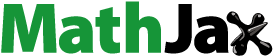
Abstract
Eritadenine from shiitake mushroom is a secondary metabolite with hypocholesterolemic, hypotensive and antiparasitic properties, thus promising for pharmaceutical and agricultural applications. Eritadenine is obtained from submerged mycelial cultures of shiitake, but the actual yields remain unsatisfactory to explore potential applications or industrial-scale production. In this study, green and blue LED lights were tested to increase yields of eritadenine in submerged cultures of shiitake. Notably, blue LEDs increased yields by 13–14 times, reaching 165.7 mg/L, compared to darkness (11.2 mg/L) and green light (12.1 mg/L) (p < 0.05, Tukey test). Nitrogen sources yeast extract (YE) and peptone (at 2 g/L) increased eritadenine production. YE promoted 22.6 mg/L, while peptone 18.3 mg/L. The recovery of eritadenine was evaluated using amberlite and activated charcoal (AC) adsorption isotherms. AC demonstrated the highest adsorption rate, with 75 mg of eritadenine per gram of AC, according to the Freundlich isotherm. The desorption rate reached 93.95% at pH 10. The extract obtained from submerged cultures had eritadenine content of 63.31%, corresponding to 87.86% of recovery, according to HPLC analysis. Furthermore, the novel bioherbicidal potential of eritadenine was tested on in vitro Chrysanthemum morifolium plants. The cultures extract containing eritadenine had a detrimental impact on plant development, generating mortality of 100% at 3%, 0.5%, and 0.25%. Moreover, pure eritadenine exhibited a phytotoxic effect similar than glyphosate on leaves, stems and roots. These findings highlight the significant bioherbicidal properties of eritadenine. Further studies are needed to understand the biosynthetic pathway of eritadenine and its bioherbicidal properties on weeds and illicit crops.
1. Introduction
Eritadenine, a purine alkaloid-like secondary metabolite, is primarily found in shiitake mushroom and in smaller quantities in other higher fungi (basidiomycetes) such as Flammulina, Hericium, and Agaricus [Citation1]. This compound exhibits several interesting biological activities, including: (i) the reduction of blood cholesterol and the improvement of dyslipidemia by decreasing phosphatidylcholine content and increasing phosphatidylethanolamine content in the liver [Citation2]. (ii) Inhibition of the blood pressure-regulating angiotensin hormone [Citation1], and (iii) inhibition of in vitro growth of the human parasite protozoa Cryptosporidium sp. [Citation3], achieved by blocking the S-adenosyl-l-homocysteine hydrolase (SAHH) protein, which is essential for methylation and regulation reactions in cells [Citation4]. Eritadenine production through submerged cultivation of shiitake mycelium has been performed by various authors, including us [Citation5–7]. Eritadenine yields have varied from 10.2 mg/L [Citation5], 25 mg/L [Citation6] and 88 mg/L [Citation8]. Nevertheless, these yields continue to be unsatisfactory for further exploration of potential applications or for scaling up production to an industrial level.
Nitrogen sources exert an influence on eritadenine production. They significantly impact the growth and biosynthesis of secondary metabolites during the submerged cultivation of filamentous fungi [Citation9,Citation10]. The supplementation of shiitake with a cereal extract containing 34% crude protein has been shown to promote superior yields of eritadenine, reaching up to 25 mg/L [Citation6]. Furthermore, it is noteworthy that peptone and yeast extract (YE) have a positive influence on lovastatin production when used with Aspergillus terreus [Citation9]. Similar effects of nitrogenous nutrients have been observed in the production of secondary metabolites chaetominine in Aspergillus sp. [Citation11], and cephalosporin in Acremonium [Citation12]. Nitrogen plays a key role in gene regulation, both in primary and secondary metabolism [Citation13]. It can induce the expression of gene clusters associated with secondary metabolism through regulatory proteins, a phenomenon referred to as “nitrogen metabolite repression” [Citation14]. These findings collectively suggest that the supplementation of nitrogen sources by this biotechnological process is a feasible alternative to producing or increasing the metabolite eritadenine.
Light has demonstrated effects on fungal metabolism [Citation15], acting as a regulator of gene expression to produce secondary metabolites [Citation16]. Various light receptors have been identified in mushrooms, including shiitake. For instance, the Le.phrA gene encodes a protein with photoreceptor domains sensitive to blue light [Citation17]. An ontology analysis of the transcriptome in shiitake grown under blue light revealed that of the 221 upregulated genes, 178 are related to biological processes such as the biosynthesis and metabolism of primary and secondary metabolites [Citation18]. In Monascus, the white collar (WC) photoreceptors with DNA binding domains are known to be homologous to the PoWC-1 and PoWC-2 genes of Pleurotus ostreatus [Citation19]. Under blue light conditions, Monascus is stimulated to produce higher yields of citrinin [Citation20], ankaflavin [Citation16] and monascin [Citation21]. Similarly, green light has been shown to enhance pigment production in Chaetomium cupreum [Citation22] and promote higher biomass yields in shiitake [Citation23]. However, it’s worth noting that the influence of LED light on eritadenine and biomass production in submerged cultures of shiitake remains to be investigated.
Recovery of eritadenine remains a challenging task. Eritadenine has been extracted from shiitake fruiting bodies using adsorption techniques with amberlite resins and supercritical gas extraction with CO2 [Citation24,Citation25]. However, these approaches have not been used to recover the metabolite from submerged fermentations of shiitake. Furthermore, the utilization of activated charcoal (AC) has demonstrated to be an effective method for extracting various metabolites, such as clavulanic and fumaric acids, from microbial fermentations [Citation26,Citation27]. Additionally, it is necessary to evaluate adsorption isotherms to understand the adsorption mechanism of eritadenine on a certain sorbent [Citation28]. Remarkably, the adsorption of eritadenine using AC and the corresponding isotherms have yet to be reported. This information gap represents a significant impediment to obtaining this metabolite in substantial quantities.
Herbicides play a significant role in the agricultural sector, as crops often demand substantial quantities of them to manage unwanted weeds. It is projected that by 2025, the global demand for herbicides will reach 150,000 million pounds, with a total estimated value of 2,000 million dollars [Citation29]. Unfortunately, in many instances, weed control involves the use of herbicides that are harmful to people and the environment, representing high economic burdens [Citation29]. The prolonged use of chemical herbicides can lead to weed resistance and tolerance, contributing to health concerns like poisoning and cancer [Citation30]. Fungal-based bioherbicides have emerged as a sustainable and an environmentally friendly alternative [Citation31]. Phytotoxic effects derived from secondary metabolites produced in submerged cultures are reported by fungi including Aspergillus niger [Citation32], Fusarium avenaceum [Citation33], and Fusarium oxysporum [Citation34]. Some of these substances exhibit bioherbicidal properties, causing defoliation of plants and other adverse effects such as germination inhibition and stem growth inhibition [Citation32]. For instance, in seedlings of the weed Bidens pilosa, Aspergillus niger inhibits organs by up to 97% in roots and 85% in plumules [Citation30]. Additionally, the metabolite phomentrioloxin derived from the fungus Phomopsis sp. applied at 2% concentration on leaf punctures, causes necrosis and chlorophyll degradation [Citation35]. However, the extraction of substances with bioherbicide properties from submerged cultures of shiitake, including eritadenine, has not yet been explored as a potential bioherbicide. In this study, we aimed to assess the effect of blue and green LED lights, as well as nitrogen sources such as yeast extract (YE) and peptone, at various concentrations on the enhancement of eritadenine production in submerged cultures of shiitake mycelium. Additionally, the extraction of eritadenine from fermented broths using activated charcoal (AC) and amberlite adsorption, with adjustments to the Freundlich and Langmuir isotherms models was mounted. Finally, we sought to determine the in vitro herbicidal activity of the cultures extract containing eritadenine on chrysanthemum morifolium plants cultured in vitro.
2. Materials and methods
2.1. Study area
This research was conducted at the Mycology Laboratory of Universidad Católica de Oriente, situated in Rionegro, Antioquia, Colombia (N6 9 206.4; W75 22 0.024); and the Chemistry Laboratory of Centro de la Ciencia y la Investigación Farmacéutica (CECIF), located in Sabaneta, Antioquia, Colombia (N6 9 29.517; W75 36 16.75).
2.2. Strain and propagation
The shiitake isolate LEUCO1, which is closely related to strain CR62 based on ITS genes conducted in a previous study [Citation7], was utilized. Under sterile conditions, LEUCO1 was inoculated into Petri dishes with malt extract agar (MEA) at a concentration of 48 g/L and then incubated for twelve days at 24 °C until obtaining full colonization of the Petri dish. All reactants were purchased from Merck, unless another provider is mentioned.
2.3. Eritadenine production using nitrogen sources and LED light
Six 0.5 cm diameter shiitake mycelium disks from agar cultures were inoculated into 250 mL Erlenmeyers containing 100 mL of basal broth composed of malt extract at 20 g/L, supplemented with nitrogen sources such as yeast extract (YE) or peptone at concentrations of 1 and 2 g/L. Erlenmeyers flasks were incubated in an orbital shaker (Actum, Colombia) at 100 rpm and 24 °C for 20 days. In another experiment, erlenmeyers flasks with the same basal broth, supplemented with 2% peptone, were used to conduct fermentations under blue LED light, green LED light or darkness.
The orbital shaker consisted of a three-level apparatus with green and blue LED lights at each level featuring two pairs of 15 W LEDs of the same color, resulting in a total of 8 LED lights per level. When the LEDs of the same color were activated, they formed a lit square of 625 cm2, positioned 40 cm above the erlenmeyers flasks. The green LEDs emitted light at a wavelength of 504 nm, while the blue ones emitted light at 482 nm. The flasks were illuminated with the corresponding color of light for 1 h per day with a 24 - hour interval, providing an intensity of 269 Lux during the 20 days of the fermentation. The shaker was isolated from natural light with cardboard to ensure that only the intended light conditions were provided to the cultures, maintaining the darkness condition in accordance with the light program. Fermentations not conducted under light conditions were exposed to 12 h of natural room light (107 lux measured at 12 noon) and 12 h of darkness. After the fermentations finished, eritadenine, biomass, and the final pH were measured.
2.4. The separation of eritadenine through adsorption and desorption
From the previously blue LED-irradiated fermentations, eritadenine adsorption was evaluated using two common adsorbents, (1) Amberlite IR120 Na+ (Sigma Aldrich, particle of 0.7 mm average) and (2) AC, DARCO, 100 mesh (Merck, particle of 0.149 mm). Adsorption isotherms were carried out in accordance with the methodology outlined by Ayub et al. [Citation28].
Five different dilutions were generated from the obtained fermentations by arbitrary dilution with deionized water, resulting in six different eritadenine concentrations, including the original concentration. The pH of these dilutions was adjusted to 3.5 using HCl. The concentrations in mg/L were as follows: 165.7, 150.4, 120.0, 100.4, 90.3, and 82.1 (determined via HPLC). A mixture of 30 mL of each dilution and 20 mg of adsorbent was placed in 50 mL erlenmeyers flasks, which were then incubated on a shaker at 100 rpm and 20 °C for 24 h to achieve the adsorption equilibrium. This process was done for each dilution, resulting in six adsorptions per adsorbent. The adsorbent was separated with Whatman filter paper (number four), and the eritadenine content in the liquid phase was quantified using HPLC. With the collected data, the adsorption capacity (qe) was calculated using Equationequation (1)(1)
(1) , and subsequently, isotherm models of Freundlich and Langmuir were determined using Equationequations (2)
(2)
(2) and Equation(3)
(3)
(3) , as outlined by Ayub et al. [Citation28].
(1)
(1)
Where qe is the adsorption capacity in mg of adsorbate/g adsorbent. Ci is the initial concentration of adsorbate, Ce is the concentration of adsorbate in equilibrium (remaining in solution after adsorption), W is the weight of the sorbent, and V is the volume of solution.
(2)
(2)
(3)
(3)
From the Langmuir Equationequation (2)(2)
(2) , qmax signifies the maximum adsorption capacity, expressed in mg/g, where Ce denotes the concentration at equilibrium and KL represents the Langmuir constant, necessary to calculate RL, which is the separation factor. Within the framework of the Freundlich Equationequation (3)
(3)
(3) , Kf stands as the empirical Freundlich constant, measured in mg/g, providing an indication of the approximate adsorption capacity. Simultaneously, 1/n is the heterogeneity factor.
2.5. Desorption assay
Eritadenine was obtained from submerged fermentations in erlenmeyers containing 100 mL of broth with base media plus peptone (2 g/L), with incubation at 24 °C, 100 rpm, blue LED 1 h/day, 20 days. Eritadenine was adsorbed by mixing 100 mL of this broth plus 1 g of AC, with incubation at 20 °C and 100 rpm for 24 h. This was performed with nine experimental units in 500 mL erlenmeyers. Then, AC was separated from the liquid, producing nine AC portions that were used to evaluate desorption divided into three treatments of different pH values (7, 8 and 10) [Citation24, Citation36]. Every AC portion was mixed with the corresponding treatment (20 mL) set at 100 rpm, 20 °C, 24 h. Then, the AC was filtered, and the eritadenine was measured in the filtered liquid by HPLC. With the initial and final concentrations of eritadenine, the recuperation percentage was calculated. The experiment conformed a complete randomized design with three repetitions. Recuperation percentages were analyzed by ANOVA.
2.6. Obtention of a cultures extract containing eritadenine
For this extraction, three (3) liters of fermented broth (obtained from the same batch as the previous experiment) were combined with 30 grams of AC in a 4 L flask. This mixture was incubated at 100 rpm at 20 °C for 24 h. The AC was subsequently filtered, followed by another round of incubation at 20 °C for 24 h at 100 rpm after adding 300 mL of ammonia to water to reach a pH of 10. The AC was then filtered once more. The liquid phase was subjected to rotoevaporation (Heidolf, laborta 4002 WB-G1) under conditions of 66–67 mb and 40 °C. Eritadenine content in the residue was quantified using HPLC. This extraction process was conducted three times to ensure repeatability and accuracy.
2.7. Quantitation of eritadenine using HPLC
The quantification method employed in this study followed the procedures outlined in our previous investigation [Citation8]. An Agillent Technologies 1200 Series apparatus, equipped with a C18 column, was used for this purpose. The mobile phase consisted of acetonitrile with a gradient starting at 2% and increasing to 60% during the initial 10 min. Additionally, trifluoroacetic acid (TFA) at a concentration of 0.1% was introduced, transitioning the acetonitrile composition from 60% back to 2% over the course of one minute, spanning from the 10th to the 11th minute. The analysis was conducted at a constant temperature of 23 °C, with detection at 260 nm. Quantification was carried out by means of a calibration curve employing standard-grade eritadenine sourced from Santa Cruz Biotechnology Inc., as a reference.
2.8. Plant propagation and bioherbicidal activity evaluation of the cultures extract containing eritadenine
Chrysanthemum morifolium plants (cultivar Maisy) were in vitro micropropagated using internodal buds. These buds were disinfected through sequential washing with 10% iodine soap, 70% ethanol, and a subsequent immersion in sodium hypochlorite (1%) for five minutes each. Under a laminar flow hood, 0.5 cm-long buds were planted in flasks of 120 mL with 10 mL of MS media [Citation37] and bacteriological agar (Phytotech). The plants were incubated in darkness for 10 days. Following this period, the conditions were adjusted to 18 °C, with a light cycle comprising 12 h of natural light (260 lux measured at 12 noon) and 12 h of darkness, lasting 35 days. This resulted in the proliferation of plants to be used in subsequent experiments.
Stems measuring 1 cm, featuring two nodes and devoid of leaves, were subsequently transferred into MS solid media enriched with the AIB hormone (Indolebutyric acid, Sigma) at a concentration of 1 mg/L. The goal was to induce root formation. In order to examine the bioherbicidal activity, four different concentrations of the cultures extract containing eritadenine were integrated into the media as distinct treatments (0.125%, 0.25%, 0.5%, and 3% in weight/volume), along with a control group, each consisting of 10 plants. The extract was microfiltered using syringe filters with pores of 0.2 µm (GVS, USA) and subsequently added to the sterilized media, which had been subjected to heat treatment at 121 °C, 15 PSI, for 20 min. The plants were then incubated for a period of 30 days at 18 °C under 12 h of light (260 lux) and 12 h of darkness. Measurements encompassing the length of stems, length of roots, number of leaves, and plant survival were meticulously recorded as response variables.
2.9. Evaluation of the bioherbicidal activity of pure eritadenine
To assess the bioherbicidal activity of pure eritadenine, we employed 30 mL test tubes containing 5 mL of the same solid medium MS supplemented with indole butyric acid (IBA) at the same concentrations utilized in the previous experiment. Two distinct treatments were executed: The first treatment involved introducing standard pure eritadenine (Santa Cruz Biotechnology, Inc.) into the medium, at a concentration of 200 mg/L. In the second treatment, glyphosate (Roundup 747 from Monsanto) was added to the other media at the same concentration. Furthermore, a control group devoid of eritadenine or glyphosate was included in the experiment. In each treatment, 1 cm-long Chrysanthemum stems, possessing two nodes with the leaves removed, were planted. The plants were incubated for 30 days at 18 °C under 12 h of light (260 lux) and 12 h of darkness. The experiment consisted of a completely randomized design with five replications per treatment. Data concerning plant organs were recorded in the same manner as in the previous experiment with the cultures extract containing eritadenine. The explants were considered: (1) alive: if they were minimally green in and around the buds, with some browning possible in parts other than the buds; (2) dead: if they showed wilting of stems, leaves and roots; and (3) necrotic: minimally with buds, leaves or stems with brown or black spots.
3. Results and discusion
3.1. Eritadenine responds to LED lights
LED light exerts a notable influence on the production of eritadenine in shiitake mycelium, particularly with respect to the LEUCO1 strain. Blue LED light induces a substantial enhancement in eritadenine yield, surpassing that of darkness and green light by a factor of 13–14, yielding 165.7 mg/L, compared to 11.2 mg/L in darkness and 12.1 mg/L in the presence of green light, as illustrated in . This concentration represents nearly double the highest results reported in our previous study, which employed mycelial immobilization in calcium alginate [Citation8] that achieved a yield of 88.1 mg/L. Notably, blue LED light has also been observed to promote higher yields of secondary metabolites in Monascus. For instance, studies by Yang et al. [Citation20] reported increased production of citrinine; Chen et al. [Citation21] documented elevated monascin levels; and Wang et al. [Citation16] observed enhanced ankaflavin production under the influence of blue LED light. It is worth noting that blue light plays a critical role in fungi by regulating the expression of key enzyme genes within metabolic pathways and the expression of regulator genes, including long non - coding cRNAs (lncRNAs). Under blue light, the lncRNA AOANCR regulates the mraox gene involved in citrinine production in Monascus [Citation20].
Figure 1. Eritadenine yields (A), biomass (B) and final pH (C) with the LED lights used during submerged cultures. Values are averages of ± DS (n = 3). Different letters above the bars show statistical differences at p < 0.05, according to ANOVA and Tukey tests. Bl is blue light; Gr is green light.
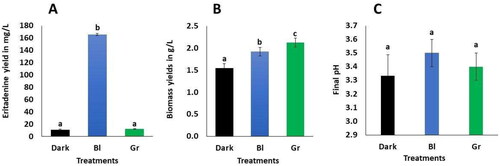
Furthermore, blue light is involved in the regulation of transcription factors, and fungi are known to possess photoreceptor proteins like white collar 1 and 2 (WC-1 and WC-2) that act as transcriptional factors, containing DNA-binding GATA zinc finger motifs. These motifs are homologous to the PoWC-1 and PoWC-2 genes identified in Pleurotus ostreatus [Citation19]. In shitake mushrooms, the putative Le.phrA gene encodes a blue light photoreceptor protein related to the formation of brown film by the tyrosinase and the formation of the fruiting body [Citation17, Citation38]. Similarly, transcriptomes have revealed in shiitake that blue light induces the expression of genes belonging to molecules involved in metabolic processes, such as protector pigments [Citation39], heat-shock proteins and secondary metabolites [Citation18]. On the other hand, in fungal plasma membranes, there are photoreceptors for green light known as opsins, although their specific functions remain unknown [Citation40]. Interestingly, green light enhances biomass production in shiitake; but it does not stimulate higher carotenoids yields in this species [Citation23]. Therefore, we hypothesize that LEUCO1 contains photoreceptors and regulators responsible for eritadenine biosynthesis, which are activated by blue light at a wavelength of 482 nm but not by green light at 504 nm. This study potentially marks the first report of blue LED light serving as a stimulating factor (elicitor) for the biosynthesis of the secondary metabolite eritadenine in shiitake mycelium.
3.2. Eritadenine yields with nitrogen sources
Nitrogen sources yielded superior results when compared to the control, which consisted solely of the base broth, as demonstrated in . Yeast extract (2 g/L) promoted up to twice the yield of eritadenine compared to the control, followed by peptone (2 g/L). Both nitrogen sources (1 g/L) showed lower and similar yields. A similar observation was made by Enman et al. [Citation6], who obtained 25 mg/L of eritadenine, using a broth supplemented with an aqueous cereal extract composed of a complex mixture of nutrients, including 34% crude protein. Nitrogen sources are important to filamentous fungi in submerged culture and, depending on the type and quantity, play a central role in gene regulation for secondary metabolites. This phenomenon is known as nitrogen metabolite repression, which explains the production of metabolites like fusaric acid in Fusarium [Citation13] or cephalosporin in Acremonium chrysogenum [Citation12]. Consequently, various fungal species have well-characterized AreA transcription factors, whose activation is dependent on the type and quantity of nitrogen sources, inducing the expression of certain clusters of secondary metabolites like polyketide synthase, leading to the production of a great variety of secondary metabolites like lovastatin or penicillin [Citation41]. The enhanced yields of eritadenine obtained with yeast extract as opposed to peptone can be partially attributed to the higher vitamin content in yeast extract, particularly vitamins A, D, E, C, B12, B3, and B1 [Citation42,Citation43].
Figure 2. Eritadenine yields, biomass and final pH in submerged cultures using various nitrogen sources. The values presented are the means with standard deviations (±DS) and are based on a sample size of n = 3. Different letters above the bars show statistical differences at p < 0.05; eritadenine and biomass (A and B) were evaluated by ANOVA and Tukey tests, while final pH (C) evaluated by Kruskal-Wallis test. YE1 is yeast extract at 1 g/L; YE2 is yeast extract at 2 g/L; Pep1 is peptone at 1 g/L; Pep2 is peptone at 2 g/L.
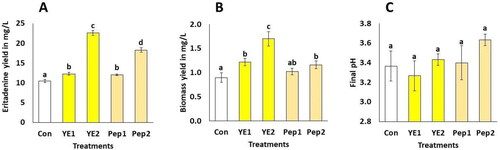
Similarly, with respect to shiitake mycelium, all amino acids except methionine have the potential to stimulate the production of the antioxidant metabolite ergothioneine [Citation44]. This leads us to infer that YE contains substances that promote the expression of genes related to eritadenine biosynthesis. In contrast, carbon sources (sucrose and starch) and salts (KH2PO4 and MgSO4) do not promote eritadenine production in shiitake. Multiple levels of these additives were tested and demonstrated yields similar to those of the control treatment (data not shown). These novel findings allow us to assert, for the first time, the crucial role of nitrogen sources in the biosynthesis of eritadenine in shiitake mushrooms.
3.3. Biomass yields under LED lights and nitrogen sources
The application of LED lights improved biomass production, the best yields were obtained under green light, followed by blue light (2.1 g/L and 1.9 g/L), as shown in . This finding is consistent with the observation of Glukhova et al. [Citation23], who reported a higher growth rate of shiitake in Petri dishes exposed to green and blue light compared to darkness. Their studies demonstrated an up to 1.6-fold increase in mycelial growth when exposed to green light for just one minute per day, compared to the darkness condition [Citation23]. In contrast, other fungi exhibited different responses to green and blue light. For instance, in Chaetomium cupreum, there was no discernible growth increase associated with any particular light color [Citation22]. Similarly, in Monascus, fusarium and Penicillium exposure to blue LEDs resulted in decreased growth [Citation20, Citation45]. This phenomen may be attributed to the repression of some primary metabolism genes [Citation20]. As Heintzen [Citation46] suggested, when fungi perceive light through the photosensitive chromophores such as flavin and retinal, various primary and secondary metabolic processes within the cell are activated. In the case of shiitake, blue light induces the expression of five genes responsible for laccase production. Lacasses are typical enzymes found in wood-decomposing fungi and play a crucial role in breaking down cellulose polysaccharides into monosaccharides. These monosaccharides serve as a carbon source to support fungal growth [Citation20]. Based on the aforementioned observations, we hypothesize that green and blue light stimuli induce primary metabolic mechanisms in the LEUCO1 isolate. It is plausible that under blue or green light, LEUCO1 actively expressed specific genes related to cell proliferation, thereby enhancing biomass yields. A study by Kim et al. [Citation18] on shiitake, for instance, found that transcriptome analysis under blue light revealed elevated expression levels in genes associated with primary metabolism processes, cell cycle regulation, lipid metabolism, carbohydrate transport, membrane assembly, and cytochrome p450. Furthermore, in Pleurotus ostreatus, blue light was identified to regulate genes essential for growth [Citation47]. On the other hand, nitrogen sources also affect biomass yields.
Results indicate that nitrogen sources also exert a discernible impact on biomass yields. The results clearly demonstrate that nitrogen sources exert a direct influence on biomass production. Specifically, YE at a concentration of 2 g/L yielded the highest biomass, followed by peptone at both 1 g/L and 2 g/L, with this two later being statistically similar between them and statistically similar to YE at 1 g/L. These findings slightly surpass the biomass reported by Ramesh et al. [Citation48], who achieved 1.3 g/L of Xilaria sp. biomass in a 40-day culture period. Other studies have also shown that optimal biomass production is achieved using YE rather than peptone in Pleurotus albidus [Citation10] and Trametes versicolor [Citation49]. This phenomenon can be attributed to the presence of organic nitrogen sources in YE and peptone, which are known to promote higher fungal biomass yields compared to inorganic sources along with increased enzymatic activity [Citation10]. Furthermore, ligninolytic enzymes exhibit heightened activity when shiitake fermentations are supplemented with soybean as opposed to ammonium nitrate, which primarily enhances growth [Citation50]. This pattern suggests that similar mechanisms may be at play in the case of the LEUCO1 isolate.
3.4. Adsorption of eritadenine and HPLC evaluation of the cultures extract containing eritadenine
Amberlite IR120 Na+ and AC showed capacity to adsorb eritadenine from shiitake submerged fermentations as depicted in . In both sorbents, the Langmuir and Freundlich models yielded values below 1 for RL (the separation factor) and 1/n (the adsorption capacity), indicating that these sorbents facilitate a favorable adsorption process, as suggested by Ragadhita and Nandiyanto [Citation51]. Furthemore, both models corroborate the superior adsorption capacity of AC compared to amberlite.
Figure 3. Isotherms of Langmuir and Freundlich adsorption models with their parameters for eritadenine adsorption from submerged cultures of shiitake with activated charcoal and amberlite IR120Na+. (A) Langmuir of AC; (B) Langmuir of IR120 Na+; (C) Freundlich of AC; and (D) Freundlich of amberlite IR120 Na+.
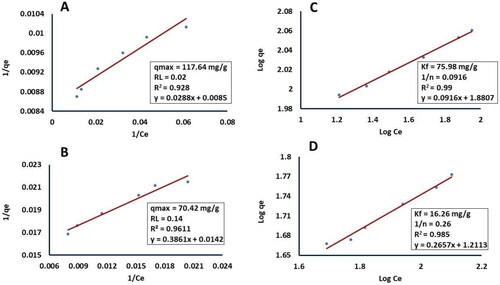
According to the Freundlich model, 75 mg of eritadenine can be adsorbed per gram of AC, while only 16.2 mg can be adsorbed per gram of Amberlite (). These results surpass those reported by Lopes and Colli [Citation26], who achieved an adsorption of 48.5 mg of clavulanic acid per gram of carbon adsorbent (CA) from Streptomyces fermentations. However, they are lower than the findings of Zhang et al. [Citation27], who attained an adsorption of 200 mg of fumaric acid per gram of CA from Rhizopus fungi fermentations.
The current scientific understanding acknowledges the well-established adsorption capacity of activated carbon (AC) for achieving the separation of various metabolites from complex mixtures. This includes compounds such as polyphenols [Citation52], organic acids [Citation26,Citation27], and polyalcohols [Citation53]. This remarkable capability arises from AC's nature as a porous, amorphous solid characterized by layers of hydrophobic graphite with a significant surface area. Additionally, AC features heterogeneous hydrophilic functional groups that, importantly, do not induce chemical alterations in the adsorbates [Citation52]. Moreover, the AC type employed in this particular investigation, the DARCO variant, contains hydrophilic acid groups, including carboxyl groups, at a concentration of 0.9 mmol/m2 [Citation54]. These functional groups are likely to play a role in the adsorption of eritadenine.
Amberlite IR120 Na+ on the other hand, is a resin consisting of spherical particles within a styrene-divinylbenzene matrix, possessing sulfonic acid functional groups along with interchangeable Na+ cations [Citation55,Citation56]. Souderjani et al. [Citation56] reported that the particles of amberlite IR120 Na+ exhibit an average diameter of 0.7 mm. In contrast, Darco AC comprises particles with a diameter of 0.14 mm and a surface area measuring 478 m2 per g [Citation54,Citation57]. Consequently, the larger surface area of AC in comparison to that of amberlite elucidates the observed higher absorption capacity, substantiated by its great affinity for eritadenine, as indicated by the 1/n parameter of the Freundslich isotherm.
Given that the eritadenine molecule features NH2+ and COO- ionizable groups [Citation5], the utilization of adsorbents with charged groups such as AC or amberlite for the recovery of eritadenine is well justified. Possibly, this metabolite is adsorbed by electrostatic forces on both sorbents [Citation36,Citation52].
However, the existing literature has primarily showcased the application of amberlite IR120 H+ and amberlite IRA400 from fruiting bodies without conducting a comprehensive analysis of adsorption capacity using isotherms [Citation24]. Of the two models considered, the Freundlich model elucidates more effectively the adsorption on AC (R2 = 0.99) and amberlite (R2 = 0.98), when compared to the Langmuir model (R2 = 0.92 and 0.96, respectively), as shown in . In accordance with Muhamad et al. [Citation58], the adsorption of eritadenine on both adsorbents takes place on a heterogeneous surface with a non-uniform distribution of energy, involving multiple layers of molecules on the surface of these sorbents. Additionally, the parameter 1/n exhibits low values of 0.09 with AC and 0.26 with amberlite. As noted by Penedo et al. [Citation59] and García-Pérez et al. [Citation52], this suggests a robust interaction between both sorbents and eritadenine, with a higher capacity for adsorption denoted by the Freundlich isotherm, particularly with AC. This implies a greater affinity and capacity for adsorbing the metabolite.
The results showed the most effective eritadenine desorption from AC occurred at pH 10 (93.95%), followed by pH 8 (47.47%) and pH 7 (18.46%). These findings suggest a direct relationship between desorption and higher alkaline pH values. Özkaya [Citation60] and Mojoudi et al. [Citation36] reported lower desorption values (ranging between 50 and 60%) for phenol when using AC with 0.1 M NaOH (pH = 13). These authors postulated that desorption takes place due to the deprotonation of the AC under alkaline conditions, leading to increased electrostatic repulsion between the sorbent and the adsorbates, such as eritadenine. It appears that at pH 10, the acid groups of the DARCO AC release protons, resulting in the liberation of eritadenine. Nevertheless, the optimal pH value for eritadenine desorption from AC was not determined in this research.
On the other hand, HPLC analysis carried out on the cultures extract containing eritadenine recovered using AC showed a 63.31% average content of eritadenine as shown in . This equates to an impressive recovery rate of 87.86% from the submerged fermentations of shiitake LEUCO1. From submerged cultures, similar recuperation has been reported for citric acid and lactic acid with Yarrowia and Lactobacillus respectively [Citation61,Citation62]. The first metabolite needed an electrodialysis concentration plus adsorption with CA, desorption at pH 12, and elution with methanol. While, in the second case, the metabolite was obtained by adsorption with IRA-400 resin plus desorption with NaOH 1 N (pH = 14). Therefore, the results obtained in the eritadenine recovery using AC are highly promising, and this may very well be the inaugural report of eritadenine adsorption using AC and isotherms. This method employs more commonly available and less hazardous reagents than other protocols, entails fewer steps, operates at milder pH values, and holds potential utility in future endeavors focused on scaling up the extraction of eritadenine from submerged fungal cultures. However, more research about optimal eritadenine pH desorption might be performed.
3.5. Phytotoxic effects of the cultures extract containing eritadenine
The cultures extract containing eritadenine obtained from AC was assessed on C. morifolium plants, although this experiment was not originally planned. Initially, the extract was tested against the TSWV virus, which had been cultivated on C. morifolium plants (data not shown), but it yielded unsatisfactory results. To our surprise, a potential bioherbicide effect on C. morifolium plants became evident. Consequently, various concentrations of the extract were formulated to evaluate this effect. At the end of 30 days, the 3%, 0.5% and 0.25% extract treatments generated zero development of leaf, root and stem with 100% mortality. The 3% treatment presented necrosis (black color) in more than 50% of the areas, mainly in buds, surroundings and most of the stems. While, the 0.5 and 0.25% treatments, presented necrosis in less than 50% of the area, mainly in buds and their surroundings (). Furthermore, at a concentration of 0.125%, almost all the individuals showed a minimum production of stems and leaves, but no roots (). However, at 30 days, there was a 70% mortality rate, with buds, leaves and surroundings presenting a brown color. In few of those individuals, buds with necrosis (black color) were observed, while the remaining 30% continued alive, maintaining their green color on stems, buds and leaves. These outcomes demonstrated statistically significant differences from the control, which produced elongation of green stems, production of green leaves, and white/pale green roots (), without death nor necrosis, as showed in and Citation5.
Figure 4. Comparison of the phytotoxic effects of the cultures extract containing eritadenine and pure eritadenine on Chrysanthemum morifolium plants. (A, B) HPLC chromatograms of the standard substance and the extract. (C) Phytotoxic effect of the extract at 0.25% and its control (D). (E) Phytotoxic effect of glyphosate (Gli), pure eritadenine (Eri) and control (Con).
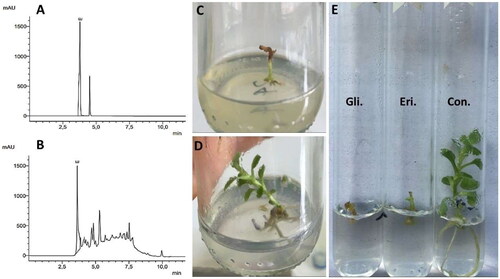
Figure 5. In vitro phytotoxic effects of the cultures extract containing eritadenine at 0.125% on Chrysanthemum morifolium. Plant values are averages of ± DS (n = 10). Different letters above the bars show statistical differences at p < 0.05, according to the U Mann-Whitney test. (A) length of stems. (B) number of leaves. (C) length of roots. The data for roots were not statistically analyzed; the average values are shown on the corresponding bars.
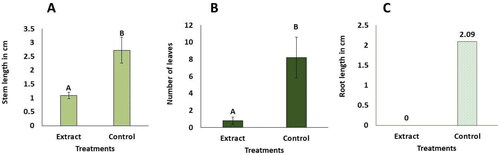
The results obtained clearly demonstrate a potent phytotoxic effect of the cultures extract containing eritadenine on the C. morifolium plants across all four concentrations that were assessed. These findings align with the bioherbicidal effect previously reported on seedlings of various herb species using organisms such as Aspergillus niger [Citation32], Fusarium avenaceum, Aureobacidium pullulans [Citation33], and Fusarium oxysporum [Citation34], using fermented broths applied to the seedlings. These authors mention that the phytotoxicity effect is due to the fact that these fungi produce toxins or secondary metabolites that are excreted into the fermented culture broth. However, it is noteworthy that the authors did not provide a comprehensive characterization of the chemical composition of the extract. Similarly, fungal herbicidal substances can induce various other detrimental effects, including the inhibition of germination and stem production [Citation32]. These substances can significantly impact root development, leading to inhibition levels of up to 97%, as observed in studies involving Trichoderma hamatum and Aspergillus niger [Citation30]. Given that the LEUCO1 extract comprises 63.3% eritadenine, there is the possibility of a synergistic interaction between this metabolite and the other unidentified compounds present in the extract. In support of this, the metabolite AMC (5-butyl-2-pyridine carboxylic acid) from Aspergillus has been reported to exhibit a synergistic effect, enhancing the action of antibiotics such as streptomycin, ciprofloxacin, and vancomycin against both Gram-positive and Gram-negative bacteria [Citation64].
However, it is essential to note that the extract did not demonstrate any inhibitory activity against the phytopathogens, including Ralstonia bacteria, the TSWV virus, and the Botrytis fungus (data not shown).
3.6. Phytotoxic effect of pure eritadenine
Results shown in indicate a pronounced phytotoxic effect of pure eritadenine. This metabolite significantly hindered the development of organs in C. morifolium plants, resulting in low mean values for the number of leaves, length of stems, and zero production of roots. Notably, these values were statistically similar to those observed in the glyphosate treatment group (). In contrast, both eritadenine and glyphosate treatments differed significantly from the control group, which exhibited the lowest mean values for organ development. Similar bioherbicidal activity has been reported in the basidiomycetous Hericium herinaceous, which is known to contain bioherbicidal substances called erinachromanes. These compounds have a more pronounced effect on the roots of lettuce plants. It is noteworthy that, as all erinachromanes possess a hydroxymethyl function, the authors suggest that this particular group may contribute to the bioherbicidal activity observed in all tested erinachromanes [Citation65,Citation66].
Figure 6. In vitro phytotoxic effects of pure eritadenine and glyphosate at 200 mg/L on Chrysanthemum morifolium. Values represent averages of ± DS (n = 5). Treatments marked with different letters indicate statistical significance at p < 0.05, according to Kruskal-Wallis and Dunn tests (Eri. Eritadenine; Gly. Glyphosate). (A) number of leaves; (B) length of stems; (C) length of roots. It’s important to note that the data related to root measurements were not subjected to statistical analysis; however, average values are presented on the respective bars.
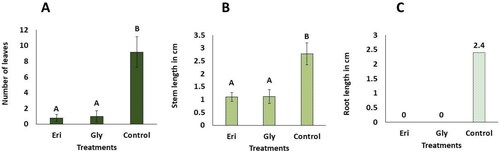
In spite of the inhibition of organs development in C. morifolium by both eritadenine and glyphosate. After 30 days, the explants were noted to be alive showing a green color in buds, stems and the few leaves formed, but only one individual of the glyphosate treatment that produced no organs at all was observed dead, presenting the starting of necrosis with a light brown color in the buds and stem. In contrast, the control individuals did not present death or necrosis, since they maintained a green color in stems and leaves along with a white/pale green color in roots (). These results can be attributed to the use of lower doses (200 mg/L = 0.02% w/v) of both substances in this experiment compared to the cultures extract containing eritadenine tested previously, which resulted in plant death or severe damage. For instance, the lowest dose of extract at 0.125% equated to 0.078% of pure eritadenine. Also, glyphosate is recommended to be applied at concentrations of no less than 1%, in accordance with label instructions. In contrast to the current findings, the metabolite phomentrioloxin from the fungus Phomopsis sp., when applied at a 2% concentration to needle punctures in leaves, caused necrosis after five days [Citation35]. However, it is worth noting that this concentration is higher than that used in the present work (200 mg/L = 0.02% w/v). In addition, in this experiment, no wounds were made on the in vitro planted shoots; rather, eritadenine was incorporated into the culture medium to be absorbed by the plants. Therefore, it is possible that necrosis and subsequent death could occur in C. morifolium plants by slightly increasing the concentration of eritadenine applied, as observed in the test with the extract at 0.25% ().
On the other hand, C. morifolium is an ornamental plant that was used here as a model organism to determine its susceptibility to the potential bioherbicide of eritadenine, without intending to promote the control of this important plant, since on the contrary, the study area (Colombia) is one of the largest producers worldwide [Citation67]. Therefore, this plant was chosen because (1) its wide availability in the study location, (2) the effect of eritadenine against its TSWV virus was being studied in this species propagated in vitro, (3) upon knowing its behavior on exposing it to agrochemicals such as herbicides, agronomic practices can be improved to enhance its yields [Citation68], and (4) because this plant belongs to the botanical family Asteraceae, that comprices over 25,000 herbaceous flowering species, including many of economic interest, as cultivated plants or invasive weeds, that share similar physiology and morphology characteristics [Citation69]. In particular, the Asteraceae, and the majority of weeds are broadleaf grasses, representing up to 86% of the weeds observed in wheat fields, so many herbicides are designed specifically to control these plants, by damaging most of these species in a nonselective manner [Citation63]. Therefore, since C. morifolium is a plant of this type, it is expected that its susceptibility to eritadenine can also be extrapolated to other broad-leaved plants, but this should be verified experimentally. Also, to test the phytotoxicity of the extract and the eritadenine, the in vitro micropropagation offers nutritional and environmental optimal conditions to C. morifolium, along with the exclusion of serious phytopathogens like white rust (Puccinia horiana) that are commonly present nearby the study zone and could have some influence on the results [Citation67, Citation70]; hence, this laboratory method instead of plants grown in soil was adopted.
We postulate that the bioherbicidal activity of eritadenine may involve the inhibition of the enzyme S-adenosyl-l-homocysteine hydrolase (SAHH) in C. morifolium. This process is well documented by Ctrnáctá et al. [Citation3] and Yang et al. [Citation4], who mention how the competitive inhibition of this enzyme leads to the accumulation of S-adenosylhomocysteine (SAH) and a subsequent reduction in the S-adenosylmethionine (SAM): SAH ratio within cells. Given that SAM serves as a donor substrate for methyl groups for SAM- dependent methylation reactions involving various biological molecules, including DNA, this inhibition ultimately disrupts cell proliferation [Citation71].
Also, in plants like Arabidopsis, SAHH plays an essential role in growth. Partial loss of this enzyme is associated with slow growth, reduced size, diminished fertility and shorter primary roots, often characterized by limited or absent root hairs [Citation72]. Then probably eritadenine interferes with methylation - dependent metabolic processes involved in the biosynthesis of cellular components crucial for cell multiplication in C. morifolium plants.
On the other hand, eritadenine features a reactive carboxyl group in its chemical structure [Citation6]. This group may potentially participate in reactions that impede the biosynthesis of essential molecules such as chlorophyll. In this sense, some derivatives of fomentrioloxin from the fungus Phomopsis sp. exert the phytotoxic effects by capitalizing on hydroxyl groups that react with chlorophyll, leading to its degradation [Citation35]. In Brassica and Avena plants, exposure to fermented broth from the Fusarium avenaceum fungus results in a significant reduction of a chlorophyll content to around 50% of the initial value after 144 h of contact [Citation33].
Weed control in crops represents an enormous industry, with an estimation that the annual costs related to management and production losses to the agricultural industry caused by weeds are USD 33 billion in the USA [Citation73], with the additional problem that there are reported more than 530 cases of herbicide resistance in 272 weed species, involving 168 herbicides in 72 countries [Citation74]. Hence, in order to help overcome the development of herbicide resistance, new systems with different modes of action are urgently required [Citation75]. This is a strong argument in favor of testing the biological effects of eritadenine not only on human uses but also on plants.
Based on the results derived from this study, eritadenine emerges as a promising bioherbicide with the potential to make a substantial contribution to global agriculture. It stands out as an eco-friendly candidate that poses no harm to human health or the environment. Consequently, further research is imperative to assess the bioherbicidal efficacy of eritadenine across a range of plant species, in pot assays, using carefully cultivated plants with healthy leaves treated with the spray method at different dosages. This exploration should extend to encompass even illicit crops and invasive plant species.
4. Conclusion
This research elucidates methods for obtaining significant quantities of eritadenine through submerged cultures of shiitake mycelium. Blue LED has been shown to induce a remarkable enhancement of eritadenine production, increasing it by up to 13–14 times when compared to conditions of darkness or exposure to green LED light. Moreover, the nitrogen source YE has been observed to increase eritadenine yields, highlighting its role as an essential nutrient for enhancing its production. Efficient separation of this metabolite can be achieved through the use of activated charcoal (AC), resulting in the extraction of eritadenine at concentrations exceeding 60%. The cultures extract containing eritadenine obtained in this study exhibited 100% in vitro phytotoxic activity, leading to the complete demise of C. morifolium plants at the tested concentrations of 3%, 0.5%, and 0.25%. At a concentration of 0.125%, a 70% mortality rate was observed. Similarly, pure eritadenine demonstrated potent phytotoxic effects on these plants, akin to those caused by the herbicide glyphosate, effectively inhibiting the development of plant organs (leaves, stems and roots). Notably, this research marks the first determination of bioherbicidal activity in eritadenine. Future research endeavors may focus on identifying the genes and biosynthetic pathways associated with the herbicidal activity of eritadenine. The data generated in this investigation holds promise for potential applications in the future commercial-scale production of eritadenine and the exploration of new uses of this metabolite.
Authors’ contributions
BD, FR, DC and WS conceived the project. BD mounted the experimental parts. FR, DC and WS advised the execution of the experiments. CS obtained the HPLC data. All authors wrote and edited the paper.
Acknowledgments
The authors want to be thankful to Yurani Chica Tobón and Esteban Loaiza from the Universidad Católica de Oriente and to Diego Rojas Vahos from the Science and Pharmaceutical Research Center CECIF because they supported in various ways the achievement of this project.
Disclosure statement
There is no potential conflict of interest between the authors.
Data availability statement
Data supporting the findings of this study are available from the corresponding author upon an e-mail request.
Additional information
Funding
References
- Afrin S, Rakib M, Kim B, et al. Eritadenine from edible mushrooms inhibits activity of angiotensin converting enzyme in vitro. J Agric Food Chem. 2016;64(11):2263–2268. doi:10.1021/acs.jafc.5b05869.
- Shimada Y, Morita T, Sugiyama K. Eritadenine-induced alterations of plasma lipoprotein lipid concentrations and phosphatidylcholine molecular species profile in rats fed cholesterol free and cholesterol enriched diets. Biosci Biotechnol Biochem. 2003;67(5):996–1006. doi:10.1271/bbb.67.996.
- Ctrnáctá V, Fritzler JM, Surinová M, et al. Efficacy of sadenosylhomocysteine hydrolase inhibitors, D-eritadenine and (S)-DHPA, against the growth of Cryptosporidium parvum in vitro. Exp Parasitol. 2010;126(2):113–116. doi:10.1016/j.exppara.2010.04.007.
- Yang H, Hwang I, Kim S, et al. Preventive effects of Lentinus edodes on homocysteinemia in mice. Exp Ther Med. 2013;6(2):465–468. doi:10.3892/etm.2013.1130.
- Enman J, Hodge D, Berglund K, et al. Production of the bioactive compound eritadenine by submerged cultivation of shiitake (Lentinus edodes) mycelia. J Agric Food Chem. 2008;56(8):2609–2612. doi:10.1021/jf800091a.
- Enman J, Hodge D, Berglund K, et al. Growth promotive conditions for enhanced eritadenine production during submerged cultivation of Lentinus edodes. J Chem Tech Biotech. 2012;87(7):903–907. doi:10.1002/jctb.3697.
- Duran-Rivera B, Rojas-Rodas F, Silva-López W, et al. Molecular identification of shiitake [Lentinula edodes Berk (Pegler)] and production of secondary metabolites with biotechnological potential. RB. 2020;5(3):1183–1188. doi:10.21931/RB/2020.05.03.3.
- Duran-Rivera B, Moreno-Suarez J, Rojas F, et al. Enhancement of eritadenine production using three carbon sources, immobilization and surfactants in submerged culture with shiitake mushroom (Lentinula edodes) (Berk.) Singer. Afr J Food Sci. 2018;12(12):374–382. doi:10.5897/AJFS2017.1654.
- Abd R, Lim E, Hasan H, et al. The investigation of media components for optimal metabolite production of Aspergillus terreus ATCC 20542. J Microbiol Methods. 2019;164:105672. doi:10.1016/j.mimet.2019.105672.
- Kirsch L, de Macedo A, Teixeira F. Production of mycelial biomass by the amazonian edible mushroom Pleurotus albidus. Braz J Microbiol. 2016;47(3):658–664. doi:10.1016/j.bjm.2016.04.007.
- Zhang Y, Jiao R, Lu Y, et al. Improvement of chaetominine production by tryptophan feeding and medium optimization in submerged fermentation of Aspergillus fumigatus CY018. Bioresour Bioprocess. 2016;3(1):45–49. 2- doi:10.1186/s40643-016-0117-5.
- Li J, Pan Y, Liu G. Disruption of the nitrogen regulatory gene AcareA in Acremonium chrysogenum leads to reduction of cephalosporin production and repression of nitrogen metabolism. Fungal Genet Biol. 2013;61:69–79. doi:10.1016/j.fgb.2013.10.006.
- Niehaus E, Janevska S, von Bargen K, et al. Apicidin F: characterization and genetic manipulation of a new secondary metabolite gene cluster in the rice pathogen Fusarium fujikuroi. PLoS One. 2014;9(7):e103336. doi:10.1371/journal.pone.0103336.
- López-Berges M, Rispall N, Prados-Rosales R, et al. A nitrogen response pathway regulates virulence functions in Fusarium oxysporum via the protein kinase tor and the bZIP protein MeaB. Plant Cell. 2010;22(7):2459–2475. doi:10.1105/tpc.110.075937.
- Chen D, Chen M, Wu S, et al. The molecular mechanisms of Monascus purpureus m9 responses to blue light based on the transcriptome analysis. Sci Rep. 2017;7(1):5537. doi:10.1038/s41598-017-05990-x.
- Wang C, Chen D, Chen M, et al. Stimulatory effects of blue light on the growth, monascin and ankaflavin production in Monascus. Biotechnol Lett. 2015;37(5):1043–1048. doi:10.1007/s10529-014-1763-3.
- Sano H, Narikiyo T, Kaneko S, et al. Sequence analysis and expression of a blue-light photoreceptor gene, Le.phrA from the basidiomycetous mushroom Lentinula edodes. Biosci Biotechnol Biochem. 2007;71(9):2206–2213. doi:10.1271/bbb.70170.
- Kim J, Kim D, Park Y, et al. Transcriptome analysis of the edible mushroom Lentinula edodes in response to blue light. PLoS One. 2020;15(3):e0230680. doi:10.1371/journal.pone.0230680.
- Qi Y, Sun X, Ma L, et al. Identification of two Pleurotus ostreatus blue light receptor genes (PoWC-1 and PoWC-2) and in vivo confirmation of complex PoWC-12 formation through yeast two hybrid system. Fungal Biol. 2020;124(1):8–14. doi:10.1016/j.funbio.2019.10.004.
- Yang H, Wang X, Li Z, et al. The effect of blue light on the production of citrinin in Monascus purpureus m9 by regulating the mraox gene through lncRNA AOANCR. Toxins 2019;11(9):536. doi:10.3390/toxins11090536.
- Chen D, Xue C, Chen M, et al. Effects of blue light on pigment biosynthesis of Monascus. J Microbiol. 2016;54(4):305–310. doi:10.1007/s12275-016-6011-1.
- Soumya S, Sreelatha G, Sharmila T. Light influences pigment, biomass and morphology in Chaetomium cupreum - ss02 - a photoresponse study. Int J Curr Microbiol Appl Sci. 2014;3(4):53–64.
- Glukhova L, Sokolyanskaya L, Plotnikov E, et al. Increased mycelial biomass production by Lentinula edodes intermittently illuminated by green light emitting diodes. Biotechnol Lett. 2014;36(11):2283–2289. doi:10.1007/s10529-014-1605-3.
- Enman J, Rova U, Berglund K. Quantification of the bioactive compound eritadenine in selected strains of shiitake mushroom (Lentinus edodes). J Agric Food Chem. 2007;55(4):1177–1180. doi:10.1021/jf062559+.
- Morales D, Piris A, Ruiz-Rodriguez A, et al. Extraction of bioactive compounds against cardiovascular diseases from Lentinula edodes using a sequential extraction method. Biotechnol Prog. 2018;34(3):746–755. doi:10.1002/btpr.2616.
- Lopes C, Colli B. Overproduction of clavulanic acid by extractive fermentation. Electron. J. Biotechnol. 2015;18(3):154–160. doi:10.1016/j.ejbt.2015.03.001.
- Zhang K, Zhang L, Yang S. Fumaric acid recovery and purification from fermentation broth by activated carbon adsorption followed with desorption by acetone. Ind Eng Chem Res. 2014;53(32):12802–12808. doi:10.1021/ie501559f.
- Ayub A, Raza Z, Majeed M, et al. Development of sustainable magnetic chitosan biosorbent beads for kinetic remediation of arsenic contaminated water. Int J Biol Macromol. 2020;163:603–617. doi:10.1016/j.ijbiomac.2020.06.287.
- Hossain M. Recent perspective of herbicide: review of demand and adoption in world agriculture. J Bangladesh Agric Univ. 2016;13(1):19–30. doi:10.3329/jbau.v13i1.28707.
- Daba A, Berecha G, Tadesse M, et al. Evaluation of the herbicidal potential of some fungal species against Bidens pilosa, the coffee farming weeds. Saudi J Biol Sci. 2021;28(11):6408–6416. doi:10.1016/j.sjbs.2021.07.011.
- Hasan M, Ahmad-Hamdani M, Rosli A, et al. Bioherbicides: an Eco-Friendly tool for sustainable weed management. Plants 2021;10(6):1212. doi:10.3390/plants10061212.
- Bashir U, Khan A, Javaid A. Herbicidal activity of Aspergillus niger metabolites against parthenium weed. Planta Daninha. 2018;v36:e018167123. doi:10.1590/s0100-83582018360100025.
- Cheng L, Zhu H, Wei Y, et al. Study on herbicidal potential of two fungi in Qinghai region. OALib. 2022;09(01):1–20. doi:10.4236/oalib.1108294.
- Singh A, Pandey A. Evaluation of herbicidal potential of selected mycoherbicidal strain against a noxious weed Cassia otusifolia L. ASAG. 2020;4:03–07. doi:10.31080/ASAG.2020.04.0907.
- Cimmino A, Andolfi A, Zonno M, et al. Phomentrioloxin, a fungal phytotoxin with potential herbicidal activity, and its derivatives: a structure-activity relationship study. J Agric Food Chem. 2013;61(40):9645–9649. doi:10.1021/jf4030618.
- Mojoudi N, Mirghaffari N, Soleimani M, et al. Phenol adsorption on high microporous activated carbons prepared from oily sludge: equilibrium, kinetic and thermodynamic studies. Sci Rep. 2019;9(1):19352. doi:10.1038/s41598-019-55794-4.
- Murashige T, Skoog F. A revised medium for rapid growth and bio assays with tobacco tissue cultures. Plant Physiol. 1962;15(3):473–497. doi:10.1111/j.1399-3054.1962.tb08052.x.
- Sano H, Kaneko S, Sakamoto Y, et al. The basidiomycetous mushroom Lentinula edodes white collar-2 homolog PHRB, a partner of putative blue-light photoreceptor PHRA, binds to a specific site in the promoter region of the L. edodes tyrosinase gene. Fungal Genet Biol. 2009;46(4):333–341. doi:10.1016/j.fgb.2009.01.001.
- Tang L, Tan Q, Bao D, et al. Comparative proteomic analysis of light induced mycelial brown film formation in Lentinula edodes. Biomed Res Int. 2016;2016:5837293–5837298. doi:10.1155/2016/5837293.
- Corrochano L. Light in the fungal world: from photoreception to gene transcription and Beyond. Annu Rev Genet. 2019;53(1):149–170. doi:10.1146/annurev-genet-120417-031415.
- Brakhage A. Regulation of fungal secondary metabolism. Nat Rev Microbiol. 2013;11(1):21–32. doi:10.1038/nrmicro2916.
- Izah S, Enaregha E, Epidi J. Vitamin content of Saccharomyces cerevisiae biomass cultured in cassava wastewater. MOJ Toxicol. 2019;5(1):42–45. doi:10.15406/mojt.2019.05.00151.
- Müller J, Beckers M, Mußmann N, et al. Elucidation of auxotrophic deficiencies of Bacillus pumilus DSM 18097 to develop a defined minimal medium. Microb Cell Fact. 2018;17(1):106. doi:10.1186/s12934-018-0956-1.
- Tepwong P, Giri A, Sasaki F, et al. Mycobial enhancement of ergothioneine by submerged cultivation of edible mushroom mycelia and its application as an antioxidative compound. Food Chem. 2012;131(1):247–258. doi:10.1016/j.foodchem.2011.08.070.
- Velmurugan P, Lee Y, Venil C, et al. Effect of light on growth, intracellular and extracellular pigment production by five pigment-producing filamentous fungi in synthetic medium. J Biosci Bioeng. 2010;109(4):346–350. doi:10.1016/j.jbiosc.2009.10.003.
- Heintzen C. Plant and fungal photopigments. WIREs Membr Transp Signal. 2012;1(4):411–432. doi:10.1002/wmts.36.
- Nakano Y, Fujii H, Kojima M. Identification of blue-light photoresponse genes in oyster mushroom mycelia. Biosci Biotechnol Biochem. 2010;74(10):2160–2165. doi:10.1271/bbb.100565.
- Ramesh V, Karunakaran C, Rajendran A. Optimization of submerged culture conditions for mycelial biomass production with enhanced antibacterial activity of the medicinal macro fungus Xylaria sp. Strain R006 against drug resistant bacterial pathogens. CREAM. 2014;4(1):88–98. doi:10.5943/cream/4/1/7.
- Adebayo-Tayo B, Emeka U. Influence of different nutrient sources on exopolysaccharide production and biomass yield by submerged culture of Trametes versicolor and Coprinus sp. AU J.T. 2011;15(2):63–69.
- Pedri L, Lozano M, Hermann K, et al. Influence of nitrogen sources on the enzymatic activity and grown by Lentinula edodes in biomass Eucalyptus benthamii. Braz J Biol. 2015;75(4):940–947. doi:10.1590/1519-6984.03214.
- Ragadhita R, Nandiyanto A. How to calculate adsorption isotherms of particles using two-parameter monolayer adsorption models and equations. Indonesian J Sci Technol. 2021;6(1):205–234. doi:10.17509/ijost.v6i1.32354.
- García-Pérez P, Losada-Barreiro S, Gallego P, et al. Adsorption of gallic acid, propyl gallate and polyphenols from Bryophyllum extracts on activated carbon. Sci Rep. 2019;9(1):14830. 15 doi:10.1038/s41598-019-51322-6.
- Soares C, Soares F. Purification of biotechnological xylitol from Candida tropicalis fermentation using activated carbon in fixed-bed adsorption columns with continuous feed. FBP. 2021;126:73–80. doi:10.1016/j.fbp.2020.12.013.
- García R, Peralta L, Segura C, et al. Study of the catalytic conversion and adsorption of abietic acid on activated carbon: effect of surface acidity. J Chil Chem Soc. 2016;61(4):3239–3245. doi:10.4067/S0717-97072016000400018.
- Özmetin C, Aydin Ö. A semi-empirical model for adsorption of magnesium ion from magnesium impurity-containing saturated boric acid solutions on amberlite IR-120 resin. Fresenius Environ. Bull. 2007;16:720–725.
- Souderjani E, Keshtkar A, Mousavian M. Application of response surface methodology for thorium (IV) removal using amberlite IR-120 and IRA-400: ion exchange equilibrium and kinetics. JPST. 2017;3(2):101–112. doi:10.22104/JPST.2017.2267.1088.
- Sabio E, Zamora-Polo F, González J, et al. Characterization under static and dynamic conditions of commercial activated carbons for their use in wastewater plants. Appl Surf Sci. 2006;252(17):6058–6063. doi:10.1016/j.apsusc.2005.11.026.
- Muhamad M, Abdullah S, Hasan H, et al. Adsorption isotherm and kinetic studies of pentachlorophenol removal from aqueous solution onto coconut shell-based granular activated carbon. J Environ Sci Technol. 2018;11:68–78. doi:10.3923/jest.2018.68.78.
- Penedo M, Manals E, Calzadilla F, et al. Adsorción de níquel y cobalto sobre carbón activado de cascarón de coco. Tecnología Química. 2015;35(1):110–124.
- Özkaya B. Adsorption and desorption of phenol on activated carbon and a comparison of isotherm models. J Hazard Mater. 2006;129(1–3):158–163. doi:10.1016/j.jhazmat.2005.08.025.
- Aurich A, Hofmann J, Oltrogge R, et al. Improved isolation of microbiologically produced (2R,3S)-isocitric acid by adsorption on activated carbon and recovery with methanol. Org Process Res Dev. 2017;21(6):866–870. doi:10.1021/acs.oprd.7b00090.
- Quintero J, Acosta A, Mejía C, et al. Purification of lactic acid obtained from a fermentative process of cassava syrup using ion exchange resins. Rev Fac Ingeniería. 2012;65(65):139–151. doi:10.17533/udea.redin.14225.
- Ayana B. Determination of efficacy of broad leaf herbicides on various weed floras in wheat field under rain fed production system. IJNRLS. 2022;9(3):1–9. doi:10.5281/zenodo.6510978.
- Mandal V, Ghosh N, Mitra P, et al. Production and characterization of a broad-spectrum antimicrobial 5-butyl-2-pyridine carboxylic acid from Aspergillus fumigatus nHF-01. Sci Rep. 2022;12(1):6006. doi:10.1038/s41598-022-09925-z.
- Wu J, Tokunaga T, Kondo M, et al. Erinaceolactones a to C, from the culture broth of Hericium erinaceus. J Nat Prod. 2015;78(1):155–158. doi:10.1021/np500623s.
- Wu J, Uchida K, Ridwan A, et al. Erinachromanes a and B and erinaphenol a from the culture broth of Hericium erinaceus. J Agric Food Chem. 2019;67(11):3134–3139. doi:10.1021/acs.jafc.8b06050.
- De Backer M, Alaei H, Bockstaele E, et al. Identification and characterization of pathotypes in Puccinia horiana, a rust pathogen of Chrysanthemum x morifolium. Eur J Plant Pathol. 2011;130(3):325–338. doi:10.1007/s10658-011-9756-8.
- Hensley D, Gibbons F. Tolerance of some garden flowers to selected preemergence herbicides. Trans Kansas Acad Sci. 1985;88(3/4):146–153. doi:10.2307/3627886.
- Araújo C, Morgado C, Gomes A, et al. Asteraceae family: a review of its allelopathic potential and the case of Acmella oleracea and Sphagneticola trilobata. Rodriguesia. 2021;72:e01622020. doi:10.1590/2175-7860202172137.
- Hollmann P, Lohbrunner G, Shamoun S, et al. Establishment and characterization of Rubus tissue culture systems for in vitro bioassays against phytotoxins from Rubus fungal pathogens. PCTOC. 2002;68(1):43–48. doi:10.1023/A:1012915118227.
- Li X, Huang L, Hong Y, et al. Co-silencing of tomato S-adenosylhomocysteine hydrolase genes confers increased immunity against Pseudomonas syringae pv. tomato DC3000 and enhanced tolerance to drought stress. Front Plant Sci. 2015;6:717. doi:10.3389/fpls.2015.00717.
- Wu X, Li F, Kolenovsky A, et al. A mutant deficient in S-adenosylhomocysteine hydrolase in arabidopsis shows defects in roothair development. Botany. 2009;87(6):571–584. doi:10.1139/B08-124.
- Chauhan B. Grand challenges in weed management. Front Agron. 2020;1:1–4. doi:10.3389/fagro.2019.00003.
- Heap I. Current Status of the International Herbicide-Resistant Weed Database. International Herbicide-Resistant Weed Database. 2024. Available online: http://www.weedscience.org. (accessed on 15 January 2024).
- Roberts J, Florentine S, Fernando W, et al. Achievements, developments and future challenges in the field of bioherbicides for weed control: a global review. Plants 2022;11(17):2242. doi:10.3390/plants11172242.