Abstract
Dual biocontrol of both insects and plant pathogens has been reported for certain fungal entomopathogens, including Beauveria bassiana and Lecanicillum spp. In this study, we demonstrate, for the first time, the dual biocontrol potential of two fungal isolates identified by morphological and phylogenetic analyses as Isaria javanica. Both these isolates caused mortality in the greater wax moth, and hence can be considered entomopathogens. Spores of the isolates were also pathogenic to nymphs of the green peach aphid (Myzus persicae), with an LC50 value of 107 spores/mL 4 days after inoculation and an LT50 of 4.2 days with a dose of 108 spores/mL. In vitro antifungal assays also demonstrated a strong inhibitory effect on the growth of two fungi that are pathogenic to peppers, Colletotrichum gloeosporioides and Phytophthora capsici. These results indicate that I. javanica isolates could be used as novel biocontrol agents for the simultaneous control of aphids and fungal diseases, such as anthracnose and Phytophthora blight, in an integrated pest management framework for red pepper.
The rapid adoption of organic agricultural practices during the past decade has markedly increased the world-wide demand for plant biopesticides that can be used to control weeds, insect pests, and microbial/viral pathogens. In order to meet this need, the biopesticide industry is eagerly searching for new and effective active agents. Many plant-associated microbes act as plant probiotics, in part because they produce biopesticides [Citation1]. Biocontrol microbes are selected because of their antagonistic activity against a primary target, such as plant pathogens or harmful insects; however, many isolates have been found to have multiple beneficial effects on plant health. For example, certain bacterial biocontrol species, including species of Pseudomonas and Bacillus, enhance plant growth and induce systemic resistance, which can augment tolerance to plant diseases and abiotic stress [Citation2–6].
Many entomopathogenic fungal isolates have been commercialized world-wide in various formulations as mycoinsecticides for the control of phytophagous insects [Citation7]. However, certain entomopathogenic fungi are also known to directly inhibit plant microbial pathogens, induce systemic resistance, and enhance plant growth [Citation4]. These useful traits are associated with the production of antimicrobial and/or insecticidal products [Citation8,Citation9]. For example, the peptide isaferin, extracted from the mycelia of Isaria felina, is used as an insecticide for control of the oriental armyworm Leucania separata, and also functions as a mycocide in control the plant pathogenic fungi Rhizoctonia solani and Sclerotinia sclerotiorum [Citation10]. Furthermore, certain strains of Beauveria bassiana produce a broad-spectrum antimicrobial compound, beauvericin [Citation11,Citation12], which is also produced by other entomopathogens, including isolates of Paecilomyces, Isaria, and Fusarium [Citation13]. The production of beauvericin may also contribute to the suppression of damping-off caused by R. solani or Pythium myriotylum in tomato and cotton [Citation12]. Isolates of I. fumosoroseus and I. farinosus control powdery mildew through mycoparasitism of this obligate plant pathogen [Citation14,Citation15]. Furthermore, extracellular chitinase has been proposed as a key virulence factor in insect mortality caused by I. fumosorosea [Citation16]. Thus, secreted metabolites and enzymes may be involved in the control of insects and fungal pathogens.
Interestingly, some entomopathogenic fungi with the potential to control plant diseases, including species of Lecanicillum, Beauveria, and Metarhizium, are plant endophytes [Citation4,Citation11,Citation12,Citation17–19]. Given that no additional biocontrol agent applications are required once such isolates have colonized a plant, they are ideal for utilization as formulations in integrated pest management under field conditions [Citation20–22].
In the present study, we initially screened isolated fungi for their entomopathogenic activity, and subsequently examined whether they were inhibitory to the growth of plant pathogenic fungi, thereby establishing whether these isolates have dual biocontrol activity. On the basis of morphological and molecular phylogenic analyses, we identified two isolates of I. javanica with such biocontrol potential. These isolates showed potential for use in the field as part of cost- and labor-effective integrated pest management programs.
To isolate potential entomopathogenic fungi, 10 larvae of the greater wax moth Galleria mellonella were placed in sterile Petri dishes, each containing soil collected from various agricultural fields in Korea. The larvae of G. mellonella are model insects used to isolate microbial entomopathogens [Citation20]. After incubation for 14 d at 25 °C, infected larvae were suspended in sterile water by vortexing vigorously. To obtain pure fungal isolates, the suspension was serially diluted and plated out on potato dextrose agar (PDA; Difco Inc. Detroit, MI, USA) containing streptomycin (50 μg/mL) to prevent bacterial growth. Single fungal colonies were picked and cultivated on PDA. Segments of agar containing mycelium of the purified isolates grown on PDA plates were transferred to 10% sterile glycerol and stored at −80 °C.
Each isolate causing mortality in the moth larvae was initially examined for its ability to inhibit growth of the fungus Colletotrichum gloeosporioides (KACC 40896), obtained from the Korean Agricultural Culture Collection (KACC) National Agrobiodiversity Center, Wanju, South Korea. This fungus is the causal agent of anthracnose, one of the most important limiting factors for pepper production in Korea [Citation21]. The entomopathogenic fungal isolate and the pepper pathogen were simultaneously inoculated onto PDA by placing 7 mm2 plugs of the respective mycelia, previously grown on PDA, 5 cm apart from each other. Growth inhibition of the fungal pathogen was determined 7 d after initiating co-incubation. From an initial screen of over 200 different entomopathogenic fungal isolates, two isolates, pf185 and pf212, were selected for further study because they showed the strongest antifungal activity against C. gloeosporioides. These two isolates originated from soil collected in different regions of South Korea: isolate pf185 was isolated from soil collected from a location with GPS coordinates of latitude 36.496975 and longitude 126.361456, and isolate pf212 was isolated from soil collected at a location with GPS coordinates latitude 35.106851 and longitude 121.814057.
Phylogenic analysis of the isolated entomopathogens was based on sequences derived from two genomic regions, the internal transcribed spacer (ITS) of rRNA genes and a partial sequence of the gene encoding β-tubulin [Citation22,Citation23]. The fungi were grown in potato dextrose broth (PDB) for 5 d at 25 °C, with agitation at 150 rpm. Total genomic DNA was extracted from the fungal mass using a DNeasy Plant Mini Kit (Qiagen Inc., Valencia, CA, USA), according to the manufacturer’s instructions. Sequences were amplified by the polymerase chain reaction (PCR) using the primers ITS1 (5′-TCCGTAGGTGAACCTGCGG-3′) and ITS4 (5′-TCCGTAGGTGAACCTGCGG-3′) for the ITS region [Citation23], and Bt2a (5′-GGTAACCAAATCGGTGCTGCTTTC-3′) and Bt2b (5′-ACCCTCAGTGTAGTGACCCTTGGC-3′) for the tubulin gene [Citation22]. PCR reactions were performed using a Bioneer premix (Bioneer Inc., Daejeon, Korea), and products of approximately 560 bp and 330 bp were generated from the ITS region and the tubulin gene, respectively. The PCR products were purified using a purification kit (Bioneer) and cloned into a pGEM T-easy vector (Promega, Madison, WI, USA). Nucleotide sequences of the PCR products were generated by Solgent Inc. (Daejeon, Korea). These sequences have been deposited in GenBank (Supplemental ) as LY490787.1 (ITS) and MH340496 (β-tubulin) for pf185, and LY490786.1 (ITS) and MH340497 (β-tubulin) for pf212.
Table 1. Comparison of the size and shape of conidia from Isaria javanica isolates pf185 and pf212.
Initial analysis using BLASTN (available at the National Center for Biotechnology Information) using sequences for the ITS region and the partial gene sequences for beta-tubulin of both isolates showed identity with Isaria spp. Therefore, the PCR sequences were aligned with the sequences of Isaria sp. ex-type strains obtained from GenBank (Supplemental Table 1), using CLUSTAL X, and edited using BioEdit 7 [Citation24]. Phylogenetic trees were generated using MEGA 7 software [Citation25] based on the neighbor-joining method, with the Kimura two-parameter model (Kimura, 1980) and bootstrap values based on 1,000 replications. Phylogenetic analysis revealed that the ITS and β-tubulin sequences of both isolates were 99.99% identical to those of I. javanica ex-type CBS134.22 (). Interestingly, our phylogenic analysis indicated that I. javanica pf185 and pf212 belong to a distinct subgroup containing I. fumosorosea ex-type CBS244.31.
Figure 1. Phylogenetic trees based on the internal transcribed spacer (ITS, A) and partial ß-tubulin (TUB2, B) sequences of isolates pf185 and pf212 and ex-type strains of the Isaria genus (Supplemental Table 1). The phylogenetic trees were constructed using the neighbor-joining method and Kimura’s two-parameter model. The numbers at nodes indicate consensus bootstrap values based on 1,000 replications. Scale bar = 0.01 substitutions per nucleotide position. Sequences from Metarhizium anisopliae and M. brunneum were used as the outgroups for ITS and ß-tubulin sequence analyses, respectively. Abbreviations: ARSEF, Agricultural Research Service Collection of Entomopathogenic Fungal Cultures (Ithaca, NY, USA); CBS, Centraal Bureau voor Schimmelcultures (Utrecht, The Netherlands).
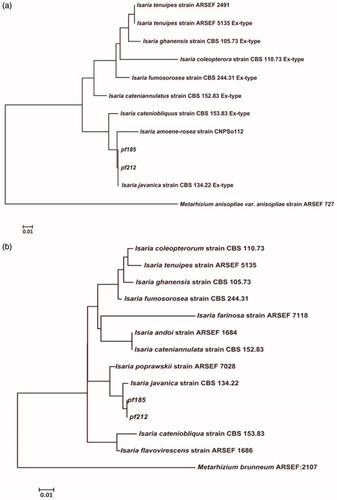
However, given that recent reports have indicated the incorrect identification of some Isaria isolates based on molecular analyses [Citation26,Citation27], we also examined the morphology of conidia and phialides for comparison with published data on isolates characterized as ex-types of the Isaria genus [Citation27,Citation28]. Isolates pf185 and pf212 were grown on PDA for 7 d at 25 °C to facilitate spore formation. Slides were prepared by suspending scrapes from these fungal cultures in sterile water, followed by light microscopy examination at ×200, ×400, and ×1,000 magnification (Leica M165 FC; Leica Microsystems, Wetzlar, Germany). For observation of phialides, samples were stained with lactophenol cotton blue with iodine glycerol reagent, as previously described [Citation29]. Images were photographed digitally using a Leica DFC 450 C camera and analyzed using an Image analyzer (IMT i-Solution Inc., Scarborough, Canada). At least 40–70 independent measurements of the lengths and widths of conidia (n = 40) and phialides (n = 70) were made for each isolate. Observations were performed twice and data were analyzed with Student’s t-test (p < 0.05) using SPSS (version 23; SPSS Inc., Chicago, IL, USA).
Morphological features of the pf185 and pf212 isolates and their comparison with the ex-type strain are summarized in and illustrated in . For pf185, spores were 4.9 ± 1.8 μm in length and 2.1 ± 0.5 μm in width (), whereas those for pf212 were longer at 7.2 ± 1.4 μm (t = −5.711, p < 0.0001) but similar in width at 2.3 ± 0.5 μm (t = −2.436, p = 0.017). Growth of other I. javanica isolates for 5 d on a different medium has been shown to result in conidial lengths of between 2 and 5 μm and widths of 1 to 2 μm [Citation27,Citation28]. However, all conidia were cylindrical to fusiform in shape, although unlike the conidia of other I. javanica isolates, the conidia from pf185 and pf212, were hyaline and not pink in color ().
Figure 2. Morphology of the conidia and phialides of Isaria javanica isolates pf185 and pf212. Phialides with developing conidial chains of I. javanica pf185 (A) and I. javanica pf212 (B). Typical shapes of individual conidia of I. javanica pf185 (C) and I. javanica pf212 (D). Scale bars represent 10 μm. The images are representative of two independent observations with at least 40 conidia and 70 phialides.
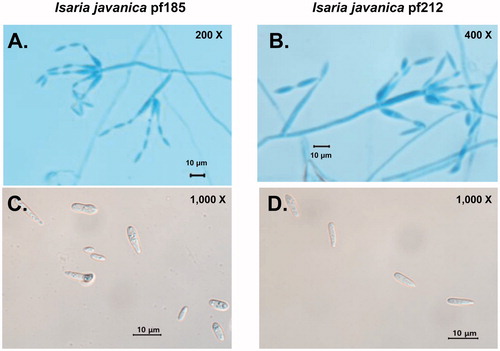
The phialides of the pf185 and pf212 isolates displayed a wide globose basal portion with a long distal neck, and overall lengths 8.0 ± 2.8 μm and 7.7 ± 1.8 μm, and widths of 1.6 ± 0.3 μm and 1.6 ± 0.4 μm, respectively ( and ). Although these measurements were not significantly different, (t = 0.691, p = 0.491 for length and t = 318, p = 0.190 for width) (), they tended to be longer and less wide than those of other I. javanica isolates, which showed lengths of 4–6 μm and widths of 2–3 μm [Citation27,Citation28]. Differences in growth conditions could account for the variability reported here. All phialides were smooth- and colorless, with few or no synnemata [Citation27,Citation28]. Consequently, combining information obtained from the phylogenic and morphological analyses, the pf185 and pf212 isolates were classified as isolates of I. javanica and have been deposited in the KACC with designated numbers KACC93241P and KACC93240P, respectively.
To extend our characterization of the antifungal activity of isolates pf185 and pf212, in vitro co-inoculation assays on PDA plates were conducted against additional phytopathogenic fungi, namely, Fusarium oxysporum (KACC 44452), a wilt pathogen of tomato, and Rhizoctonia solani (KACC 40111), the causative agent of damping-off in many crops. We also examined activity against the pepper phytophthora blight pathogen Phytophthora capsici (KACC 44716). Assays of growth inhibition caused by I. javanica isolates pf185 and pf212 on PDA were performed in vitro. Fungal growth was generally measured after a 7-d co-incubation, although for the fast-growing fungus R. solani, growth was recorded at 3 d. Growth of each fungal pathogen was measured as the average diameter of the colony size in four different directions. Percentage fungal growth inhibition was calculated as [(A − B)/A] × 100, where A is the radial growth of the fungi in the absence of I. javanica and B is the radial growth in plates inoculated with both test organism and I. javanica isolate. The experiment was performed twice, with each treatment being replicated five times. Data were analyzed with Student’s t-test (p < 0.05) using SPSS (version 23; SPSS Inc.).
In the dual growth assays, I. javanica pf185 was marginally more antagonistic than isolate pf212. Although inhibition was strongest against C. gloeosporioides, it was still evident against P. capsici. Substantially lower inhibition was observed against F. oxysporum, whereas no inhibition was observed against R. solani (), which is in contrast to previous reports for an isolate of I. felina [Citation10]. Thus, it will be useful to explore the extent of differences in the biocontrol potential of different Isaria genus isolates.
Results obtained for the aphicidal activities of isolates pf185 and pf212 against the green peach aphid (M. persicae) were essentially similar, and therefore here we only present the data for pf185. An aphid culture, obtained from Dr. Han of the Agricultural Microbiology Division, National Academy of Agricultural Science, was propagated on Chinese cabbage. Chinese cabbage seedlings were planted into sterile soil-less medium (peat moss:vermiculite:perlite, 7:3:3, vol/vol/vol) with one seedling per pot, which was watered every 2 d. Healthy apterous adult aphids were transferred onto young cabbage leaves, with approximately 20 aphids per leaf, and allowed to reproduce for 2 d to produce the 1–2-d-old second-instar nymphs used for bioassays. Sections of Chinese cabbage leaves (3.5 × 3.5 cm) were placed in an insect breeding dish (10 ×40 cm; SPL Science, Daejeon, Korea) containing sterile cotton, soaked in distilled water, and inoculated with 20 second-instar nymphs by transfer with a soft brush. Suspensions of I. javanica pf185 conidia diluted to contain 106–8 spores/mL were applied directly in 20-μL aliquots onto the nymphs using a micropipette. The conidia were obtained from cultures grown for 7 d at 25 °C on PDA by flooding the plates with sterile water followed by filtration through two layers of sterile cheese cloth to remove hyphal debris. Spore numbers were determined using a hemocytometer. After application of spore suspensions, or sterile water as a control, the insect breeding dishes were incubated at 25 °C and aphid mortality was monitored daily for 7 d. Nymphs were considered dead when they failed to move after multiple prods with a fine paint brush. Dead aphids were removed from the colonies and examined for overt mycosis after overnight incubation in a moist chamber at 25 °C. The total mortality for each treatment was corrected according to Abbott’s formula based on mortality observed in the control [Citation30]. Corrected mortality (%) = (1 – number in fungal treatment/number in control treatment) × 100. All experiments were performed with two independent treatments, each of which was replicated three times, using 20 second-instar nymphs per treatment. Data were analyzed with ANOVA (p < 0.05) using SPSS (version 23; SPSS Inc.), and if the F test produced a significant result, differences in each measurement were further examined using Duncan’s multiple range test (p < 0.05). The lethal concentration (LC) and lethal time (LT) values were grouped using Tukey’s post-hoc test, if variance analysis results were significant at the 95% confidence level using SPSS. LC and LT values were also examined with Probit analysis [Citation31] using SPSS. Regression analysis between the aphicidal activity and the LCs or LTs of I. javanica pf212 were determined, and Spearman correlation coefficients (R-values) and corresponding p-values were determined using SPSS.
The cumulative mortalities caused by application of spores of the pf185 isolate were dependent upon both spore concentration and days after treatment. Mortality gradually increased with time after treatment, and after 4 d an effect of spore concentration was observed (). More than 50% mortality was observed at 4 d with spores applied at concentrations of between 107 and 108 spores/mL (). Aphids treated with 106 spores/mL showed less than 50% mortality even after exposure for 6 d. For second-instar nymphs sprayed with 0.02% Tween 80 as a control, the average mortality after 6 d was 10%. In contrast, more than 90% mortality was observed after 6 d among aphids treated with 109 spores/mL ().
Figure 3. Growth inhibition of four plant pathogens caused by Isaria javanica pf185 and pf212 when grown in dual cultures on potato dextrose agar. Fo, Fusarium oxysporum; Cg, Colletotrichum gloeosporioides; Pc, Phytophthora capsici; and Rs, Rhizoctonia solani. (A) Error bars represent the mean standard error of 10 total replicates per treatment in two independent experiments. P-values are provided for the significant differences in growth under different treatments (Student’s t-test, α < 0.05). (B) The images were obtained at 7 d after co-cultivation. The fungal pathogen was inoculated on the left-hand side of the plate, and I. javanica pf185 or pf212 on the right-hand side. The images are representative of two independent experiments with five plates for each experiment.
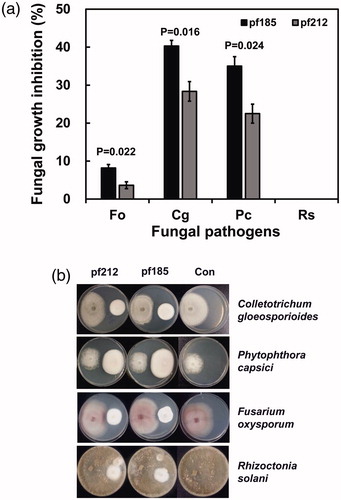
Figure 4. The percentage cumulative mortality of second-instar green peach aphid nymphs, following treatment with spore suspensions of I. javanica pf185. The nymphs were treated with spores at concentrations of 106 (6), 107 (7), and 108 (8) spores/mL (8), as described in the text. Error bars represent the mean standard error of three replicates per treatment in two independent experiments. Data were analyzed using ANOVA (p < 0.05), and if the F test was significant, differences were further elucidated using Duncan’s multiple range test. Different letters indicate significant differences at p < 0.05 at each time point.
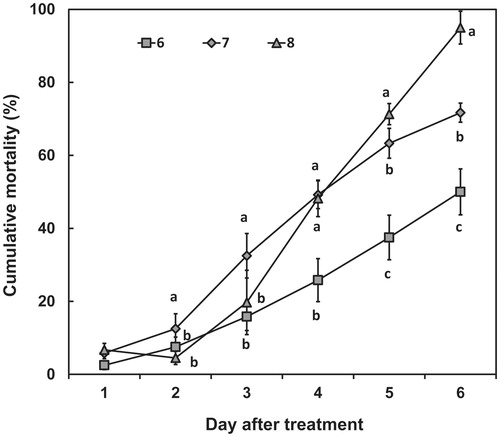
Calculations indicate that the LC50 for I. javanica was log10 = 7 at 4 d, and log10 = 6.5 at 5 d. For LC90, the titer was log10 = 9.5 (with 95% confidence limits of 8.8–10.9) at 4 d, and log10 = 8.6 (with 95% confidence limits of 8.1–9.6) at 5 d. The LT50 increased in treatments with lower spore concentrations (). The LT90 was 6.4 d (with 95% confidence limits of 6.0–7.3) for 108 spores/mL, 10.3 d (with 95% confidence limits of 8.3–24.0) for 107 spores/mL, and 13.6 d (with 95% confidence limits of 10.1–38.7) for 106 spores/mL ( and ). On the basis of these values, we estimated that the effective concentration (LC90) and time (LT90) for successful control of green peach aphids are 108 spores/mL and 6 d, respectively.
Table 2. Probit analysis of larval mortality for estimation of the median lethal concentrations of Isaria javanica pf185.
Table 3. Probit analysis of detached-leaf bioassays for estimation of changes in the lethal times of Isaria javanica pf185 at different spore concentrations for second-instar nymphs of the peach aphid.
The pattern of aphid mortality caused by isolates pf185 and pf212 is consistent with that previously observed for other Isaria isolates: over 70% mortality after 9–15 d exposure in brown citrus aphids treated with an isolate of I. fumosorosea, with 100% mortality occurring by day 22 [Citation32]. Our previous studies using I. javanica isolate pf04, showed high virulence against the sweet potato whitefly [Citation33,Citation34], with LT50 and LT90 values of 3.8 and 5 d following application of 109 spores/mL, respectively [Citation33]. These findings from the whitefly and aphid studies indicate that prolonged exposure and high spore densities are required for effective insect control. Thus, the medium for growth must be optimized. Previously, we found that I. javanica pf04, had optimal conidia formation when grown under solid state fermentation on a barley substrate supplemented with silkworm powder and moth larvae [Citation33]. The demonstration of antifungal activities in dual culture assays on PDA indicates that extracellular products are involved, and dibutyl succinate from pf185 was identified as a key metabolite for antifungal and aphicidal activity [Citation35]. These findings accordingly support the possibility of the production of effective cross-kingdom metabolites by other entomopathogens [Citation8,Citation9]. Consequently, because maximizing the dual biocontrol potential of strains is important, we are examining the effect of various growth conditions, including nutrition, temperature, and length of culture, utilizing in vitro assays for fungal growth antagonism.
In summary, we found that two isolates of the entomopathogenic fungus I. javanica showed dual biocontrol activities against different plant pathogenic fungi and the larvae and nymphs of moths and aphids, respectively. Isolate identification was based on a comparison of base sequences from two conserved fungal loci and the morphology of their spores and phialides with those of ex-type strains of the Isaria genus. Studies are currently in progress with the aim of optimizing growth conditions for the production of both high conidial concentrations and extracellular metabolites with antifungal activity. The selected I. javanica isolates could be used as novel biocontrol agents for dual biocontrol of insects and fungal diseases in red pepper.
Supplemental Material
Download MS Word (13.4 KB)Disclosure statement
No potential conflict of interest was reported by the authors.
Additional information
Funding
References
- Kim YC, Anderson AJ. Rhizosphere pseudomonads as probiotics improving plant health. Mol Plant Pathol. 2018;19;2349–2359.
- Anderson AJ, Kim YC. Biopesticides produced by plant-probiotic Pseudomonas chlororaphis isolates. Crop Prot. 2018;105:62–69.
- Jaber LR, Enkerli J. Fungal entomopathogens as endophytes: can they promote plant growth?. Biocontrol Sci Technol. 2017;27:28–41.
- Jaber LR, Ownley BH. Can we use entomopathogenic fungi as endophytes for dual biological control of insect pests and plant pathogens? Biol Control. 2018;116:36–45.
- Chen XH, Koumoutsi A, Scholz R, et al. Comparative analysis of the complete genome sequence of the plant growth–promoting bacterium Bacillus amyloliquefaciens FZB42. Nat Biotechnol. 2007;25:1007.
- Shafi J, Tian H, Ji M. Bacillus species as versatile weapons for plant pathogens: a review. Biotechnol Biotechnol Equip. 2017;31:446–459.
- de Faria MR, Wraight SP. Mycoinsecticides and mycoacaricides: A comprehensive list with worldwide coverage and international classification of formulation types. Biol Control. 2007;43:237–256.
- Wang X, Gong X, Li P, et al. Structural diversity and biological activities of cyclic depsipeptides from fungi. Molecules. 2018;23:169.
- Gibson DM, Donzelli BGG, Krasnoff SB, et al. Discovering the secondary metabolite potential encoded within entomopathogenic fungi. Nat Prod Rep. 2014;31:1287–1305.
- Guo YX, Liu QH, Ng TB, et al. Isarfelin, a peptide with antifungal and insecticidal activities from Isaria felina. Peptides. 2005;26:2384–2391.
- Ownley BH, Gwinn KD, Vega FE. Endophytic fungal entomopathogens with activity against plant pathogens: Ecology and evolution. In: Roy HE, Vega FE, Chandler D, Goettel MS, Pell J, Wajnberg E, editors. The Ecology of Fungal Entomopathogens. Dordrecht: Springer Netherlands; 2010. p. 113–128.
- Ownley BH, Griffin MR, Klingeman WE, et al. Beauveria bassiana: endophytic colonization and plant disease control. J Invertebr Pathol. 2008;98:267–270.
- Wang Q, Xu L. Beauvericin, a bioactive compound produced by fungi: a short review. Molecules. 2012;17:2367.
- Kavková M, Čurn V. Paecilomyces fumosoroseus (Deuteromycotina: Hyphomycetes) as a potential mycoparasite on Sphaerotheca fuliginea (Ascomycotina: Erysiphales). Mycopathologia. 2005;159:53–63.
- Szentiványi O, Varga K, Wyand R, et al. Paecilomyces farinosus destroys powdery mildew colonies in detached leaf cultures but not on whole plants. Eur J Plant Pathol. 2006;115:351–356.
- Huang Z, Hao Y, Gao T, et al. The Ifchit1 chitinase gene acts as a critical virulence factor in the insect pathogenic fungus Isaria fumosorosea. Appl Microbiol Biotechnol. 2016;100:5491–5503.
- Jaber LR, Salem NM. Endophytic colonisation of squash by the fungal entomopathogen Beauveria bassiana (Ascomycota: Hypocreales) for managing Zucchini yellow mosaic virus in cucurbits. Biocontrol Sci Technol. 2014;24:1096–1109.
- Sasan RK, Bidochka MJ . The insect-pathogenic fungus Metarhizium robertsii (Clavicipitaceae) is also an endophyte that stimulates plant root development . Am J Bot. 2012;99:101–107.
- Sasan RK, Bidochka MJ. Antagonism of the endophytic insect pathogenic fungus Metarhizium robertsii against the bean plant pathogen Fusarium solani f. sp. phaseoli. Can J Plant Pathol. 2013;35:288–293.
- Ramarao N, Nielsen-Leroux C, Lereclus D. The insect Galleria mellonella as a powerful infection model to investigate bacterial pathogenesis. J Vis Exp. 2012;4392.
- Kim KD, Oh BJ, Yang J. Differential Interactions of a Colletotrichum gloeosporioides isolate with green and red pepper fruits. Phytoparasitica. 1999;27:97–106.
- Glass NL, Donaldson GC. Development of primer sets designed for use with the PCR to amplify conserved genes from filamentous ascomycetes. Appl Environ Microbiol. 1995;61:1323–1330.
- White TJ, Bruns TL,S, Taylor JW. Amplification and direct sequencing of fungal ribosomal RNA genes for phylogenetics. Innis MA, Gelfand DH, Sninsky JJ, White TJ, editors. San Diego: Academic Press; 1990, p. 315–322.
- Hall TA. BioEdit: a user-friendly biological sequence alignment editor and analysis program for Windows 95/98/NT. Nucleic Acids Symp Ser (Oxf). 1999;41:95–98.
- Kumar S, Stecher G, Tamura K. MEGA7: Molecular evolutionary Genetics Analysis Version 7.0 for Bigger Datasets. Mol Biol Evol. 2016;33:1870–1874.
- D'Alessandro CP, Jones LR, Humber RA, et al. Characterization and phylogeny of Isaria spp. strains (Ascomycota: Hypocreales) using ITS1‐5.8S‐ITS2 and elongation factor 1‐alpha sequences. J Basic Microbiol. 2014;54:S21–S31.
- Gallou A, Serna-Domínguez MG, Berlanga-Padilla AM, et al. Species clarification of Isaria isolates used as biocontrol agents against Diaphorina citri (Hemiptera: Liviidae) in Mexico. Fungal Biol. 2016;120:414–423.
- Cabanillas HE, de León JH, Humber RA, et al. Isaria poprawskii sp. nov. (Hypocreales: Cordycipitaceae), a new entomopathogenic fungus from Texas affecting sweet potato whitefly. Mycoscience. 2013;54:158–169.
- Shamly V, Kali A, Srirangaraj S, et al. Comparison of microscopic morphology of fungi using lactophenol cotton blue (LPCB), iodine glycerol and congo red formaldehyde staining. J Clin Diagn Res. 2014;8:DL01–DDL2.
- Abbott WS. A method of computing the effectiveness of an insecticide. J Econ Entomol. 1925;18:265–267.
- Russell RM, Robertson JL. Programming probit analysis. Bull Entomol Soc Am. 1979;25:191.
- Hunter WB, Avery PB, Pick D, et al. Broad spectrum potential of Isaria fumosorosea against insect pests of citrus. Fla Entomol. 2011;94:1051.
- Xie L, Han JH, Kim JJ, et al. Effects of culture conditions on conidial production of the sweet potato whitefly pathogenic fungus Isaria javanica. Mycoscience. 2016;57:64–70.
- Zhu H, Kim JJ. Susceptibility of the tobacco whitefly, Bemisia tabaci (Hemiptera: Aleyrodidae) biotype Q to entomopathogenic fungi. Biocontrol Sci Techn. 2011;21:1471–1483.
- Lee Y-S, Han JH, Kang BR, et al. Dibutyl succinate, produced by an insect-pathogenic fungus, Isaria javanica pf185, is a metabolite that controls of aphids and a fungal disease, anthracnose. Pest Manag Sci. 2018. doi:10.1002/ps.5191.