the size of the GFP-lacI chimera. When GFP-lacI was fused to one or two mCherries or none at all, cohesin levels did not rise above the uninduced controls. By contrast, cohesin was found at 5’lacO when GFP-lacI was fused to three or four mCherries.
A more thorough analysis of the cohesin distribution around the locus is shown in Fig. S1. In the three mCherry strain, cohesin abundance increased only at sites immediately preceding the lac operator array, as expected for complexes travelling from the induced gene into a downstream roadblock. Conversely, little change occurred with GFP-lacI alone, including at sites tested downstream of the array. That secondary accumulation sites were not found may indicate that the complex distributes throughout the region in the absence of a single strong barrier. To be certain that an aberrant property of mCherry was not responsible for 5’lacO accumulation, the experiment was repeated with a chimera bearing three copies of monomeric yeGFP. Similar results were obtained (Fig. S3). To test whether the unusually long size of the array was a contributing factor (roughly 128 operators within 5 kb), the experiment was repeated with a short array (eight lac operators within just 300 bp). Similar results were obtained (). Taken together, these data indicate that non-yeast proteins of sufficient size block passage of cohesin complexes mobilized by transcription.
Trapped cohesin complexes remain cohesive
To determine whether the complexes at 5’lacO hold sister chromatids together, we evaluated cohesion of the chromosomal domain. In our strains, target sites for the R site-specific recombinase flank the entire region (). Expression of the recombinase during an M phase arrest generates a pair of DNA circles, one from each sister chromatid. The GFP-lacI chimeras yield a single dot of fluorescence if the DNA circles are cohesed whereas a pair of dots appears if they are not (Fig. S2). Cells were arrested in M phase and then URA3 transcription was induced before recombination (). In the uninduced controls, cohesion was observed in all strains due to the presence of cohesive cohesin on URA3 (,Citation34). When transcription was induced, cohesion was greatly reduced in strains with chimeras bearing one or two mCherries or none at all. By contrast, maximal cohesion persisted when transcription was induced in strains expressing chimeras with three and four mCherries. These results indicate that DNA-bound chimeras equal to or larger than three mCherries block passage of translocating cohesive cohesin. We conclude that at least some of the trapped cohesin complexes detected by ChIP-qPCR in are engaged in sister chromatid cohesion.
FIG 3 Synthetic barriers of sufficient size block transcription-driven translocation of cohesive cohesin. (A) An assay for cohesion at URA3. Site specific recombination in M phase converts the GFP-tagged locus into a pair of DNA circles that are visualized by fluorescence microscopy. Half-filled boxes represent RS target sites for the inducible R recombinase. (B) Experimental flow chart. Transcription was induced after M phase arrest and then DNA circles were formed by galactose-induced expression of the R recombinase. (C) Cohesion analysis of DNA circles. Strains MSB35.2, MRG6806, MRG6808, MSB167 and MRG7060 were used. P values for pairwise χ2 tests evaluate the impact of transcriptional induction for each strain. Error bars represent the standard error of proportion. n = the number of cells examined.
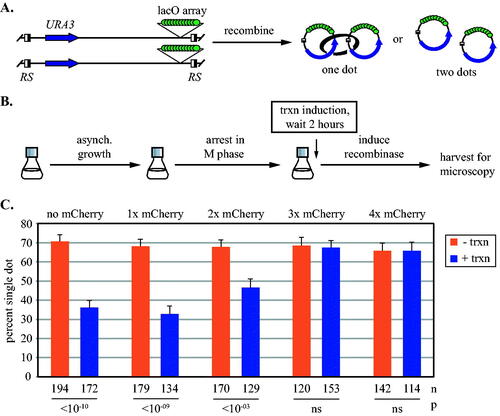
A larger barrier is required to block cohesin translocation in late G1 phase
The studies above examined translocation of cohesin on newly replicated chromatids in M phase-arrested cells. To study translocation on unreplicated chromatin, cells were arrested in late G1 by expressing a non-degradable allele of Sic1 (Sic1(4m)) that prevents S phase entry by stably inhibiting Cdk1.Citation40 By definition, the chromatin-bound complexes at this stage of the cell cycle are not cohesive. The steps of the growth protocol are depicted in and validation of the growth arrest is shown in Fig. S4. Under these conditions, transcriptional induction caused accumulation of cohesin at 5’lacO only in a strain expressing the four mCherry barrier. In strains expressing three mCherries or GFP-lacI alone, cohesin accumulation matched the uninduced controls (). Analysis of a second site immediately adjacent to 5’lacO confirmed the new size threshold (Fig. S5A). Thus, barriers of different size are required to block cohesin at different stages of the cell cycle. We infer that an event during S phase affects translocation of cohesin past obstacles.
FIG 4 Synthetic barriers to cohesin translocation before and after S phase. (A) Experimental flow chart. Expression of the stable Cdk1 inhibitor Sic1(4m)-AID* was induced during an alpha factor-mediated G1 arrest. Release from the arrest caused a subsequent arrest in late G1. The AID* epitope on Sic1(4m) permitted auxin-mediated destruction of the protein, allowing resumption of growth that was arrested in the following M phase by Cdc20 depletion. Transcription was induced as indicated. Details are provided in Materials and Methods. (B) Barriers to translocation in late G1-arrested cells. ChIP-qPCR of Mcd1-TAP at 5’lacO was performed in strains MRG7027, MRG7026 and MRG7059. URA3 transcription was induced after late G1 arrest. The results were validated at a second site immediately adjacent to 5’lacO in Fig. S5A. (C) Barriers to translocation in cells progressing from G1 to M phase. URA3 was induced simultaneously with release from alpha factor arrest, thereby yielding activated transcription during late G1 arrest. The transcriptional induction was maintained for the duration of the experiment when cells were released from late G1 and rearrested in M phase. Strains MRG7033, MRG7032 and MRG7282 were used.
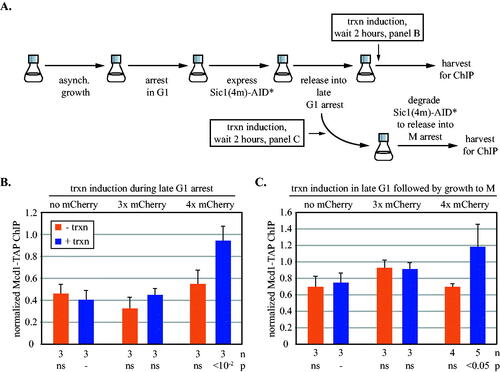
FIG 5 Transcription-driven translocation of cohesin loaded in M phase. (A) Experimental flow chart. All strains possessed a temperature-sensitive mcd1-S525N allele, as well as an ectopic, galactose inducible TAP-tagged MCD1 allele, which was expressed after cells were grown into M phase arrest at permissive temperature. Cultures were then either shifted to 37oC (panel B) or maintained at 25oC (panels C and D) before inducing URA3 transcription. See Materials and Methods for details. ChIP-qPCR was used to monitor Mcd1-TAP at 5’lacO in strains MRG7652, MRG7653 and MRG7654 (panels B and C), and mcd1-S525N-10xFLAG in strains MSB7666, MSB7667 and MSB7668 (panel D). All results were validated at a second site immediately adjacent to 5’lacO in Fig. S5B to S5D.
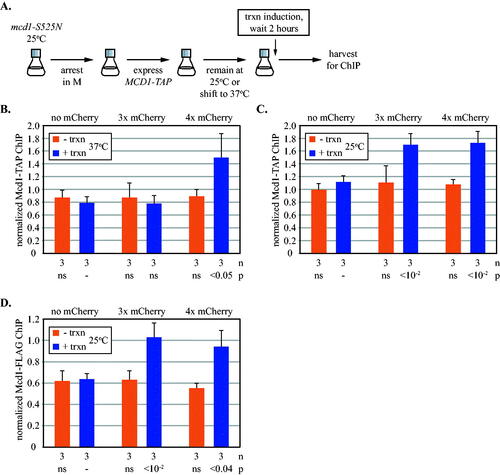
Translocation of complexes that arrive after DNA replication
Cohesin loads onto chromosomes from late G1 to anaphase. Complexes that arrive after DNA replication are not cohesive, do not acquire S phase-dependent acetylation and are subject to turnover by Wpl1.Citation15,Citation41–44 To gain a more complete picture of cohesin dynamics at URA3, transcription was induced in cells approaching a late G1 arrest and then the cultures were released for growth into a subsequent M phase arrest. Monitoring cohesin at the end of this protocol thus reports on complexes that load and translocate before S phase, as well as those that load and translocate after S phase. Complexes may also arrive during replication of the gene but this interval is likely too short to affect the results. The novel arrest-and-release procedure was achieved with an AID degron affixed to “non-degradable” Sic1(4m). Adding the auxin analog 1-naphthaleneacetic acid triggers destruction of Sic(4m) and resumption of growth, which was arrested in M phase by Cdc20 depletion ( and Fig. S4). shows that when URA3 transcription was induced, cohesin only accumulated at 5’lacO in the strain expressing the four mCherry obstacle. That the barrier limit did not revert to three mCherries indicates that late arriving, non-cohesive cohesin was not blocked by the smaller barrier.
To examine late arriving complexes exclusively, TAP-tagged Mcd1 was expressed from an ectopic locus only after M phase arrest (). The endogenous MCD1 gene in these strains was replaced with an untagged temperature sensitive allele mcd1-S525N (also known as scc1-73).Citation45 In a first iteration of the experiment, cells were shifted to non-permissive temperature to disassemble pre-existing cohesin after expressing new Mcd1-TAP in M phase. Subsequent induction of URA3 led to accumulation of TAP-tagged cohesin at only the four mCherry obstacle ( and Fig. S5B). These data are consistent with late loading non-cohesive complexes translocating processively on only a single chromatid even though both are present. In a second iteration of the experiment, cells were maintained at the permissive temperature to preserve the untagged cohesive cohesin that loaded on schedule. In this case, the late loaded cohesin accumulated at both the three and four mCherry barriers upon induction of transcription ( and Fig. S5C). The results indicate that stalled cohesive cohesin blocks the passage of non-cohesive complexes that would otherwise traverse the smaller barrier. As a final control, Mcd1-S525N was tagged with a FLAG epitope to monitor translocation of cohesive cohesin at permissive temperature in this protocol. As expected, the tagged protein was found at both the three and four mCherries barriers ( and Fig. S5D). Thus, cohesin complexes that load naturally and become cohesive during S phase block translocation of non-cohesive complexes.
Wpl1 and Eco1 modulate cohesin’s encounters with barriers
We next tested the influence of regulators of cohesin, Eco1 and Wpl1. The proteins were depleted by auxin inducible degradation during a G1 arrest and then cells were grown to an arrest in the subsequent M phase (). Induction of transcription at this stage thus measures the impact of regulator loss from G1 through M. Clearance of the AID-tagged proteins is shown in Fig. S6A to S6C. Transcriptional regulation of URA3 was unchanged by the depletions (Fig. S6D and Fig. S7B).
FIG 6 The impact of cohesin regulators on transcription-driven translocation past synthetic barriers. (A) Experimental flow chart. Strains with 3xmAID-tagged proteins and a no tag control were arrested in G1 with alpha factor and then treated with 100 µM NAA before release into M arrest when URA3 transcription was induced. See Materials and Methods for details. (B) Impact of cohesin regulators on cohesion of DNA circles. Cells were grown as described as in (A) except (1) DNA circles were excised following M arrest, and (2) transcription was not induced. Strains MSB203, MSB204, MSB205 and MSB206 were used. Protein depletions are signified by downward arrows above each column. P values for pairwise χ2 tests are presented relative to a wild-type. (C) Cohesin translocation in strains with no protein depletion. Strains MRG7270.1, MRG7269.1 and MRG7275.1 express OsTir1 but lack AID-tagged genes. (D) Cohesin translocation without Wpl1. Wpl1-3xmAID was depleted from strains MRG7327.1, MRG7329.1, MRG7324.1. (E) Translocation in the absence of Eco1 and Wpl1. Strains MRG7332.1, MRG7356.1 and MRG7353.1 with 3xmAID alleles of eco1 and wpl1 were used. (F) Impact of basal URA3 transcription on cohesin translocation in the absence of Eco1 and Wp1. Both proteins were depleted in strains that carried either the intact URA3 cassette (wt), a precise deletion of the URA3 promoter (Δpro) or a larger deletion that also included upstream sequences (Δpro+). See Fig. S8A for details. Accumulation of cohesin at 5’lacO was measured in media containing uracil. Strains MRG7332.1, MSB7715, MSB7710, MRG7353.1, MSB7716, and MSB7712 were used. Student’s t tests are presented separately for the sets of zero and four mCherry barriers. (G) Cohesin translocation without Eco1. Eco1-3xmAID was depleted in strains MRG7301.1, MRG7300.1, MRG7302.1. P values for pairwise Student’s t tests evaluate the impact of transcriptional induction.
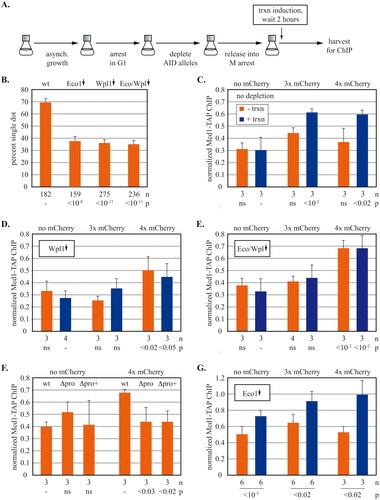
Interpretation of these experiments requires knowing whether the depletions affect cohesion of the locus of study. Therefore, pairing of URA3 DNA circles was evaluated during M arrest. Induction of the gene was omitted for simplicity. As expected, shows that depletion of Eco1 eliminated cohesion. The residual frequency of single dots was comparable to that of DNA circles lacking URA3.Citation34 Co-depletion of Eco1 and Wpl1 also yielded a severe cohesion defect, as anticipated from earlier work (See,Citation46 and references there in). Wpl1 depletion was previously shown to have an intermediate effect on cohesion.Citation46–48 Here, Wpl1 depletion abolished cohesion of the circles. Small extrachromosomal DNAs may be well suited to detect cohesion defects because they lack the buffer of additional cohesion sites in a long chromosomal arm. These data indicate that the locus of interest lacks cohesive cohesin when Wpl1 and/or Eco1 are removed.
In the absence of any protein depletion, cohesin accumulated at barriers with three or more mCherries, as described earlier (). When Wpl1 alone was depleted, two notable changes occurred (). First, the three mCherry chimera no longer functioned as a roadblock. Accumulation of cohesin at the four mCherry barrier persisted, albeit at reduced levels. The switch from a three mCherry barrier to four mCherries was more dramatic when both Eco1 and Wpl1 were depleted (). Thus, the threshold size for barrier activity increases in the absence of Wpl1 alone or in conjunction with the loss of Eco1. The data are consistent with loss of cohesion, resulting in translocation of non-cohesive complexes on single chromatids but see Discussion for further considerations.
The second notable change is that cohesin accumulation at four mCherry barrier did not require induction of URA3. This induction-independent signal was seen more clearly after depleting both Wpl1 and Eco1 (). Accumulation without URA3 induction also occurred during late-G1 arrest in a strain lacking Wpl1 (Fig. S7A). Measurement of URA3 mRNA showed that Wpl1 loss did not cause constitutive activation of the gene (Fig. S6D and Fig. S7B). Instead we hypothesized that basal URA3 transcription is sufficient to translocate cohesin in the absence of Wpl1. This was tested by deleting the core URA3 promoter (Δpro) or deleting a larger region that included additional upstream sequences (Δpro+) (Fig. S8). For this experiment both Wpl1 and Eco1 were depleted because the double depletion yielded the greatest induction-independent signal. shows that the promoter deletions eliminiated cohesin accumulation at the four mCherry barrier. By contrast, the low level accumulation at GFP-lacI was unchanged. Thus, basal transcription is sufficient to transport cohesin to barriers in the absence of Wpl1 and Eco1. Cohesin residence time on chromosomes increases in Wpl1 mutants.Citation41 This increase may amplify the impact of even low levels of transcription on cohesin translocation.
Unexpectedly, depletion of just Eco1 caused transcription-dependent cohesin accumulation at GFP-lacI without mCherries (). This was due specifically to loss of Eco1 because it did not occur in the same strain when auxin was omitted (Fig. S9A). The blockade was also dependent on the chimera: cohesin accumulation did not occur in a strain lacking GFP-lacI (Fig. S9B). It appears that Eco1, presumably through Smc3 acetylation, facilitates translocation past the smallest synthetic barrier. Interestingly, the paradoxical accumulation of cohesin at GFP-lacI is abolished when Eco1 and Wpl1 are co-depleted (). Perhaps, cohesin eventually bypasses the small barrier when residence time is extended by simultaneous loss of Wpl1.
DISCUSSION
In this study we show that synthetic roadblocks of sufficient size impede transcription-driven translocation of cohesin in vivo. Large native proteins may similarly delay passage of the complex without utilizing specific protein-protein interactions like those that govern the boundary activity of CTCF.Citation49 While our work was underway, a study using dCas9 showed that the ectopic protein can block the expansion of loops by cohesin in vivo.Citation50 Our work goes further in three significant ways (summarized in ). First, we show that transcription triggers translocation of both cohesive and non-cohesive complexes. Second, we show that the two types of complexes are blocked by barriers of different size: four mCherries are required for non-cohesive cohesin () while three mCherries are required for cohesive complexes (). Third, we show that stalled cohesive complexes block passage of non-cohesive complexes ().
FIG 7 Barriers of sufficient size block transcription-triggered, processive movement of cohesin in vivo. (A) Cohesive cohesin cannot pass a barrier larger than three mCherries during M phase. Non-cohesive complexes translocating on a single DNA (in late G1 or M phase) cannot pass a barrier larger than four mCherries. Cohesin is depicted as a topologically-bound, black ring for simplicity. (B) Non-cohesive cohesin is blocked by cohesive cohesin that cannot pass three mCherries. (C) In the absence of Eco1 alone, cohesin cannot pass GFP-lacI, the smallest barrier that is bypassed in all other conditions.
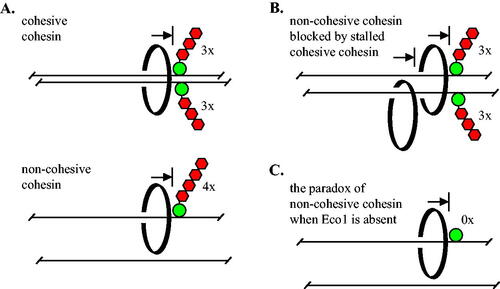
Studies of cohesin within cells demonstrated that single cohesive complexes can entrap both sister chromatids topologically.Citation3,Citation4 Complexes assembled in vitro can also bind two DNAs simultaneously, and move on DNA either by diffusion or mechanical force.Citation51,Citation52 Presumably, the cohesive cohesin that translocates in our studies is similarly bound to DNA. Crosslinking analyses have defined a central DNA chamber formed by the interactions of core subunits (Smc1, Smc3 and Mcd1), which is divided into two sub-chambers by associations of the Smc head domains.Citation53,Citation54 While it is tempting to estimate chamber size from the known dimensions of GFP, lacI, mCherry and the nucleosome, too many variables remain undetermined to do so (e.g. the impacts of flexible linkers, lacI dimerization and dense binding to the lacO array). Nevertheless, synthetic obstacles of increasing size provide a relative measure of the chamber(s) of cohesive cohesin that embrace DNA during transcription-driven translocation.
Cohesin within cells can also bind single chromatids in a topological manner.Citation3,Citation4 These complexes diffuse on DNA in vitro unless mobilized by an enzyme like RNA polymerase.Citation20,Citation22 The non-cohesive complexes in our studies may be bound in this way. If so, doubling the number of topologically bound DNAs could explain the more stringent barrier threshold size, either because they must both occupy a single chamber or because a smaller sub-chamber is used by one of the two. Additional cell-cycle dependent parameters, like Smc3 acetylation or cohesin oligomerization, could also modulate such size restrictions.Citation55
This simple DNA entrapment scenario does not account for the recent discovery of a non-topological binding mode for complexes that extrude loops. Remarkably, loop extrusion persists when DNA access to the central chamber of purified cohesin is blocked by peptide fusions and crosslinking.Citation23 Correspondingly, intracellular translocation of cohesin persists in mutants that do not support topological entrapment.Citation56 Thus, it is possible that the non-cohesive, translocating complexes in our study are extruding loops and not topologically engaged. Such a view must account for the fact that loop extruding complexes bypass roadblocks in vitro that greatly exceed the size of cohesin, a feat that is difficult to explain by a topological or even pseudo topological embrace.Citation57 By contrast, translocation of both cohesive and non-cohesive complexes here displays sharp transitions in the threshold size of much smaller synthetic barriers. Thus, while it is clear that synthetic obstacles stall non-cohesive cohesin, it is not yet clear how these transcription-driven complexes bind DNA or what aspect of translocation is impeded.
Both Eco1 and Wpl1 influence the size and distribution of chromosome loops in yeast, as do their homologs in higher eukaryotes.Citation7,Citation8,Citation29,Citation30,Citation31,Citation58,Citation59 Whereas Wpl1 restricts loop size by limiting the residence time and location of loop extruding complexes on DNA, Eco1 acts by limiting loop extruding activity via recruitment of Pds5. If transcription triggers movement of loop extruding complexes toward our synthetic barriers, then conditions that favor loop extension might favor their transit past synthetic barriers. Indeed, depletion of Wpl1 increased the threshold size of a barrier in M phase cells from three to four mCherries and co-depletion of Eco1 and Wpl1 made the effect more pronounced (). However, the locus under study was not cohesed in either of these situations (). Thus, a simpler explanation may prevail: the increase in barrier size from three to four mCherries reflects the absence of cohesive complexes.
Lastly, depletion of Eco1 alone yielded an entirely unexpected result: GFP-lacI alone was sufficient to impede cohesin passage (). This unusual impact of the acetyltransferase will require further investigation.
Our work shows that stalled cohesive cohesin blocks passage of non-cohesive complexes (). Recent findings align with this conclusion. During mouse meiosis, cohesive cohesin complexes bearing Rec8 (meiosis-specific Scc1) limit the passage of loop extruding complexes bearing Scc1.Citation60 Additionally, loop extrusion complexes in human mitotic cells do not simply bypass cohesive complexes but instead help position them.Citation12 Finally, stably bound cohesin in yeast (possibly cohesive complexes) blocks passage of complexes that extrude loops.Citation7 It will be interesting to see how widely stalled cohesive cohesin contributes to shaping the genome by non-cohesive complexes, and whether this process is used to regulate biological events.
MATERIALS AND METHODS
Yeast strains and plasmids
Strains generated for this study were confirmed by PCR and/or DNA sequencing. Strains and primers are listed in Table S1 and Table S2, respectively. GFP-lacI with a linked SV40 NLS was expressed from either a single or tandem integration of plasmid pGVH60, as noted in the strain table. These integrants were modified by PCR-mediated gene tagging using multimeric repeats of mCherry from plasmids pMAM12 and pMAM44 or three yeGFP(A206R)s from plasmid pMAM88. Strains with tandem pGVH60 thus yielded simultaneous expression a GFP-lacI chimera and GFP-lacI alone. However, strains co-expressing GFP-lacI/GFP-lacI-mCherry chimeras produced identical results to strains expressing the mCherry chimeras alone (data not shown). The original array of 256 lac operators shortened early in the strain lineage and now contains roughly 128 operators, as determined by Southern blotting. The nuclear envelope tag SS-mCherry-HDEL was expressed from integrated plasmid ZJOM090 (Addgene ID: 133647, Zhiping Xie). Integrating plasmid pXDA2-FFAT was assembled by in vivo recombination from pAFS144-FFAT and pXRA2.Citation61 pXDL2-GAL1p-Sic1(4m)-AID*-9xmyc was similarly assembled from pXRL2,Citation61 pRS306-GAL-Mt-4A-SIC1-HA-HIS6-ActCitation40 and pKan-AID*-9myc.Citation62 Integrating plasmid pXDnMX-GAL1p-Scc1(R180D,R268D)-TAP was assembled by in vivo recombination from YIplac128-GAL1p-Scc1(R180D,R268D)-3xHA (LEU2), pXDnMX,Citation61 and a PCR fragment containing a TAP tag. Other tagged genes were constructed by PCR-mediated gene replacement with the 6xHIS-10xFLAG module from strain YSI142 or the 3xmAID-5xFLAG module from pST1933 (NBRP ID: BYP8880, Seiji Tanaka). OsTir1 was from integrated pTIR5 (ADH1p-OsTIR1-CaADH1t, Thomas Eng thesis, U.C. Berkeley) or integrated pMSB27 (ADH1p-OsTIR1(yeast codon optimized)), which was derived by in vivo recombination from pXRT1Citation61 and pMK200 (NBRP ID: BYP7569, Masato Kanemaki). URA3 promoter deletions were made by Crispr gene editing using in vivo recombination to program the gRNA expression cassette within a Cas9 expression plasmid bRA90 (Addgene ID: 100951, James Haber).
Cell growth and arrest
To arrest cells in M phase for ChIP-qPCR, MET3p-cdc20 was shut off by adding methionine (Cf = 2 mM) to mid-log cultures grown in SC-met media. After 2.5 h (roughly 80% of the cells adopted a dumbbell shape), cells were washed twice with water, split in half and resuspended in either SC complete or SC media lacking uracil to induce URA3. Two hours later the cultures were fixed for ChIP, according to.Citation34 To arrest cells in M phase for cohesion assays, cultures were grown in SC-met for 6–8 h and then diluted 200–400 fold into SC-met plus raffinose for overnight growth. When cells reached mid-log the following morning, methionine was added. 150 minutes later, cells were washed twice and resuspended in SC plus raffinose media that either contained or lacked uracil. Two hours later, galactose was added (Cf = 2%) to induce DNA circularization by the R recombinase. Cells were fixed with paraformaldehyde after two additional hours. To arrest cells for ChIP in late G1, cultures were grown in SC-met for 6–8 h and then diluted 200–400 fold into SC-met plus raffinose for overnight growth. When the cultures reached mid-log the following morning, alpha factor was added (Cf = 10 µM), and 90 minutes later galactose was added to induce expression of GAL1p-SIC1(4m)-AID*-9xmyc. After one additional hour, cultures were washed twice with water and resuspended in SC-met plus galactose and pronase E (Cf = 0.1 mg/ml) to release from pheromone arrest and re-arrest in late G1. One hour later, the cells were washed twice, split and resuspended in SC-met plus galactose either containing or lacking uracil. Two hours later, the cultures were fixed for ChIP. For release from late G1 arrest, cells were grown into the arrest as described above but with the following exceptions. After cells were washed to remove alpha factor, they were split, resuspended in SC complete media plus galactose and pronase E that either contained or lacked uracil. Two hours later, dextrose (Cf = 2%) was added to stop expression of Sic1(4m)-AID*-9xmyc and 1-naphthaleneacetic acid (NAA, Cf = 100 µM) was added to degrade the existing protein. Cultures were fixed for ChIP two hours later when cells accumulated in M phase. To provide Mcd1-TAP exclusively in M phase, strains with mcd1-S525N were grown at 25oC in SC-met plus raffinose as described above until mid-log cultures were arrested in M phase by Cdc20 depletion. Two and half hours later, galactose was added to induce expression of GAL1p-mcd1(R180D,R268D)-TAP. The mutated arginines of the separase cleavage sites do not affect cells arrested in M. One hour later cultures were split and one half was shifted to 37oC. Thirty minutes later, the cultures were washed twice, split again and resuspended with the same media either containing or lacking uracil. Cells were fixed for ChIP after an additional two hours. For depletion of the cohesin regulators with 3xmAID tags, mid-log cultures in SC-met were arrested by the addition of alpha factor. 90 minutes later NAA was added. One hour later cultures were released from G1 to re-arrest in the subsequent M phase by washing twice and resuspending in SC complete media containing pronase E. 90 minutes later, cells were washed twice, split and resuspended in SC complete media containing or lacking uracil. Two hours later, cells were fixed for ChIP. For cohesion assays involving protein depletions, cells were grown similarly except that initial cultures were diluted into SC-met plus raffinose for overnight growth before G1 arrest, protein depletion and release to M phase arrest the following day. Galactose was then added and cells were fixed two hours later.
Other techniques
ChIP-qPCR, RT-qPCR and cohesion assays were performed as described in Borrie et al.Citation34 ChIP reactions used anti-TAP, anti-FLAG and protein A-coated Dynabeads (CAB1001 and 10001D, Invitrogen; F1804, Sigma). qPCR for ChIP was performed with the Qiagen Rotor-Gene SYBR green kit until it was superseded by the Qiagen QuantiNova SYBR Green kit (, Fig. S5 and ). All values were normalized to an unlinked cohesin-bound site (549.7 kb on Chr IV). For ChIP-qPCR and RT-qPCR, the mean and standard deviation of three or more biological replicates are presented. For cohesion assays, the data from three independent trials were pooled because they satisfied χ2 tests of the homogeneity of proportions. Error bars represent the standard error of proportions. Statistical significance was determined by either pairwise Student’s t tests (ChIP-qPCR and RT-qPCR) or χ2 tests (cohesion). Immunoblots were probed with anti-GFP (G1544, Sigma), anti-myc (9E10, Roche), anti-mini-AID (M214-3, MBL), anti-Pgk1 (ab113687, Abcam) or anti-Rad23 (from the Madura laboratory), and secondary antibodies (W401B and W402B, Promega).
Supplemental Material
Download Zip (16.7 MB)ACKNOWLEDGEMENTS
We thank the following individuals for reagents: Jason Brickner (pAFS144-FFAT), Michael Knop (pMAM12, pMAM44 and pMAM88), Takehiko Kobayashi (strain YSI142 with MCD1-6xHIS-10xFLAG::kanMX), Kiran Madura (anti-Rad23), Adam Rudner (pTIR5), and Frank Uhlmann (YIplac128-GAL1p-Scc1(R180D,R268D)-3xHA (LEU2) and strain K5833 with scc1-73).
SUPPLEMENTAL MATERIAL
Supplemental data for this article can be accessed online at https://doi.org/10.1080/10985549.2023.2199660.
DATA AVAILABILITY STATEMENT
A book of Excel spreadsheets with the primary data for the figures in this study is available at Figshare https://doi.org/10.6084/m9.figshare.22551586.
Additional information
Funding
REFERENCES
- Davidson IF, Peters J-M. Genome folding through loop extrusion by SMC complexes. Nat Rev Mol Cell Biol. 2021;22:445–464. doi:10.1038/s41580-021-00349-7.
- Remeseiro S, Cuadrado A, Losada A. Cohesin in development and disease. Development. 2013;140:3715–3718. doi:10.1242/dev.090605.
- Gligoris TG, Scheinost JC, Burmann F, Petela N, Chan KL, Uluocak P, Beckouët F, Gruber S, Nasmyth K, Löwe J. Closing the cohesin ring: structure and function of its Smc3-kleisin interface. Science. 2014;346:963–967. doi:10.1126/science.1256917.
- Haering CH, Farcas AM, Arumugam P, Metson J, Nasmyth K. The cohesin ring concatenates sister DNA molecules. Nature. 2008;454:297–301. doi:10.1038/nature07098.
- Glynn EF, Megee PC, Yu HG, Mistrot C, Ünal E, Koshland DE, DeRisi JL, Gerton JL. Genome-wide mapping of the cohesin complex in the yeast Saccharomyces cerevisiae. PLoS Biol. 2004;2:e259. doi:10.1371/journal.pbio.0020259.
- Lengronne A, Katou Y, Mori S, Yokobayashi S, Kelly GP, Itoh T, Watanabe Y, Shirahige K, Uhlmann F. Cohesin relocation from sites of chromosomal loading to places of convergent transcription. Nature. 2004;430:573–578. doi:10.1038/nature02742.
- Costantino L, Hsieh TS, Lamothe R, Darzacq X, Koshland D. Cohesin residency determines chromatin loop patterns. eLife. 2020;9:e59889. doi:10.7554/eLife.59889.
- Dauban L, Montagne R, Thierry A, Lazar-Stefanita L, Bastie N, Gadal O, Cournac A, Koszul R, Beckouet F. Regulation of cohesin-mediated chromosome folding by Eco1 and other partners. Mol Cell. 2020;77:1279–1293.e4. doi:10.1016/j.molcel.2020.01.019.
- Oomen ME, Hedger AK, Watts JK, Dekker J. Detecting chromatin interactions between and along sister chromatids with SisterC. Nat Methods. 2020;17:1002–1009. doi:10.1038/s41592-020-0930-9.
- Schalbetter SA, Fudenberg G, Baxter J, Pollard KS, Neale MJ. Principles of meiotic chromosome assembly revealed in S. cerevisiae. Nat Commun. 2019;10:4795. doi:10.1038/s41467-019-12629-0.
- Hadjur S, Williams LM, Ryan NK, Cobb BS, Sexton T, Fraser P, Fisher AG, Merkenschlager M. Cohesins form chromosomal cis-interactions at the developmentally regulated IFNG locus. Nature. 2009;460:410–413. doi:10.1038/nature08079.
- Mitter M, Gasser C, Takacs Z, Langer CCH, Tang W, Jessberger G, Beales CT, Neuner E, Ameres SL, Peters J-M, et al. Conformation of sister chromatids in the replicated human genome. Nature. 2020;586:139–144. doi:10.1038/s41586-020-2744-4.
- Rao SSP, Huang SC, Glenn St Hilaire B, Engreitz JM, Perez EM, Kieffer-Kwon KR, Sanborn AL, Johnstone SE, Bascom GD, Bochkov ID, et al. Cohesin loss eliminates all loop domains. Cell. 2017;171:305–320.e24. doi:10.1016/j.cell.2017.09.026.
- Uhlmann F. SMC complexes: from DNA to chromosomes. Nat Rev Mol Cell Biol. 2016;17:399–412. doi:10.1038/nrm.2016.30.
- Lengronne A, McIntyre J, Katou Y, Kanoh Y, Hopfner K-P, Shirahige K, Uhlmann F. Establishment of sister chromatid cohesion at the S. cerevisiae replication fork. Mol Cell. 2006;23:787–799. doi:10.1016/j.molcel.2006.08.018.
- D'Ambrosio C, Schmidt CK, Katou Y, Kelly G, Itoh T, Shirahige K, Uhlmann F. Identification of cis-acting sites for condensin loading onto budding yeast chromosomes. Genes Dev. 2008;22:2215–2227. doi:10.1101/gad.1675708.
- Busslinger GA, Stocsits RR, van der Lelij P, Axelsson E, Tedeschi A, Galjart N, Peters JM. Cohesin is positioned in mammalian genomes by transcription, CTCF and Wapl. Nature. 2017;544:503–507. doi:10.1038/nature22063.
- Ocampo-Hafalla M, Muñoz S, Samora CP, Uhlmann F. Evidence for cohesin sliding along budding yeast chromosomes. Open Biol. 2016;6:e150178. doi:10.1098/rsob.150178.
- Bausch C, Noone S, Henry JM, Gaudenz K, Sanderson B, Seidel C, Gerton JL. Transcription alters chromosomal locations of cohesin in Saccharomyces cerevisiae. Mol Cell Biol. 2007;27:8522–8532. doi:10.1128/MCB.01007-07.
- Davidson IF, Goetz D, Zaczek MP, Molodtsov MI, Huis In 't Veld PJ, Weissmann F, Litos G, Cisneros DA, Ocampo-Hafalla M, Ladurner R, et al. Rapid movement and transcriptional re-localization of human cohesin on DNA. Embo J. 2016;35:2671–2685. doi:10.15252/embj.201695402.
- Kanke M, Tahara E, Huis In’t Veld PJ, Nishiyama T. Cohesin acetylation and Wapl-Pds5 oppositely regulate translocation of cohesin along DNA. Embo J. 2016;35:2686–2698. doi:10.15252/embj.201695756.
- Stigler J, Çamdere GO, Koshland DE, Greene EC. Single-molecule imaging reveals a collapsed conformational state for DNA-bound cohesin. Cell Rep. 2016;15:988–998. doi:10.1016/j.celrep.2016.04.003.
- Davidson IF, Bauer B, Goetz D, Tang W, Wutz G, Peters JM. DNA loop extrusion by human cohesin. Science. 2019;366:1338–1345. doi:10.1126/science.aaz3418.
- Kim Y, Shi Z, Zhang H, Finkelstein IJ, Yu H. Human cohesin compacts DNA by loop extrusion. Science. 2019;366:1345–1349. doi:10.1126/science.aaz4475.
- Fudenberg G, Imakaev M, Lu C, Goloborodko A, Abdennur N, Mirny LA. Formation of chromosomal domains by loop extrusion. Cell Rep. 2016;15:2038–2049. doi:10.1016/j.celrep.2016.04.085.
- Nasmyth K. Disseminating the genome: joining, resolving, and separating sister chromatids during mitosis and meiosis. Annu Rev Genet. 2001;35:673–745. doi:10.1146/annurev.genet.35.102401.091334.
- Sanborn AL, Rao SS, Huang SC, Durand NC, Huntley MH, Jewett AI, Bochkov ID, Chinnappan D, Cutkosky A, Li J, et al. Chromatin extrusion explains key features of loop and domain formation in wild-type and engineered genomes. Proc Natl Acad Sci U S A. 2015;112:e6456.
- Gassler J, Brandao HB, Imakaev M, Flyamer IM, Ladstatter S, Bickmore WA, Peters J-M, Mirny LA, Tachibana K. A mechanism of cohesin-dependent loop extrusion organizes zygotic genome architecture. Embo J. 2017;36:3600–3618. doi:10.15252/embj.201798083.
- Haarhuis JHI, van der Weide RH, Blomen VA, Yanez-Cuna JO, Amendola M, van Ruiten MS, Krijger PHL, Teunissen H, Medema RH, van Steensel B, et al. The cohesin release factor WAPL restricts chromatin loop extension. Cell. 2017;169:693–707.e14. doi:10.1016/j.cell.2017.04.013.
- Wutz G, Varnai C, Nagasaka K, Cisneros DA, Stocsits RR, Tang W, Schoenfelder S, Jessberger G, Muhar M, Hossain MJ, et al. Topologically associating domains and chromatin loops depend on cohesin and are regulated by CTCF, WAPL, and PDS5 proteins. Embo J. 2017;36:3573–3599. doi:10.15252/embj.201798004.
- Bastié N, Chapard C, Dauban L, Gadal O, Beckouët F, Koszul R. Smc3 acetylation, Pds5 and Scc2 control the translocase activity that establishes cohesin-dependent chromatin loops. Nat Struct Mol Biol. 2022;29:575–585. 10.1038/s41594-022-00780-0.
- Dequeker BJH, Scherr MJ, Brandao HB, Gassler J, Powell S, Gaspar I, Flyamer IM, Lalic A, Tang W, Stocsits R, et al. MCM complexes are barriers that restrict cohesin-mediated loop extrusion. Nature. 2022;606:197–203. doi:10.1038/s41586-022-04730-0.
- Banigan EJ, Tang W, Van Den Berg AA, Stocsits RR, Wutz G, Brandão HB, Busslinger GA, Peters J-M, Mirny LA. Transcription shapes 3D chromatin organization by interacting with loop extrusion. Proc Natl Acad Sci U S A. 2023;120:e2210480120. doi:10.1073/pnas.2210480120.
- Borrie MS, Campor JS, Joshi H, Gartenberg MR. Binding, sliding, and function of cohesin during transcriptional activation. Proc Natl Acad Sci U S A. 2017;114:e1062.
- Gartenberg MR, Neumann FN, Laroche T, Blaszczyk M, Gasser SM. Sir-mediated repression can occur independently of chromosomal and subnuclear contexts. Cell. 2004;119:955–967. doi:10.1016/j.cell.2004.11.008.
- Brickner JH, Walter P. Gene recruitment of the activated INO1 locus to the nuclear membrane. PLoS Biol. 2004;2:e342. eng. doi:10.1371/journal.pbio.0020342.
- Karaboja X, Ren Z, Brandao HB, Paul P, Rudner DZ, Wang X. XerD unloads bacterial SMC complexes at the replication terminus. Mol Cell. 2021;81:756–766.e8. doi:10.1016/j.molcel.2020.12.027.
- Huang L, Pike D, Sleat DE, Nanda V, Lobel P. Potential pitfalls and solutions for use of fluorescent fusion proteins to study the lysosome. PLoS One. 2014;9:e88893. doi:10.1371/journal.pone.0088893.
- Shaner NC, Campbell RE, Steinbach PA, Giepmans BN, Palmer AE, Tsien RY. Improved monomeric red, orange and yellow fluorescent proteins derived from Discosoma sp. red fluorescent protein. Nat Biotechnol. 2004;22:1567–1572. doi:10.1038/nbt1037.
- Verma R, Annan RS, Huddleston MJ, Carr SA, Reynard G, Deshaies RJ. Phosphorylation of Sic1p by G1 Cdk required for its degradation and entry into S phase. Science. 1997;278:455–460. doi:10.1126/science.278.5337.455.
- Chan KL, Roig MB, Hu B, Beckouët F, Metson J, Nasmyth K. Cohesin’s DNA exit gate is distinct from its entrance gate and is regulated by acetylation. Cell. 2012;150:961–974. doi:10.1016/j.cell.2012.07.028.
- Haering CH, Schoffnegger D, Nishino T, Helmhart W, Nasmyth K, Löwe J. Structure and stability of cohesin’s Smc1-kleisin interaction. Mol Cell. 2004;15:951–964. doi:10.1016/j.molcel.2004.08.030.
- Ström L, Lindroos HB, Shirahige K, Sjögren C. Postreplicative recruitment of cohesin to double-strand breaks is required for DNA repair. Mol Cell. 2004;16:1003–1015. doi:10.1016/j.molcel.2004.11.026.
- Uhlmann F, Nasmyth K. Cohesion between sister chromatids must be established during DNA replication. Curr Biol. 1998;8:1095–1101. doi:10.1016/s0960-9822(98)70463-4.
- Michaelis C, Ciosk R, Nasmyth K. Cohesins: chromosomal proteins that prevent premature separation of sister chromatids. Cell. 1997;91:35–45. English. doi:10.1016/s0092-8674(01)80007-6.
- Guacci V, Stricklin J, Bloom MS, Guō X, Bhatter M, Koshland D. A novel mechanism for the establishment of sister chromatid cohesion by the ECO1 acetyltransferase. Mol Biol Cell. 2015;26:117–133. doi:10.1091/mbc.E14-08-1268.
- Rowland BD, Roig MB, Nishino T, Kurze A, Uluocak P, Mishra A, Beckouët F, Underwood P, Metson J, Imre R, et al. Building sister chromatid cohesion: Smc3 acetylation counteracts an antiestablishment activity. Mol Cell. 2009;33:763–774. eng. doi:10.1016/j.molcel.2009.02.028.
- Sutani T, Kawaguchi T, Kanno R, Itoh T, Shirahige K. Budding yeast Wpl1(Rad61)-Pds5 complex counteracts sister chromatid cohesion-establishing reaction. Curr Biol. 2009;19:492–497. doi:10.1016/j.cub.2009.01.062.
- Li Y, Haarhuis JHI, Sedeno Cacciatore A, Oldenkamp R, van Ruiten MS, Willems L, Teunissen H, Muir KW, de Wit E, Rowland BD, et al. The structural basis for cohesin-CTCF-anchored loops. Nature. 2020;578:472–476. doi:10.1038/s41586-019-1910-z.
- Zhang Y, Zhang X, Ba Z, Liang Z, Dring EW, Hu H, Lou J, Kyritsis N, Zurita J, Shamim MS, et al. The fundamental role of chromatin loop extrusion in physiological V(D)J recombination. Nature. 2019;573:600–604. doi:10.1038/s41586-019-1547-y.
- Gutierrez-Escribano P, Newton MD, Llauró A, Huber J, Tanasie L, Davy J, Aly I, Aramayo R, Montoya A, Kramer H, et al. A conserved ATP- and Scc2/4-dependent activity for cohesin in tethering DNA molecules. Sci Adv. 2019;5:eaay6804. doi:10.1126/sciadv.aay6804.
- Murayama Y, Samora CP, Kurokawa Y, Iwasaki H, Uhlmann F. Establishment of DNA-DNA interactions by the cohesin ring. Cell. 2018;172:465–477.e15. doi:10.1016/j.cell.2017.12.021.
- Chapard C, Jones R, van Oepen T, Scheinost JC, Nasmyth K. Sister DNA entrapment between juxtaposed Smc heads and kleisin of the cohesin complex. Mol Cell. 2019;75:224–237.e5. doi:10.1016/j.molcel.2019.05.023.
- Collier JE, Lee BG, Roig MB, Yatskevich S, Petela NJ, Metson J, Voulgaris M, Gonzalez Llamazares A, Lowe J, Nasmyth KA. Transport of DNA within cohesin involves clamping on top of engaged heads by Scc2 and entrapment within the ring by Scc3. eLife. 2020;9:e59560. doi:10.7554/eLife.59560.
- Xiang S, Koshland D. Cohesin architecture and clustering in vivo. Elife. 2021;10:e62243. doi:10.7554/eLife.62243.
- Srinivasan M, Scheinost JC, Petela NJ, Gligoris TG, Wissler M, Ogushi S, Collier JE, Voulgaris M, Kurze A, Chan KL, et al. The cohesin ring uses its hinge to organize DNA using non-topological as well as topological mechanisms. Cell. 2018;173:1508–1519.e18. doi:10.1016/j.cell.2018.04.015.
- Pradhan B, Barth R, Kim E, Davidson IF, Bauer B, van Laar T, Yang W, Ryu JK, van der Torre J, Peters JM, et al. SMC complexes can traverse physical roadblocks bigger than their ring size. Cell Rep. 2022;41:111491. doi:10.1016/j.celrep.2022.111491.
- Barton RE, Massari LF, Robertson D, Marston AL. Eco1-dependent cohesin acetylation anchors chromatin loops and cohesion to define functional meiotic chromosome domains. eLife. 2022;11:e74447. doi:10.7554/eLife.74447.
- Wutz G, Ladurner R, St Hilaire BG, Stocsits RR, Nagasaka K, Pignard B, Sanborn A, Tang W, Varnai C, Ivanov MP, et al. ESCO1 and CTCF enable formation of long chromatin loops by protecting cohesin(STAG1) from WAPL. eLife. 2020;9:e52091. doi:10.7554/eLife.52091.
- Chatzidaki EE, Powell S, Dequeker BJH, Gassler J, Silva MCC, Tachibana K. Ovulation suppression protects against chromosomal abnormalities in mouse eggs at advanced maternal age. Curr Biol. 2021;31:4038–4051.e7. doi:10.1016/j.cub.2021.06.076.
- Chou CC, Patel MT, Gartenberg MR. A series of conditional shuttle vectors for targeted genomic integration in budding yeast. FEMS Yeast Res. 2015;15:fov010.
- Morawska M, Ulrich HD. An expanded tool kit for the auxin-inducible degron system in budding yeast. Yeast. 2013;30:341–351. doi:10.1002/yea.2967.
- Bloom MS, Koshland D, Guacci V. Cohesin function in cohesion, condensation, and DNA repair is regulated by Wpl1p via a common mechanism in Saccharomyces cerevisiae. Genetics. 2018;208:111–124. doi:10.1534/genetics.117.300537.