Abstract
The non-mulberry silkworm, Antheraea mylitta Drury, 1773, is important for the silk industry. Its larvae are phytophagous and pass through five stages (1st–5th instar) during larval development. Cellular events during their development contribute to increased levels of pro-oxidants. Antioxidant defences are, therefore, of critical importance in minimising oxidative damage. Thus, in the present study, stage-specific oxidative challenges and relative levels of antioxidant defences have been assessed during the larval development of A. mylitta. The overall results indicate a progressive decrease in oxidative threat during larval ontogeny. Comparatively high activity of superoxide dismutase (SOD) and catalase (CAT) observed in the 1st instar larvae indicates an adaptive antioxidant response, which could attenuate the elevated oxidative challenges. CAT activity remained unaltered in the midgut during transformation of the larvae from 4th–5th instar, and it was below detection level in serum. Glutathione S-transferase (GST) activity did not exhibit a specific trend; however, it showed tissue specificity in advanced larvae. Glutathione (GSH) content was progressively enhanced during development and exhibited a compensatory function with ascorbic acid (ASA), thus substantiating the role of a GSH–ASA redox couple. Findings of the study imply that early larvae (1st–3rd instar) encounter considerable degrees of pro-oxidative assault and get protection from enzymatic antioxidants. In contrast, advanced larvae receive combined protection from enzymatic and nonenzymatic antioxidants. Therefore, it is assumed that oxidative stress during larval development of A. mylitta is stage-specific and, accordingly, the antioxidant defences are strategic in providing protection to the developing larvae.
Introduction
Reactive oxygen species (ROS) are generated through incomplete reduction of molecular oxygen (O2) during aerobic metabolism. They remain in a more reactive state than molecular oxygen and can potentially damage cellular macromolecules like proteins, DNA, lipids, etc., when physiological limits are crossed (Fridovich Citation1978). A primary ROS is a superoxide radical (O2.–) which is formed by univalent reduction of O2. Further reduction of O2.– produces hydrogen peroxide (H2O2) and a hydroxyl radical (.∙OH; Chance et al. Citation1979; Cross et al. Citation1987; Halliwell & Gutteridge Citation1992). ROS production varies in proportion to cellular activities, and, particularly during development, events like cellular proliferation, differentiation and migration for pattern formation constantly require a higher metabolic gradient, and hence a higher level of ROS (Garcia et al. Citation2010). Progressive accumulation of ROS during development predisposes the organism towards pathogenesis and declining physiological functions. All organisms possess a battery of antioxidants, which can trap ROS before they oxidise biomolecules or reduce those that have been oxidised (Steenvoorden & Henegouwen Citation1997; Blokhina et al. Citation2003; Pinnell Citation2003). Superoxide dismutase (SOD), catalase (CAT) and glutathione peroxidase (GPx) are front-line antioxidant enzymes, which limit the abundance of ROS in the cellular environment (Biewenga et al. Citation1997). Glutathione S-transferase (GST) is an important detoxifying enzyme in insects, and it has been linked to the neutralisation of a wide range of hydroperoxides and acts as a substitute for the function of selenium (Se)-dependent vertebrate GPx (Lukasik & Golawska Citation2007). This enzyme uses reduced thiol (GSH) as its substrate and maintains the thiol status of the cell. Apart from antioxidant enzymes, non-enzymatic antioxidants, especially GSH and ascorbic acid, play important roles in the development of both vertebrates and invertebrates (Brinster Citation1968; Chippendale Citation1975), and are of paramount importance to the antioxidant defence of mammals and insects (Meister Citation1994; Barbehenn Citation2003). Herbivorous insects are more susceptible to oxidative injury due to their dietary habits in addition to the usual burden of ROS imposed by the aerobic lifestyle (Gulevsky et al. Citation2006). Although a few reports are available on antioxidant defences in insects in relation to their larval development, the majority of the studies are focused on the involvement of GST (Hazelton & Lang Citation1983; Wood et al. Citation1986; Kotze & Rose Citation1987; Kostarpoulos & Papadopoulos Citation1998; Kostarpoulos et al. Citation2001). Relatively little is known about other components of the antioxidant defence system and the extent of oxidative injury caused during development. Besides, there is a paucity of information on oxidative stress and the relative degree of antioxidant protection in insect tissues during their feeding on host plants (Aslanturk et al. Citation2011).
The non-mulberry silkworm, Antheraea mylitta Drury, 1773 (Lepidoptera: Saturniidae), is an economically important insect which produces an exotic variety of silk called tasar silk (Nagaraju & Goldsmith Citation2002). Terminalia arjuna is an important food plant for this silkworm species (Abraham et al. Citation2004). In its entire ontogeny, larval stages constitute the only feeding phase, which store optimum food energy to sustain the non-feeding pupal and adult stages. It is a tetra moulter, passing through five instars (1st–5th instars), and different larval stages exhibit different feeding intensities. Rath et al. (Citation2006) and Rath (Citation2010), observed that Antheraea mylitta ingests food in an increasing order during the larval progression, and the percentage of food intake in different instars is: 1st instar: 0.21%, 2nd instar: 0.95%, 3rd instar: 3.1%, 4th instar: 14.55%, 5th instar: 81.17%. Balanced nutrients in host plants play an important role in the growth and development of insects (Bhatia & Yousuf Citation2014). Although ROS and antioxidants have been reported to influence larval development, fecundity, fertility and life span (Allen Citation1991; Orr & Sohal Citation1994), information on ROS metabolism during larval development of this species is scanty, except for a recent study by Jena et al. (Citation2013). Nevertheless, only a few parameters of the redox system in the whole-body tissue of the developing larvae of A. mylitta, reared on a different host plant i.e. Terminalia tomentosa, were studied by Jena and colleagues. Phytochemical constituents of the host plant leaves contributing to the exogenous pool of pro- and antioxidants were also not included in their study. Consumption of foliar constituents by the larvae, which differs in quantity with respect to the larval stages, will alter the pro-oxidant and anti-oxidant balance of the larval tissues. Pro-oxidant and antioxidant balance has been reported to affect larval development and aging in Drosophila (Klichko et al. Citation2004). We have therefore focused our study on stage-specific oxidative challenges and relative levels of antioxidant protection in the whole-body (WB) tissue of early larvae (1st, 2nd and 3rd instars), and two important tissues of advanced larvae: hemolymph (supernatant fraction), hereafter called serum, and midgut (MG) of this insect. Both tissues are metabolically active, and MG, in particular, is directly exposed to ROS-promoting plant allelochemicals (Krishnan & Kodrik Citation2006). ROS beyond physiological levels impair the absorption of nutrients and may promote oxidative damage to MG epithelium (Bi & Felton Citation1995). Suboptimal utilisation of nutrients adversely affects larval growth, survival, reproductive fitness and, above all, silk production (Rath Citation2010). Therefore, assessment of stage-specific oxidative challenges and relative levels of antioxidant defences during the larval development of non-mulberry silk moth are a matter of crucial importance and concern. The outcome of this study will deepen our understanding of the stage-specific ROS metabolism of lepidopteron larvae with reference to their development and feeding in general and will provide baseline information on the antioxidant defence mechanism of the Saturniidae silkworm species, in particular.
Materials and methods
Chemicals
Thiobarbituric acid (TBA), bovine serum albumin (BSA) and sephadex G-25, 5,5ʹ dithiobis 2-nitrobenzoic acid (DTNB) were purchased from Sigma Chemical Co., USA. Reduced glutathione, ascorbic acid, horseradish peroxidase, hydrogen peroxide, sodium dodecyl sulphate (SDS) and 1- Chloro- 2,4- dinitrobenzene (CDNB) were obtained from SISCO Research Laboratory, India. Digitonin was procured from Hi-media Ltd., India. All other chemicals used were of analytical grade.
Insects
Larvae of trivoltine strains of tasar silk worm (A. mylitta) were collected from identical host plants (Terminalia arjuna) of the same age group maintained in the state government sericulture field located at Baripada (21°56'6''N, 86°43'17''E), Orissa, India, 2 days after the respective moult. Larvae were brought to the laboratory and starved overnight before sacrifice.
Tissue preparation
Apparently healthy larvae were chilled at −20°C for 10 min. To prepare WB homogenate, chilled larvae were weighed, pooled (for 1st instar, 10 larvae were pooled; for each of the 2nd and 3rd instars, five larvae were pooled) and 10% weight/volume (w/v) homogenate was prepared in ice-cold 50 mM phosphate buffer, pH 7.4, containing 0.1 mM Ethylenediaminetetraacetic acid (EDTA) and a pinch of Phenylmethylsulfonyl fluoride (PMSF), using a glass teflon mechanical homogeniser. From 4th and 5th instar larvae, hemolymph was collected (pooled from three larvae) by puncturing the anterior prolegs in an ice-chilled Eppendorf tube coated with 0.03% phenylthiourea (PTU) to prevent melanisation. Then the larvae were sacrificed, midguts were collected (pooled from three larvae) and gut contents were removed, washed in physiological saline (0.67% w/v), blotted dry, and weighed in a microcentrifuge tube. A 10% (w/v) homogenate was prepared in the abovementioned homogenising buffer under ice. WB and MG homogenates were centrifuged at 10,000 × g for 20 min at 4°C, and the post-mitochondrial supernatant was collected. The hemolymph (HL) samples were centrifuged at 3000 × g for 10 min at 4°C to get rid of hemocytes, and the supernatant fraction, hereafter called serum, was taken for further analysis. Endogenous H2O2 content and CAT activity were measured in the samples immediately after centrifugation. The remaining supernatant was kept at −40°C for further analysis. All measurements were made in triplicate.
Oxidative stress indices
Measurement of lipid peroxidation (LPx)
Level of LPx was assayed by monitoring the formation of malondialdehyde (MDA) according to the method adopted by Ohkawa et al. (Citation1979). In brief, a 10% (w/v) homogenate of the MG and WB was prepared in 1.15% potassium chloride (KCl) and centrifuged at 1000 × g for 10 min at 4°C to remove cell debris. A suitably diluted HL fraction (serum) and 1000 × g supernatant of MG and WB were used for the estimation of MDA. Absorbance was read at 532 nm against a suitable blank in a microprocessor-based UV-VIS spectrophotometer. All samples were treated with 0.02% butylated hydroxytoluene to prevent endogenous oxidation. The amount of MDA formed was calculated from the extinction coefficient of 1.56 × 105 M/cm (Wills Citation1969) and was expressed as nmol MDA/ mg protein.
Measurement of hydrogen peroxide (H2O2)
The hydrogen peroxide content in serum and the post- mitochondrial fraction of MG and WB were determined spectrophotometrically using horseradish peroxidase following the method developed by Pick and Keisari (Citation1981). In this assay, pure H2O2 was taken as standard and it was linear in the range of 20–80 nmol. In each tube, 1.7 mL of phosphate buffer (50 mM pH 7.4), 0.1 mL of phenol red solution and 50 µL of horseradish peroxidase (50 units) were taken and incubated for 5 min at room temperature. Then, 0.1 mL of sample was added to it. This was followed by the immediate addition of 50 µL of sodium hydroxide (NaOH; 1 N) to stop the reaction. Absorbance was read at 610 nm against a suitable blank in a UV-VIS spectrophotometer. H2O2 content was expressed as nmoles H2O2/mg protein.
Activities of antioxidant enzymes
Assay of superoxide dismutase (SOD) activity
For the measurement of the total SOD activity, 0.4 mL of post-mitochondrial supernatant containing approximately 10–15 mg of protein was passed through a 2-mL column of sephadex G-25, and the elute was used for the activity assay following the method developed by Das et al. (Citation2000) as described earlier (Dandapat et al. Citation2003). In brief, superoxide radicals generated by photoreduction of riboflavin were allowed to react with hydroxylamine hydrochloride to produce nitrite. The nitrite in turn reacted with sulphanilic acid to produce a diazonium compound, which subsequently reacted with naphthaloamine to produce a red azo compound having absorption maxima at 543 nm. SOD scavenges superoxide radicals; therefore, nitrite formation in the reaction was inversely proportional to the amount of SOD present in the sample. One unit of enzyme activity is defined as the amount of SOD capable of inhibiting 50% of nitrite formation under assay conditions. SOD activity was calculated by subtracting the value obtained for boiled samples from the corresponding unboiled samples. SOD activity was expressed as units/mg protein.
Assay of catalase (CAT) activity
The post-mitochondrial supernatant was used directly for assay of catalase activity following the decrease in absorbency of H2O2 at 240 nm (Aebi Citation1974) for a period of 2 min at 15-second intervals. Calculation was done based on the decrease in absorbency of H2O2 at the end of 2 min by taking the extinction coefficient of H2O2 as 43.6 M/cm. Activity was expressed as pkat/mg protein (pkat = 10−12 katal). One katal is defined as the amount of enzyme that transforms one mole of substrate per second.
Assay of glutathione S-transferase (GST) activity
The column-passed samples were used for the assay of glutathione S-transferase activity following the method developed by Habig and Jakoby (Citation1981). In brief, 2.6 mL of 50 mM potassium phosphate buffer pH 6.5, 0.2 mL of 90 mM GSH and 0.1 mL of sample containing approximately 50 μg of protein were mixed together to make the reaction mixture and the reaction was initiated by adding 0.1 mL of 30 mM CDNB to it. Absorbance was recorded at 340 nm at 1-min intervals for 6 min. GST activity was calculated by subtracting the value obtained from the sample blank (no enzyme control) from the corresponding samples. Specific activity of the enzyme was calculated based on the end-point analysis by taking the extinction coefficient of CDNB, i.e. 9.61 mM/cm, and was expressed as nmol CDNB conjugate formed/min/mg protein.
Estimation of protein content
The protein content of the samples for assay of oxidative stress indices and antioxidant enzymes was estimated adopting the method of Lowry et al. (Citation1951) using bovine serum albumin as standard.
Non-enzymatic antioxidants
Estimation of glutathione (GSH) content
GSH was analysed using DTNB employing the method developed by Ellman (Citation1959). The supernatants from the midgut, and WB homogenates and the serum fractions isolated earlier, were incubated with 5% (w/v) sulfosalicylic acid in ice for 5 min and centrifuged at 1000 × g for 10 min. The deproteinised supernatant obtained from the above process was mixed with 9.0 mM DTNB and incubated for 30 min at room temperature. Absorbance at 412 nm was recorded against a blank containing only DTNB. The GSH content was expressed in µmol/g tissue wet weight (wt) (for WB and MG) and µmol/mL (for serum).
Estimation of ascorbic acid (ASA) content
Serum, midgut and whole-body tissue homogenates were precipitated in 5% (w/v) Trichloro acetic acid (TCA) in ice and centrifuged at 1000 × g for 10 min. The deproteinised supernatant was taken to estimate ASA using Folin phenol reagent employing the method adopted by Jagota and Dani (Citation1982). In this method, 0.5 mL of supernatant was taken and diluted to 2.0 mL using distilled water, followed by the addition of 0.2 mL 0.2 N folin phenol reagent. The tubes were vortexed and incubated for 10 min at room temperature. The absorbance was measured at 760 nm. Ascorbic acid was taken as the standard and it was linear in the range of 20–100 μg. ASA content was expressed as μg/g tissue wet wt (for WB and MG) and μg/mL (for serum).
Phytochemical constituents of the host plant, Terminalia arjuna
For the analysis of phytochemical constituents, leaves were collected, thoroughly washed with tap water, weighed and dried in an oven overnight at 50°C. The dried leaves were homogenised (5%) in ice-cold extraction buffer (0.1 M Tris-hydrochloride (Tris-HCl) pH 8.0, 1 mM EDTA, 5 mM sodium thiosulfate (Na2SO3) and 20 mM β-mercaptoethanol). Homogenates were centrifuged at 10,000 × g for 10 min at 4°C, and total phenolics, GSH and ASA content were determined in the supernatant. H2O2 content was measured in the fresh leaf homogenates (10%) as described below.
Measurement of total phenolics
Total phenolics content of the leaves was measured according to the method of Slinkard and Singleton (Citation1977). To 1 mL of sample were added 1 mL of 95% ethanol, 5 mL of distilled water and 0.5 mL of 50% Folin–Ciocalteu’s phenol reagent. The tubes were kept at room temperature for 5 min. To this, 1 mL of 5% sodium carbonate (Na2CO3) was added, and this mixture was kept at room temperature in the dark for 1 hour. Gallic acid was used as the working standard. The total phenolics content of the sample was expressed as mg/g leaf dry wt.
Estimation of protein content, H2O2, GSH and ASA
The estimation of protein, H2O2, GSH and ASA in the leaf samples was carried out following the methods adopted by Lowry et al. (Citation1951), Pick and Keisari (Citation1981), Ellman (Citation1959) and Jagota and Dani (Citation1982), respectively, as described above.
Statistical analysis
The results are presented as means ± standard error of means (SEM) for n = 6 samples. Differences among the means were analysed by one-way analysis of variance (ANOVA), followed by Duncan’s new multiple range test. Regression analyses were also conducted to compare the changes taking places in various parameters during larval development. Differences were considered statistically significant when p < 0.05.
Results
Changes in oxidative stress indices
Level of lipid peroxidation (, )
Among the early larval stages (1st, 2nd and 3rd instars), the highest level of LPx (MDA content) was recorded in the whole-body homogenate of 1st instar larvae (). It was further observed that the LPx level declined progressively in the WB homogenate of the larvae up to the 3rd instar. In the advanced larvae (4th and 5th instars), its level was tissue-specific, having a higher value in the serum than in the MG. Nevertheless, in both tissues the LPx level was found to be diminished during larval transformation from 4th to 5th instar ().
Figure 1. Changes in (a), lipid peroxidation (nmol MDA/mg protein) in whole-body homogenate of early larval stages and (b), lipid peroxidation level in serum and midgut of 4th and 5th instar larvae of Antheraea mylitta. Data are expressed as mean ± SEM (n = 6). Means having superscripts of different letters [lower case (a–c): early larva and midgut; upper case (A–B): serum] represent a significant difference from each other within identical tissue (p < 0.05).
![Figure 1. Changes in (a), lipid peroxidation (nmol MDA/mg protein) in whole-body homogenate of early larval stages and (b), lipid peroxidation level in serum and midgut of 4th and 5th instar larvae of Antheraea mylitta. Data are expressed as mean ± SEM (n = 6). Means having superscripts of different letters [lower case (a–c): early larva and midgut; upper case (A–B): serum] represent a significant difference from each other within identical tissue (p < 0.05).](/cms/asset/a5079c68-e6a0-4b82-a360-fc6829d6c2b2/tizo_a_1103319_f0001_b.gif)
Hydrogen peroxide content (, )
Changes in H2O2 content in the WB homogenate of early larvae followed a trend parallel to that of MDA, i.e. the highest H2O2 content was observed in the 1st instar larvae and gradually decreased in the subsequent stages (). Even in advanced larvae, a similar pattern of tissue specificity was observed in the level of H2O2 in both serum and MG during larval transformation from 4th to 5th instar ().
Figure 2. Changes in hydrogen peroxide content (nmol/mg protein) in (a), whole-body homogenate of early larval stages and (b), serum and midgut of 4th and 5th instar of Antheraea mylitta. Data are expressed as mean ± SEM (n = 6). Means having superscripts of different letters [lower case (a–c): early larva and midgut; upper case (A–B): serum] represent a significant difference from each other within identical tissue (p < 0.05).
![Figure 2. Changes in hydrogen peroxide content (nmol/mg protein) in (a), whole-body homogenate of early larval stages and (b), serum and midgut of 4th and 5th instar of Antheraea mylitta. Data are expressed as mean ± SEM (n = 6). Means having superscripts of different letters [lower case (a–c): early larva and midgut; upper case (A–B): serum] represent a significant difference from each other within identical tissue (p < 0.05).](/cms/asset/09d34f61-9b08-4665-be64-94b51b46e2c7/tizo_a_1103319_f0002_b.gif)
Changes in activities of antioxidant enzymes
Superoxide dismutase (, )
Changes in SOD activity during larval development of Antheraea mylitta exhibited a biphasic pattern. During early phases of larval development (1st–3rd instars), the highest SOD activity, observed in 1st instar larvae, gradually diminished until they attended 3rd instar (). However, in the advanced larvae (4th–5th instars), a reverse trend was observed. Its activity was significantly increased in both tissues during larval transformation from 4th to 5th instar ().
Figure 3. Changes in superoxide dismutase activity (units/mg protein) in (a), whole-body homogenate of early larval stages and (b), serum and midgut of 4th and 5th instar. Data are expressed as mean ± SEM (n = 6). Means having superscripts of different letters [lower case (a–c): early larva and midgut; upper case (A–B): serum] represent a significant difference from each other within identical tissue (p < 0.05).
![Figure 3. Changes in superoxide dismutase activity (units/mg protein) in (a), whole-body homogenate of early larval stages and (b), serum and midgut of 4th and 5th instar. Data are expressed as mean ± SEM (n = 6). Means having superscripts of different letters [lower case (a–c): early larva and midgut; upper case (A–B): serum] represent a significant difference from each other within identical tissue (p < 0.05).](/cms/asset/897aea8b-f931-4c8e-8b4c-9b1fa1aa5e71/tizo_a_1103319_f0003_b.gif)
Catalase (, )
CAT activity was considerably high in the WB homogenate of 1st instar larvae, and subsequently declined during larval progression up to 3rd instar (), and, therefore, the observed changes in CAT activity were comparable to those of SOD in the ontogeny of early larvae. In the advanced larvae, CAT activity was observed only in MG tissues, without any significant difference between 4th and 5th instars (). It is worth mentioning that, under identical assay conditions, CAT activity was below the detection level in the serum of the advanced larvae.
Figure 4. Changes in catalase activity (pkat/mg protein) in (a), whole-body homogenate of early larval stages and (b), midgut of 4th and 5th instar larvae. Data are expressed as mean ± SEM (n = 6). Means having superscripts of different lower case letters (a–c) represent a significant difference from each other within identical tissue (p < 0.05).
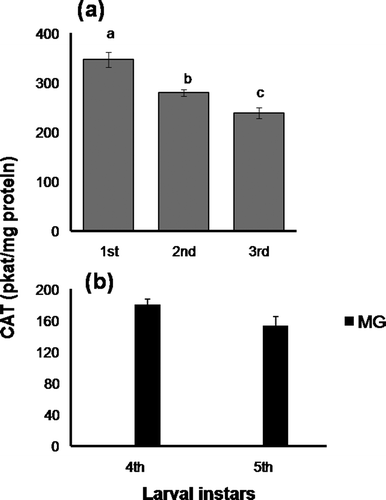
Glutathione S-transferase (, )
No linearity was observed in GST activity during the larval development of this species (, ). Its activity increased significantly during larval transformation from 1st to 2nd instar. During the late phase of larval development it also exhibited tissue-specific changes; i.e. in serum, high GST activity observed in the 4th instar declined in the 5th instar. In contrast, its activity was augmented significantly in the midgut tissues during larval progression from 4th to 5th instar.
Figure 5. Changes in glutathione S-transferase activity (nmol CDNB conjugate formed/min/mg protein) in (a), whole-body homogenate of early larval stages and (b), serum and midgut of 4th and 5th instar larvae. Data are expressed as mean ± SEM (n = 6). Means having superscripts of different letters [lower case (a–b): early larva and midgut; upper case (A–B): serum] represent a significant difference from each other within identical tissue (p < 0.05).
![Figure 5. Changes in glutathione S-transferase activity (nmol CDNB conjugate formed/min/mg protein) in (a), whole-body homogenate of early larval stages and (b), serum and midgut of 4th and 5th instar larvae. Data are expressed as mean ± SEM (n = 6). Means having superscripts of different letters [lower case (a–b): early larva and midgut; upper case (A–B): serum] represent a significant difference from each other within identical tissue (p < 0.05).](/cms/asset/5de3b753-9b2c-47b5-8e21-4ce3a74d8a45/tizo_a_1103319_f0005_b.gif)
Changes in non-enzymatic antioxidants
Glutathione (GSH) content (, )
Among the early larval stages, the lowest GSH content was observed in the WB homogenate of 1st instar larvae and, subsequently, its level increased progressively up to 3rd instar (). In the advanced larvae, changes in GSH content were found to be tissue-specific. Its content increased only in the MG tissues during larval progression from 4th to 5th instar ().
Figure 6. Changes in glutathione content (µmol/g tissue wet wt) in (a), whole-body homogenate of early larval stages and (b), GSH content in serum (µmol/mL) and midgut (µmol/g tissue wet wt) of 4th and 5th instar. Data are expressed as mean ± SEM (n = 6). Means having superscripts of different letters [lower case (a–b): early larva and midgut; upper case (A–B): serum] represent a significant difference from each other within identical tissue (p < 0.05).
![Figure 6. Changes in glutathione content (µmol/g tissue wet wt) in (a), whole-body homogenate of early larval stages and (b), GSH content in serum (µmol/mL) and midgut (µmol/g tissue wet wt) of 4th and 5th instar. Data are expressed as mean ± SEM (n = 6). Means having superscripts of different letters [lower case (a–b): early larva and midgut; upper case (A–B): serum] represent a significant difference from each other within identical tissue (p < 0.05).](/cms/asset/7ad8b79c-a75c-4595-9247-cab7df9a13ed/tizo_a_1103319_f0006_b.gif)
Ascorbic acid content (, )
A very high level of ASA was observed in the 1st instar larvae, which declined significantly in the 2nd instar and then maintained up to the 3rd instar (). In the later part of the development, ASA content was found to be higher in both tissues when the larvae were transformed from 4th to 5th instar ().
Figure 7. Changes in ascorbic acid content in whole-body homogenate (µg/g tissue wet wt) of (a), early larval stages and (b), serum (µg/mL) and midgut (µg/g tissue wet wt) of 4th and 5th instar. Data are expressed as mean ± SEM (n = 6). Means having superscripts of different letters [lower case (a–b): early larva and midgut; upper case (A–B): serum] represent a significant difference from each other within identical tissue (p < 0.05).
![Figure 7. Changes in ascorbic acid content in whole-body homogenate (µg/g tissue wet wt) of (a), early larval stages and (b), serum (µg/mL) and midgut (µg/g tissue wet wt) of 4th and 5th instar. Data are expressed as mean ± SEM (n = 6). Means having superscripts of different letters [lower case (a–b): early larva and midgut; upper case (A–B): serum] represent a significant difference from each other within identical tissue (p < 0.05).](/cms/asset/503960a4-a748-4ddc-ba23-f8a1340f7c68/tizo_a_1103319_f0007_b.gif)
Phytochemical constituents of the host plant, Terminalia arjuna
Phytochemical constituents of the host plant leaves (T. arjuna) analysed in this study are shown in .
Table I. Protein content, total phenolics, ascorbic acid, glutathione and hydrogen peroxide level in the leaf of the host plant, Terminalia arjuna. Data are expressed as mean ± SEM (n = 6).
Linear regression analysis (, , )
Regression analyses of different parameters have also been conducted to establish a possible correlation among the components of the antioxidant defence system of A. mylitta. A negative correlation (R2 = 0.8174, r = −0.9041) was observed between GSH content and SOD activity in the whole-body tissue of early instars (). In contrast, a regression analysis of the data between ASA and H2O2 (), and GSH and H2O2, () revealed a different trend. A strongly positive correlation between ASA and H2O2 (R2 = 0.8568, r = 0.9256) was observed in the whole-body homogenates of early instars; however, there is a negative correlation between GSH and H2O2 content (R2 = 0.6242, r = −0.7900).
Discussion
A comparison of levels of LPx and H2O2 contents in various stages of larval development indicated that 1st instar larvae were highly susceptible to LPx and were challenged with a relatively higher level of oxidative threat. A considerably higher level of LPx and H2O2 in the 1st instar larvae is consistent with the recent findings of Jena et al. (Citation2013) regarding this insect, which could be due to the increased production and/or decreased neutralisation of ROS. The comparatively high level of H2O2 in the 1st instar larvae of A. mylitta substantiates the above findings and are also in agreement with the recent observation of Knoefler et al. (Citation2012). The authors noted spiking of ROS, particularly H2O2, during the early development and moulting of Caenorhabditis elegans, where dual oxidase (DUOX), an enzyme involved in cuticle formation, was presumed to generate H2O2. Hu et al. (Citation2013) also documentated the role of DUOX in the silkworm, Bombyx mori (BmDuox), in the production of ROS. Silkworm larvae, before hatching (advanced embryonic stage), receive oxygen through a permeable egg membrane. However, after hatching, the 1st instar hatchlings are directly exposed to atmospheric oxygen and they need to form cuticle. Besides, the 1st instar larvae of A. mylitta started feeding on the leaves of the host plant, Terminalia arjuna. It is therefore presumed that direct exposure to ambient O2, initiation of herbivory, consumption of relatively high level of O2 [due to onset of feeding, and possibly due to the activity of dual oxidase (DUOX) for the formation of the cuticle] might have resulted in increased ROS generation. Findings of this investigation can be correlated with the results of Slama (Citation2000), who observed that during the larval development of the desert locust, Schistocerca gregaria, 1st instar feeding larvae usually consume a large amount of O2 in comparison with their pharate larvae enclosed within the egg shell. The author also noticed the highest metabolic rate in the 1st instar larvae compared to that in the other stages of their entire life cycle. Rath et al. (Citation2006) also reported that 1st instar larvae of Antheraea mylitta are metabolically active, and the metabolic efficiency decreases as the larva ages. Higher levels of H2O2 concurrent with high SOD and CAT activities in the WB homogenate of 1st instar larvae indicate such a possibility and also support this fact. It may also be noted here that lepidopteron insects generally possess a high quantity of polyunsaturated fatty acids (PUFA). In a related study, Wang et al. (Citation2006) observed the predominance of PUFA such as linolenic acid (18:3 n-3) and linoleic acid (18:2 n-6) in the larvae of the butterfly Morpho peleides. Therefore, the abundance of PUFA in the larvae may also be an additional factor responsible for its susceptibility to lipid peroxidation.
The reduced level of LPx in subsequent larval stages (2nd and 3rd instars) and in both of the tissues of advanced larvae associated with the decline in H2O2 content implies that the oxidative stress of larvae declines with advancement of larval stages. In the advanced larvae (4th and 5th instars) pro-oxidative pressure and the relative level of antioxidant protection were tissue-specific, as MG and serum displayed differential activities for antioxidant enzymes and contents of non-enzymatic antioxidants. It was observed that serum of 4th and 5th instar larvae exhibited higher level of H2O2 than the midgut did. As CAT was below the detection level in the serum (Sahoo et al. Citation2015), it is presumed that the slow removal of H2O2 from the serum by alternative antioxidant mechanisms (probably by ascorbate peroxidase/GSH) might have led to the accumulation of H2O2 in the tissue concerned. Lukasik et al. (Citation2011) also reported an alternative compensatory mechanism (GSH–ASA), which is important for protecting insects against oxidative stress. The level of LPx observed in the serum of 4th instar larvae also indicated a higher pro-oxidative burden on the tissue, and can be linked to a high level of H2O2 in 4th instar larvae.
Among the antioxidant enzymes, relatively enhanced activities of SOD and CAT were observed in 1st instar larvae, which slowly declined during larval progression from 1st to 3rd instar. Considerably higher activity of SOD and CAT in 1st instar larvae may be necessary to deal with high level of ROS, particularly H2O2. Insects have been reported to adapt quickly to elevated oxidative stress arising from plant pro-oxidant allelochemicals through up-regulation of antioxidant enzymes (Krishnan & Kodrik Citation2006). Dmochowska Slezak et al. (Citation2015), also observed the enhanced activities of antioxidant enzymes in the larval stages of the solitary bee Osmia bicornis. In a recent study, Jena et al. (Citation2014) also observed upregulation of SOD, CAT and GST activity in the MG tissues of Nosema-infected larvae of A. mylitta. They also opined that it is an adaptive antioxidant response of the tissue to elevated oxyradicals. The results of our investigation also strongly corroborate the findings of Aslanturk et al. (Citation2011). They observed the up-regulation of SOD and CAT activity in the midgut tissues of Lymantria dispar (Lepidoptera) larvae exposed to methidathion. These authors also thought that increase in SOD activity might have resulted in increased H2O2 concentration and, subsequently, in a further increase in CAT activity. In this study, we also observed a similar trend in the activities of SOD, CAT and H2O2 content in the 1st instar larvae, suggesting that these two enzymes act in concert and may influence the cellular level of H2O2. The increase in SOD activity in the serum and MG tissues of the advanced larvae during their transformation from 4th to 5th instar might be due to their increased consumption of plant allelochemicals (Pritos et al. Citation1988; Lee & Berenbaum Citation1990; Aucoin et al. Citation1991; Felton & Duffey Citation1992) on account of their voracious feeding habits. Rath et al. (Citation2006) observed that the larvae of Antheraea mylitta ingest more than 90% of the total intake during the last two instars, and particularly in the 5th instar, it is around 80%. A similar trend was also observed by Rana et al. (Citation1987) in oak silkworm, Antheraea proyeli. Comparatively, the higher SOD activity in the MG tissues than in serum can be linked to two different physiological aspects. Differential metabolic status of the tissue concerned, i.e. MG being metabolically more active than serum (Ahmad Citation1992), and increased exposure of midgut tissue to redox-active plant allelochemicals might have resulted in higher SOD activity due to the generation of a higher amount of superoxide radicals in the gut environment. Krishnan and Kodrik (Citation2006), in their study on gut antioxidant enzymes of Spodoptera littoralis caterpillars, concluded that polyphenolics (chlorogenic acid and tannin) present in the host plant leaves may substantially contribute to the production of oxidative radicals. These authors also observed that SOD activity was specifically increased in the MG tissues of caterpillars following the ingestion of polyphenolic compounds. In a related study, Doorika and Ananthi (Citation2012) reported that aqueous extract of Terminalia arjuna bark substantially improves the SOD activity and increases GSH content in isonised-induced damaged liver of albino rats. Raghavan and Kumari (Citation2006) also observed improvement in the level of antioxidant protection along with elevated GSH content in alloxan-induced diabatic rats in response to bark extract of Terminalia arjuna.
It is interesting to note that GSH content was enhanced during larval development with simultaneous induction of GST, particularly in 2nd instar larvae and in the MG of advanced larvae (5th instar). The insect digestive tract, especially the MG, is an important organ not only for food digestion but also in defence against toxicity of xenobiotics and entomopathogens (Xu et al. Citation2014). GST has been reported to cause reduction of a wide range of hydroperoxides (Lukasik & Golawska Citation2007), and as an important detoxifying enzyme against ROS (Gerardo et al. Citation2010; Ramsey et al. Citation2010). High activity of GST has also been reported in herbivore insects (Krishnan & Kodrik Citation2006). Thiboldeaux et al. (Citation1998) has correlated the elevation of GSH with growth and development in Saturniidae moth larvae. Petric-Mataruga et al. (Citation1997) suggested that upregulation of the level of GSH in herbivores may be one of the rapid forms of adaptive responses to nutritive and oxidative stress. Therefore, it is possible that induction of GSH and GSH-dependent enzyme (GST) is associated with the attenuation of oxidative stress during larval progression. The decline in SOD activity and increase in GSH content during the early stages of larval development suggest their complementary functions. The regression analysis of GSH/SOD (R2 = 0.8174; r = −0.9041, ) also strongly indicates their compensatory role during the larval development of this species. It is an established fact that GSH and SOD exhibit compensatory and complementary functions in the elimination of superoxide (Hussain et al. Citation1996) and other free radicals (Winterbourn Citation1995). Other than antioxidant properties, GSH has been reported to play an important role in the process of cell division and differentiation, and evidence also suggests that it is an important factor which regulates the onset of mitosis (Gardiner & Reed Citation1995).
The positive correlation between ASA and H2O2 (R2 = 0.8568; r = 0.9256, ) and the negative correlation between GSH and H2O2 (R2 = 0.6242; r = −0.7900, ) suggest the combined effect of GSH and ASA on the neutralisation of H2O2 during early larval development. The findings of this study corroborate our earlier report on the pupal fatbody of this insect, where we observed the role of ascorbic acid in the neutralisation of H2O2 following inhibition of catalase by aminotriazole (Patra et al. Citation2014). GSH and ASA are important for their protective action against ROS; they form a powerful redox couple to neutralise varieties of ROS in biological systems including insects (Krishnan et al. Citation2009). Findings of this investigation are also consistent with the previous report of Galovic et al. (Citation2004) on European corn borer, O. nubilalis, where a similar compensatory mechanism of this redox couple (GSH–ASA) was observed. In advanced larvae, we observed the elevation of ASA in both tissues, and GSH content was particularly increased in the MG. We presume that the elevation of GSH in the MG is a protective measure to minimise oxidative damage in the tissue concerned, probably caused by increased consumption of foliar content. Barbehenn (Citation2003) observed a high level of nonenzymatic antioxidants, particularly GSH, in the midgut tissues of polyphagous grasshoppers, and expressed the view that it is an adaptive response caused by elevated oxidative stress. High levels of low-molecular-mass antioxidants (glutathione, ascorbic acid, Vitamin E, etc.) are characteristic of insects, and appear to efficiently neutralise the deleterious effect of ROS (Blagojevic Citation1995; Barbehenn Citation2003). ASA is essential for normal growth, reproduction and development in insects (Lukasik et al. Citation2011). Nevertheless, as insects are unable to synthesise ascorbic acid, (Krishnan & Kodrik Citation2012), the increased ASA content in both tissues of advanced larvae observed in the present experiment can be linked to the sequestration of this antioxidant from the host plant through intense feeding.
Conclusions
The overall findings of this investigation suggest that in the early larval stages, 1st instar larvae are more susceptible to oxidative threat, as is evident from MDA content (LPx) and H2O2 levels in the WB homogenate of the early larvae. Direct exposure to ambient oxygen, initiation of larval feeding containing plant allelochemicals, and cuticle formation for 1st instar larvae (which require H2O2-generating dual oxidase enzyme) may be the reasons for higher levels of oxidative burden. As the larvae aged, oxidative stress levels were found to be attenuated with a strategic rise in specific antioxidant protections, as evident from the antioxidant profile in the whole-body homogenate of 2nd and 3rd instar larvae. It is further observed that 1st instar larvae are protected by enzymatic antioxidants from SOD and CAT. In contrast, advanced larvae receive a combined antioxidant protection from both enzymatic and nonenzymatic sources. The progressive rise in the GSH level during larval development suggests its involvement in the process of cellular development and differentiation, besides its antioxidant role. However, the patterns of changes in GSH and ASA, particularly during the early stages of larval development, indicate that the utilisation of GSH and ASA for the neutralisation of ROS is compensatory. This substantiates the role of the GSH–ASA redox couple for the maintenance of the cellular redox balance. In conclusion, it is clearly evident that oxidative stress indices during larval development of A. mylitta are stage- and tissue-specific, and are influenced by the metabolic status and feeding behaviour of the caterpillars. Accordingly, the role of antioxidant defences is compensatory and is modulated by the cellular redox status to provide protection to the developing larvae.
Acknowledgements
The authors are thankful to Prof. S. K. Dutta, former head, Department of Zoology, North Orissa University, Baripada, India, and Prof. G. B. N. Chainy, former head, Department of Zoology and Biotechnology, Utkal University, Bhubaneswar, India, for the provision of laboratory facilities, which enabled us to complete this work. We thank Mr. Jajati Rout, Senior Technical Inspector, State Silk Board (Baripada, Odisha), for providing the larvae. Financial assistance from the Department of Biotechnology, the Government of India and a DST-PURSE grant (DST-India) to the P.G. Department of Biotechnology, Utkal University, is gratefully acknowledged. We are sincerely grateful to Professor Jatin K. Nayak, Department of English, Utkal University, Bhubaneswar, for copy editing this manuscript.
References
- Abraham G, Thomas G, Babu CR. 2004. Induction of biomolecules in mature leaves of Terminalia arjuna due to feeding of Antheraea mylitta Drury. Science World Journal 4:887–891. doi:10.1100/tsw.2004.143.
- Aebi H. 1974. Catalase. In: Bergmayer HU, editor. Methods in Enzymatic Analysis, Vol. II. New York: Academic Press. pp. 673–678.
- Ahmad S. 1992. Biochemical defence of pro-oxidant plant allelochemicals by herbivorous insects. Biochemical Systematics and Ecology 20:269–296. doi:10.1016/0305-1978(92)90040-K.
- Allen RG. 1991. Oxygen reactive species and antioxidant responses during development: The metabolic paradox of cellular differentiation. Proceeding of the Society for Experimental Biology and Medicine 196:117–129. doi:10.3181/00379727-196-43171A.
- Aslanturk A, Kalender S, Uzunhisarcikli M, Kalender Y. 2011. Effects of methidathion on antioxidant enzyme activities and malonaldehyde level in midgut tissues of Lymantria dispar (Lepidoptera) larvae. Journal of Entomological Research Society 13:27–38.
- Aucoin RR, Philogene BJR, Arnason JT. 1991. Antioxidant enzymes as biochemical defenses against phototoxin-induced oxidative stress in three species of herbivorous lepidoptera. Archives of Insect Biochemistry and Physiology 16:139–152. doi:10.1002/(ISSN)1520-6327.
- Barbehenn RV. 2003. Antioxidants in grasshoppers: Higher levels defend the midgut tissues of a polyphagous species than a graminivorous species. Journal of Chemical Ecology 29:683–702. doi:10.1023/A:1022824820855.
- Bhatia NK, Yousuf M. 2014. Effect of rearing season, host plants and their interaction on economical traits of tropical tasar silkworm, Antheraea mylitta Drury- an overview. International Journal of Industrial Entomology 29:93–119. doi:10.7852/ijie.2014.29.1.93.
- Bi JL, Felton GW. 1995. Foliar oxidative stress and insect herbivory: Primary compounds, secondary metabolites and reactive oxygen species as components of induced resistance. Journal of Chemical Ecology 21:1511–1530. doi:10.1007/BF02035149.
- Biewenga GP, Haenen GRMM, Bast A. 1997. The pharmacology of the antioxidant lipoic acid. General Pharmacology 2:315–331. doi:10.1016/S0306-3623(96)00474-0.
- Blagojevic D. 1995. The role of antioxidant protection in senescence of the bean weevil. Ph.D. thesis, University of Belgrade.
- Blokhina OE, Virolainen K, Fagerstedl V. 2003. Antioxidants, oxidative damage and oxygen deprivation stress: A review. Annals of Botany 91:179–194. doi:10.1093/aob/mcf118.
- Brinster RL. 1968. Effect of glutathione on the development of two-cell mouse embryos invitro. Journal of Reproduction and Fertility 17:521–525. doi:10.1530/jrf.0.0170521.
- Chance B, Sies H, Boveries A. 1979. Hydroperoxide metabolism in mammalian organs. Physiological Reviews 59:527–605.
- Chippendale GM. 1975. Ascorbic acid: An essential nutrient for a plant feeding insect, Diatraea graniosella. Journal of Nutrition 105:449–507.
- Cross CE, Halliwell B, Borish ET, Pryor WA, Ames BN, Saul RL, Mc Cord JM, Harman D. 1987. Oxygen radicals and human disease. Annals of Internal Medicine 107:526–545. doi:10.7326/0003-4819-107-4-526.
- Dandapat J, Chainy GBN, Rao KJ. 2003. Lipid peroxidation and antioxidant defence status during larval development and metamorphosis of giant prawn, Macrobrachium rosenbergii. Comparative Biochemistry and Physiology C 135:221–233.
- Das K, Samanta L, Chainy GBN. 2000. A modified spectrophotometric assay of superoxide dismutase using nitrite formation of superoxide radicals. Indian Journal of Biochemistry and Biophysics 37:201–204.
- Dmochowska Slezak K, Giejdasz K, Fliszkewicz M, Zoltowska K. 2015. Variations in antioxidant defence during the development of the solitary bee Osmia bicornsis. Apidologie 46:432–444. doi:10.1007/s13592-014-0333-y.
- Doorika P, Ananthi T. 2012. Antioxidant and hepatoprotective properties of Terminalia arjuna bark on isonized induced toxicity in albino rats. Asian Journal of Pharmacology and Technology 2:15–18.
- Ellman GL. 1959. Tissue sulfhydril groups. Archives of Biochemistry and Biophysics 82:72–77. doi:10.1016/0003-9861(59)90090-6.
- Felton GW, Duffey SS. 1992. Ascorbate oxidation reduction in Helicoverpa zea as a scavenging system against dietary oxidants. Archives of Insect Biochemistry Physiology 19:27–37. doi:10.1002/(ISSN)1520-6327.
- Fridovich I. 1978. The biology of oxygen radicals. Science 201:875–880. doi:10.1126/science.210504.
- Galovic AJ, Blagojevic DP, Lajsic GG, Worland R, Spasic MB. 2004. Role of antioxidant defence during different stages of preadult life cycle in European corn borer (Ostrinia nubilalis, Hubn.): Diapause and metamorphosis. Insect Biochemistry and Physiology 55:79–89. doi:10.1002/arch.10126.
- Garcia DH, Wood DC, Obregon SC, Covarrubias L. 2010. Reactive oxygen species: A radical role in development. Free Radical Biology and Medicine 49:130–143. doi:10.1016/j.freeradbiomed.2010.03.020.
- Gardiner CS, Reed DJ. 1995. Synthesis of glutathione in the preimplantation mouse embryo. Archives of Biochemistry and Biophysics 318:30–36. doi:10.1006/abbi.1995.1200.
- Gerardo NM, Altincicek B, Anselme C, Atamian H, Barribeau SM, De Vos M, Duncan EJ, Evans JD, Gabaldón T, Ghanim M, Heddi A, Kaloshian I, Latorre A, Moya A, Nakabachi A, Parker BJ, Pérez-Brocal V, Pignatelli M, Rahbé Y, Ramsey JS, Spragg CJ, Tamames J, Tamarit D, Tamborindeguy C, Vincent-Monegat C, Vilcinskas A. 2010. Immunity and other defences in pea aphid, Acyrtosiphon pisium. Genome Biology 11:R21. doi:10.1186/gb-2010-11-2-r21.
- Gulevsky AK, Relina LI, Grishchenkova YA. 2006. Variations of the antioxidant system during development of the cold-tolerant beetle, Tenebrio molitor. Cryoletters 27:283–290.
- Habig WH, Jakoby WB. 1981. Glutathione S-transferases (rat and human). Methods in Enzymology 77:218–231.
- Halliwell B, Gutteridge JM. 1992. Free radicals, antioxidants and human disease: Where are we now? Journal of Laboratory and Clinical Medicine 119:598–620.
- Hazelton GA, Lang CA. 1983. Glutathione S-transferase activities in the yellow fever mosquito (Aedes aegypti-Loisville) during growth and aging. Biochemical Journal 210:281–287. doi:10.1042/bj2100281.
- Hu X, Yang R, Zhang X, Chen L, Xiang X, Gong C, Wu X. 2013. Molecular cloning and functional charecterization of the dual oxidase (BmDuox) gene from the Silkworm Bombyx mori. Plos One 8:e70118. doi:10.1371/journal.pone.007011.
- Hussain S, Slikker W Jr, Ali SF. 1996. Role of metallothionein and other antioxidants in scavenging superoxide radicals and their possible role in neuroprotection. Neurochemistry International 29:145–152. doi:10.1016/0197-0186(95)00114-X.
- Jagota SK, Dani HM. 1982. A new colorimetric technique for the estimation of vitamin C using folin phenol reagent. Analytical Biochemistry 127:178–182. doi:10.1016/0003-2697(82)90162-2.
- Jena K, Kar PK, Babu CS, Giri S, Singh SS, Prasad BC. 2013. Comparative study of total hydroperoxides and antioxidant defence system in the tropical tasar silkworm, Antheraea mylitta, in diapausing and non-diapausing generations. Journal of Insect Science 13:1–11. doi:10.1673/031.013.12301.
- Jena K, Pandey JP, Sinha AK. 2014. Oxidative stress and antioxidant defences in the tasar silkworm Antheraea mylitta D: Challenged with Nosema species. International Journal of Industrial Entomology 28:85–91. doi:10.7852/ijie.2014.28.2.85.
- Klichko VI, Radyuk SN, Orr WC. 2004. Profiling catalase gene expression in Drosophila melanogaster during development and aging. Archives of Insect Biochemistry and Physiology 56:34–50. doi:10.1002/(ISSN)1520-6327.
- Knoefler D, Thamsen M, Koniczek M, Niemuth NJ, Diederich A, Jakob U. 2012. Quantitative in vivo redox sensors uncover oxidative stress as an early event in life. Molecular Cell 47:767–776. doi:10.1016/j.molcel.2012.06.016.
- Kostarpoulos I, Papadopoulos AI. 1998. Glutathione S-transferase isoenzymes expressed in the three developmental stages of the insect Tenebrio molitor. Insect Biochemistry and Molecular Biology 28:901–909. doi:10.1016/S0965-1748(98)00057-5.
- Kostarpoulos I, Papadopoulos AI, Metaxakis A, Boukouvala E, Papadopolulou- Mourkidou E. 2001. Glutathione S-transferase in the defence against pyrethroids in insects. Insect Biochemistry and Molecular Biology 31:313–319. doi:10.1016/S0965-1748(00)00123-5.
- Kotze AC, Rose HA. 1987. Glutathione S-transferase in the Australian blowfly, Lucilia cuprina (Wiedemann). Pesticide Biochemistry and Physiology 29:77–86. doi:10.1016/0048-3575(87)90086-1.
- Krishnan N, Kodrik D. 2006. Antioxidant enzymes in Spodoptera littoralis (Boisduval): Are they enhanced to protect gut tissues during oxidative stress? Journal of Insect Physiology 52:11–20. doi:10.1016/j.jinsphys.2005.08.009.
- Krishnan N, Kodrik D. 2012. Endocrine control of oxidative stress in Insect. In: Farooqui T, Farooqui AA, editors. Oxidative stress in vertebrates and invertebrates. Hoboken, NJ: Wiley- Blackwell. pp. 261–270.
- Krishnan N, Kodrik D, Kludkiewicz B, Sehnal F. 2009. Glutathione-ascorbic acid redox cycle and thiredoxin reductase activity in the digestive tract of Leptinotarsa decemlineata (Say). Insect Biochemistry and Molecular Biology 39:180–188. doi:10.1016/j.ibmb.2008.11.001.
- Lee K, Berenbaum MR. 1990. Defence of parsnip webworm against phototoxic furanocoumarins: Role of antioxidant enzymes. Journal of Chemical Ecology 16:2451–2460. doi:10.1007/BF01017468.
- Lowry OH, Resebrough NJ, Farr AL, Randall RJ. 1951. Protein measurement with the folin phenol reagent. The Journal of Biological Chemistry 193:265–275.
- Lukasik I, Golawska S. 2007. Activity of Se-independent glutathione peroxidase and glutathione reductase within cereal aphid tissues. Biological Letter 4:31–39.
- Lukasik I, Golawska S, Wojcicka A, Golawski A. 2011. Effect of host plants on antioxidant system of Pea aphid Acyrthosiphon pisum. Bulletin of Insectology 64:153–158.
- Meister A. 1994. Mini review: Glutathione- ascorbic acid antioxidant system in animals. Journal of Biological Chemistry 269:9397–9400.
- Nagaraju J, Goldsmith MR. 2002. Silkworm genomics-progress and prospects. Current Science 48:415–425.
- Ohkawa H, Ohishi N, Yagi K. 1979. Assay for lipid peroxides in animal tissues by thiobarbituric acid reaction. Analytical Biochemistry 95:351–358. doi:10.1016/0003-2697(79)90738-3.
- Orr WC, Sohal RS. 1994. Extension of life span by over expression of superoxide dismutase and catalase in Drosophila melanogaster. Science 263:1128–1130. doi:10.1126/science.8108730.
- Patra B, Sahu S, Dandapat J. 2014. Effect of aminotriazole on antioxidant defense system of tasar silkworm Antheraea mylitta. Entomological Science 17:316–323. doi:10.1111/ens.2014.17.issue-3.
- Petric-Mataruga V, Blagojevic D, Spasic MB, Ivanovic J, Jankovic-Hladni M. 1997. Effect of the host plant on the antioxidative defence in the midgut of Lymantria dispar L. caterpillars of different population origins. Journal of Insect Physiology 43:101–106. doi:10.1016/S0022-1910(96)00018-2.
- Pick E, Keisari Y. 1981. Superoxide anion and hydrogen peroxide production by chemically elicited peritoneal macrophages. Induction by multiple nonphagocytic stimuli. Cellular Immunology 59:301–318. doi:10.1016/0008-8749(81)90411-1.
- Pinnell S. 2003. Cutaneous photodamage, oxidative stress, and topical antioxidant protection. Journal of the American Academy of Dermatology 48:1–22. doi:10.1067/mjd.2003.16.
- Pritos CA, Ahmad S, Bowen SM, Elliot AJ, Blomquist GJ, Pardini RS. 1988. Antioxidant enzymes of the black swallowtail butterfly, Papilio polyxenes, and their response to the pro-oxidant allelochemical, quercetin. Archives of Insect Biochemistry Physiology 8:101–112. doi:10.1002/arch.940080204.
- Raghavan B, Kumari SK. 2006. Effect of Terminalia arjuna stem bark on antioxidant status in liver and kidney of alloxan diabetic rats. Indian Journal of Physiology and Pharmacology 50:133–142.
- Ramsey JS, Rider DS, Walsh T, de Vos M, Gordon K, Ponnala L, Roe BA, Jander G. 2010. Comparative analysis of detoxification enzymes in Acrythrosiphon pisum and Myzus persicae. Insect Molocular Biology 19:155–164. doi:10.1111/j.1365-2583.2009.00973.x.
- Rana B, Prasad B, Nigam MP. 1987. Consumption and utilization of food by oak tasar silkworm Antheraea proyeli Jolly (Lepidoptera: Saturniidae). Sericologia 27:11–19.
- Rath SS. 2010. Food utilization efficiency in Antheraea mylitta fed on Terminalia arjuna Leaves. Academic Journal of Entomology 3:23–28.
- Rath SS, Singh MK, Suryanaryana N. 2006. Change in rate of feeding and assimilation in Antheraea mylitta fed on two major food plants and its effect on silk production and reproduction. Agricultural Journal 1:24–27.
- Sahoo S, Dandapat J, Samanta L. 2015. Oxidative damaged products, level of hydrogen peroxide, and antioxidant protection in diapausing pupa of Tasar silk worm, Antheraea mylitta: A comparative study in two voltine groups. International Journal of Insect Science 7:11–17.
- Slama K. 2000. Correlation between metabolic depression and ecdysteroid peak during embryogenesis of the desert locust, Schistocerca gregaria (Orthoptera: Acrididae). European Journal of Entomology 97:141–148. doi:10.14411/eje.2000.026.
- Slinkard K, Singleton VL. 1977. Total phenolics analysis: Automation and comparison with manual method. American Journal of Enology and Viticulture 28:49–55.
- Steenvoorden DP, Henegouwen V. 1997. GM: The use of endogenous antioxidants to improve photoprotection. Journal of Photochemistry and Photobiology B 41:1–10. doi:10.1016/S1011-1344(97)00081-X.
- Thiboldeaux RL, Lindroth RL, Tracy JW. 1998. Effects of juglone (5-hydroxy-1,4-naphthoquinone) on midgut morphology and glutathione status in Saturniid moth larvae. Comparative Biochemistry and Physiology C 120:481–487.
- Wang Y, Lin DS, Bolewicz L, Connor WE. 2006. The predominance of polyunsaturated fatty acids in the butterfly Morpho peleides before and after metamorphosis. The Journal of Lipid Research 47:530–536. doi:10.1194/jlr.M500346-JLR200.
- Wills ED. 1969. Lipid peroxide formation in microsomes: General considerations. Biochemical Journal 113:315–324. doi:10.1042/bj1130315.
- Winterbourn CC. 1995. Concerted antioxidant activity of glutathione and superoxide dismutase. In: Packer L, Fuchs J, editors. Biothiols in Health and Disease. New York, NY: Marcel Dekker Inc. pp. 117–134.
- Wood E, Casabé N, Melgar F, Zerba E. 1986. Distribution and properties of glutathione S-transferase from Triatoma infestans. Comparative Biochemistry and Physiology B 84:607–618.
- Xu ZB, Zou XP, Zhang N, Feng QL, Zheng SC. 2014. Detoxification of insecticides, allechemicals and heavy metals by glutathione S-transferase SlGSTE1 in the gut of Spodoptera litura. Insect Science. 22:503–511. doi:10.1111/1744-7917.12142.