ABSTRACT
A mutational laccase gene MLcc1 was synthesized with modified codons, based on the codon bias of Pichia pastoris, in order to improve the thermal stability of Trametes versicolor laccase. The mutant gene was subcloned into the expression vector pGAPZαA and was transformed into P. pastoris strain X33. The mature protein consisted of 498 amino acids and contained a changed aspartate with proline at the 14th position of the native laccase (NLCC1). Under optimum conditions, the laccase activity reached 194.06 U/L after 54 h by high cell density fermentation in a 5 L fermenter. After incubation at pH 4.6 for 2 h, the recombinant enzyme MLCC1 retained 54.9% of its maximum activity, whereas the wild-type enzyme retained about 50% of its maximum activity. More than 20% of the residual activity was detected after up to 120 min at 90 °C for MLCC1, whereas less than 10% of the activity was retained for NLCC1.
Introduction
Laccases (benzenediol: oxygen oxidoreductase, EC 1.10.3.2) catalyze the monoelectronic oxidation of substrates at the expense of molecular oxygen. This green tool remains of great relevance in many processes of the biotechnology industries.[Citation1,Citation2] Fungal laccases have attracted considerable attention owing to their higher redox potential (+800 mV) compared to the redox potential of the plants' or bacteria's laccases. So far, more than 100 enzymes have been purified from various fungal species and characterized in terms of their biochemical and catalytic properties.[Citation3]
The occurrence of ligninolytic enzyme laccase in the Trametes versicolor genome makes T. versicolor an attractive fungus for miscellaneous, biotechnological and environmental applications.[Citation4] Many native fungi are slow growers and produce low amounts of laccase, which makes them difficult for studying and for large-scale application of their enzymes.[Citation5] Meanwhile, the thermostability of a protein is both of fundamental and industrial importance.[Citation6] Thermostable enzymes allow high process temperatures with associated higher reaction rates and less risk of microbial contamination.[Citation7,Citation8]
Enzymes of superior quality have been obtained not only by site-directed or random mutagenesis, such as improvement in biochemical and catalytic parameters, but also by ‘DNA shuffling’ methods, which yield a remarkable improvement in enzymes in a very short period of time.[Citation9] In addition, heterologous expression is an important way to achieve high-level production of laccase. Several fungal laccase genes have been cloned and heterologously expressed in various hosts.[Citation10–14] However, the laccase yields, reported in these studies, could not meet the increasing market demand.[Citation15]
Considering that the thermostable enzymes are typically tolerant to many other harsh conditions often required in industry,[Citation16] we created a thermotolerant laccase gene variant MLcc1 and expressed it in Pichia pastoris. To the best of our knowledge, this is the first study, where mutation of the N-terminal part of the laccase resulted in a variant with enhanced thermal stability.
Materials and methods
Plasmids and strains
T. versicolor As 5.48 was obtained from the Chinese Academy of Sciences. P. pastoris strain X33 (Invitrogen) was used for heterologous expression. pGAPZαA (Invitrogen) was used as an expression vector.
Synthesis and construction of the expression vectors, and expression of the mutated laccase gene in P. pastoris
Similarity test of laccases from T. versicolor, Trametes hirsuta, Ceriporiopsis rivulosa, Cerrena unicolor, Thermobifida fusca, Thermus thermophilus, Opuntia ficus-indica, Escherichia coli and Bacillus sp. LS04 was done using Vector NTI software. The Asp residue in position 14 was replaced with Pro in order to improve its thermal stability. The three-dimensional (3D) model of MLCC1 and non-mutated recombinant laccase (NLCC1) was constructed through SWISS-MODEL based on 3kw7B [Citation17]; protein structures were analysed with DeepView (Swiss-Pdb Viewer).
Based on the sequence of the Lcc1 gene of T. versicolor,[Citation18] we designed the gene without the 5'-end fragment coding for the N-terminal 22 amino acid signal sequence and added a XhoI and a NotI site at the 5'- and 3'-end, respectively. After codon optimization, we resynthesized the recombinant gene MLcc1 by using the polymerase chain reaction (PCR) based two-step DNA synthesis technique. The used primers were F1 (5'-ACCATCTCCAATGCGCACGTTTCTCCCGACGGC-3'), R1 (3'-TGGTAGAGGTTACGCGTGCAAAGAGGGCTGCCG-5'), F2 (5'-ACCATCTCCAATGCGCCCGTTTCTCCCGACGGC-3') and R2 (3'-TGGTAGAGGTTACGCGGGCAAAGAGGGCTGCCG-5'). The PCR reaction system consisted of 5 µL reaction buffer (10X), 1 µL (10 µmol/L) primer F1/F2, 1 µL (10 µmol/L) primer R1/R2, 4 µL (2.5 mmol/L each) dNTP mix, 2 µL (0.5 µg) templates, 1 µL Phusion® High-Fidelity DNA Polymerase (New England BioLabs) and 36 µL nuclease-free water. The PCR conditions were: one cycle of 95 °C for 2 min, 30 cycles of 95 °C for 30 s, 60 °C for 1 min and 68 °C for 5 min, followed by a final extension at 72 °C for 10 min. The MLcc1 gene was inserted into the P. pastoris expression vector pGAPZαA in frame with the α-factor secretion signal gene, under the control of the glyceraldehyde-3-phosphate dehydrogenase (GAP) promoter. The NLCC1 of T. versicolor was not codon optimized for P. pastoris.
The presence of the PCR products was verified by restriction enzyme digestion, agarose gel electrophoresis and sequencing. The digest reaction was performed with DoubleDigest Calculator by using 5 μL 10X buffer O, 10 μL plasmid, 5 μL XhoI (Fermentas), 2.5 μL NotI (Fermentas) and 27.5 μL double-distilled (dd) H2O. The PCR products were analysed on 1% agarose gel (Bio-Rad) and sequenced in Beijing BGI Biotechnology Ltd. (Beijing, P. R. China). Competent yeast cells were prepared according to the manufacturer's instructions (Invitrogen). The transformation of pGAPZαA/Lcc1 into P. pastoris X33 was performed with 10 μg/L linearized plasmid by using BspHI (according to the manufacturer's instructions, Invitrogen). Empty plasmid was transformed into P. pastoris X33 as a control strain. All P. pastoris transformations were carried out by electroporation (2.5 kV, 5.0 ms, Bio-Rad). The positive colonies were selected from yeast extract peptone dextrose (YPD) medium [1% (w/v) yeast extract, 2% (w/v) peptone, 2% (w/v) dextrose, 1.5% (w/v) agar] containing 200 μg/mL zeocin (Sigma) and 0.2 mmol/L 2,2-azinobis(3-ehtylbenzothiazolin-6-sulfnicAcid) (ABTS). Laccase-producing transformants (P14D mutants) were identified by the presence of a green zone around the colonies. The colonies with bigger green zones were transferred by using toothpicks into a 250 mL flask with 25 mL selective liquid media (YPD liquid medium containing 200 μg/mL zeocin). Samples were taken at different intervals for determination of the laccase activity. The transformant with the highest laccase-secreting ability was screened out.
Expression of laccase by P. pastoris in liquid culture
The P. pastoris transformants were grown overnight in yeast extract peptone glycerine (YPG) broth [1% (w/v) yeast extract, 2% (w/v) peptone, 3% (w/v) glycerine] as fermentation seed. Fifty millilitres of the culture were incubated in 250 mL flasks at 22 °C at 150 rpm. Samples were taken at different intervals for determination of the optical density at 600 nm (UV2550 UV–VIS spectrophotometer, Shimadzu).
Fed-batch culture and high cell density culture were carried out (60 h) in a 5 L fermenter and were started with a basic salt medium (BSM, 26.7 mL/L of 85% phosphoric acid, 0.93 g/L CaSO4·2H2O, 14.9 g/L MgSO4·7H2O, 18.2 g/L K2SO4, 4.13 g/L KOH, 40 g/L glycerol) by adding 4 mL/L Pichia trace metal (PTM1), as described by Invitrogen, containing 0.8 mmol/L CuSO4 and 30 g/L peptone. The feeding substrates containing 0.8 mmol/L CuSO4 and 300 g/L glucose were fed into the bioreactor by using a computer-controlled feeding system. The experiment was carried out using the dissolved oxygen (DO) feed-back feeding strategy (DO was maintained at 20%–60% by adjusting the fed amount). Culture liquid (5 mL) was centrifuged (Eppendorf) for 10 min at 8000 rpm at 4 °C. The supernatant was collected for determination of the laccase activity (U/L). The precipitate was dried at 65 °C for about 24 h, and weighed to get the dry weight (g/L) after cooling. All data shown are mean values of three replicates.
Enzyme purification
Specific activity (U/mg) as well as total enzyme activity (U) of the recombinant laccase expressed in P. pastoris were obtained by using ammonium sulphate precipitation, followed by centrifugation, filtration, dialyzation and elution through nickel-affinity chromatography. The yield (%) and purification (fold) of MLCC1 were calculated after each treatment.
Ammonium sulphate was added in the filtered fermentation liquor (treated by ultrafiltration membrane with 10 kDa molecular weight cut-off) to 80% saturation at 4 °C. After centrifugation (Eppendorf) at 12,000 rpm at 4 °C for 15 min, the precipitate was collected and dialysed with 50 mmol/L phosphate-buffered saline (PBS) buffer (pH 7.0). Then the supernatant was loaded onto a nickel-charged iminodiacetic acid column (GE Healthcare) pre-equilibrated with 50 mmol/L PBS buffer (pH 7.4), containing 0.5 mol/L NaCl, 100 g/L glycerol, 10 g/L Tween-80 and dd H2O at 4 °C. The column was first washed with PBS buffer containing 10 mmol/L imidazole and then was washed with the same buffer containing 150 mmol/L imidazole. Finally, the 6 × His-tagged enzyme was eluted with the buffer containing 500 mmol/L imidazole. The fractions were collected and dialysed at 4 °C overnight against 50 mmol/L PBS buffer. The fractions containing laccase activity were pooled and concentrated by lyophilization (Beijing Boyikang Ltd) subsequently.
Sodium dodecyl sulfate polyacrylamide gel electrophoresis (SDS-PAGE; 7.5% stacking gel, 10% separation gel) was performed using low molecular weight markers (20–120 kDa, Fermentas). Samples included purified recombinant laccase by Ni2+ affinity column, sample purified from culture solution of control strain and deglycosylated laccase sample. Deglycosylation of the purified laccase was performed with the deglycosylation kit (Sigma).
Enzyme activity assay
The laccase activity assay system contained 100 mmol/L sodium acetate (pH 4.6), 0.5 mmol/L ABTS and 100 μL culture supernatant, appropriately diluted in a total volume of 1 mL. The assay conditions were scaled down in microtitre plates (total volume of 200 μL). The change in absorbance at 420 nm of the mixture, kept at 25 °C for 3 min, was recorded with microplate reader (Bio-Rad). One unit of enzyme activity was defined as the amount of enzyme required to produce 1 µmol/L of oxidized product per min (ϵ420 ༝ 36,000 L/mol·cm). The protein concentration of the crude sample was determined by the Lowry method using a bovine serum albumin as a standard protein. The protein concentration was monitored based on the absorbance at 280 nm after anion-exchange and size exclusion chromatography (ÄKTA purifier systems).
Enzyme characterization
For biochemical characterization, the active laccase was reconstituted from the lyophilized powder by suspending in distilled water to a final activity of 100 U/L. The reconstituted enzyme was used to determine the pH and temperature optimum and stability by using ABTS as a substrate. The experiments were performed either in a glass cuvette or in a microtiter plate reader for multiple laccase measurements.
The pH dependency of the oxidation of ABTS (3.0 mmol/L) by the purified laccase was examined in buffers. The used buffers were 0.3 mol/L glycine–HCl buffer (pH 2–3), 0.3 mol/L sodium citrate buffer (pH 4–6) and 0.3 mol/L phosphate buffer (pH 7–8). All buffers were strong enough to buffer the corresponding pH even after addition of the acidic ABTS solution. The dependence of MLCC1 and NLCC1 activity on pH was determined. The pH stability was determined at 50 °C for 2 h at pH from 2.2 to 8.
The optimum temperature was determined at pH 4.6 by using temperatures ranging from 30 °C to 90 °C and the temperature stability was determined at pH 4.6 at temperatures of 50 °C, 60 °C and 70 °C for 4 h by using a temperature controlled spectrophotometer (Eppendorf). The assays contained enzyme (40 U/L) and 3.0 mmol/L ABTS in 0.1 mol/L citrate and 0.2 mol/L phosphate buffer (pH 4.6). The effect of the temperature on the enzyme stability was evaluated upon incubation of the purified laccase (40 U/L) in 10 mmol/L sodium acetate buffer (pH 4.6).
The optimum pH values of MLCC1 for ABTS, 2,6-dimethyl phthalate (DMP), guaiacol and 4-hydroxy-3,5-dimethoxybenzaldehyde azine (SGZ) were determined by using pH values from 4 to 6. The activity against these substrates was also detected after the addition of hydrogen peroxide. The kinetic parameters [Km (mmol/L) and Vmax (U/min)] of the NLCC1 and the MLCC1 towards ABTS and SGZ were compared. Spectrophotometric measurements of the substrate oxidation by NLCC1 and MLCC1 were carried out in a 2 mL reaction volume containing the test substrate in 50 mmol/L sodium citrate buffer (pH 4). All assays were carried out with equal units of laccase activity.
Statistical analyses
Variance analysis was performed by Statgraphics Plus version 5.1 statistical package, using the Least Significant Difference test at 5% level of probability to compare mean values of cell dry weight and laccase activities.
Results and discussion
Selection of mutation site
Given that laccases from different fungi have different characteristics, a molecular modification could be performed, according to the structural differences, to obtain the characteristics of interest. The sequence of the N-terminal part is often used as an indication for the origin of different genera. We compared reported laccases of different origins with good thermal stability, and the results are shown in . NLCC1 had higher similarity with fungal laccase (T. hirsuta) than with plant laccase (O. ficus-indica) and bacterial laccases (E. coli, Bacillus sp. LS04). A non-similar site was chosen as mutational, so as not to interfere with the catalytic specificity. The other thermotolerant laccases had hydrophobic or neutral amino acids on the site, whereas NLCC1 had acidic amino acid (aspartate) on the same site. Considering that the content of acidic amino acids is less than 10% in these thermotolerant laccases, we decided to change aspartate with a hydrophobic amino acid. Vogt et al. [Citation8] also showed that proline was more often on the thermophilic surface for improving the protein thermal stability. Also, B. subtilis CotA exhibited improved thermostability, most probably due to its higher proline content in concert with the tighter protein packing.[Citation19] This is the first report for improving the laccase thermostability through mutation of the N-terminal sequence.
Table 1. Alignment of the N-terminal sequence of NLCC1 with other thermotolerant laccases from different organisms.
The 14th residue of both proteins had a structure of beta sheet (). The substitution of D with P, which increased the size of the side chain, could further increase the packing of this region. This change could, in turn, influence the stability of the enzyme through increasing the compactness of the protein. Ala13 interacts with Ala24 by two hydrogen bonds, and Val15 also interacts with Arg22 by two hydrogen bonds. The link position 14 between Ala13 and Val15 plays an important role in the compactness of this region. The ring structure of proline was parallel with the surface composed of the backbone and hydrogen bonds, which was perhaps in favour of the maintenance of the beta-sheet structure compared with Asp14 (). This structural reinforcement upon mutation might confer rigidity to the protein, thus enhancing its stability. Also, the change of a polar amino acid with a bigger hydrophobic one might allow establishment of hydrophobic interactions with surrounding residues, which may further stabilize the protein structure in this region.[Citation20]
Expression of the P14D mutants
The constructs were successfully expressed in P. pastoris. The mutant proteins consisted of 498 amino acids. They were secreted in an active form, as checked by the transformants' growth on the plates supplemented with ABTS which was oxidized into a green radical cation by the laccase activity (data not shown). One clone showing the highest laccase activity was chosen for further investigation.
The highest laccase activity (194.06 U/L) was obtained after 54 h of high cell density culturing in a 5 L fermenter. After this the laccase activity dropped dramatically (). The concentrated glucose solution was automatically fed with a peristaltic pump when the residual glucose dropped and the DO concentration sharply changed (above 10%). When the concentrated glucose solution was automatically fed, the DO concentration would soon decrease. When DO concentration became below 30%, the feeding was stopped immediately. The time course of laccase activity using the DO feed-back feeding strategy is shown in . Since MLcc1 was constitutively expressed in P. pastoris, the laccase activity was increased with the cell growth, especially in a short period of time after feeding. The other fermentation conditions were the same as the ones in the batch fermentation culture. Although the laccase activity in constitutive Pichia system could not reach the same level as in the inducible system, the constitutive system is also of interest for producing laccase, owing to the convenience and production efficiency.[Citation21]
Purification and glycosylation status of the mutant laccase
At the end of the purification, originated from approximately 3.5 L liquid medium, 17 mg of purified enzyme was obtained with a specific activity of 11 U/mg, which corresponded to a final yield of 28% (). A single protein band was detected on the SDS-PAGE and the purified MLCC1 appeared to be a monomeric polypeptide of 82 kDa (, Lane 1). The mass of the purified MLCC1 was higher than the NLCC1 (∼64 kDa) (data not shown), indicating that it was heavily glycosylated.
Table 2. Purification of recombinant laccase.
After deglycosylation, the protein band was still observed, which showed a migration distance close to the 57 kDa standard (, lane 4), indicating that MLCC1 was post-translationally modified also in the P. pastoris expression system. Since the protein contained 45 serine, 51 threonine, 11 glutamine and 32 asparagine residues, where glycosylation often occurred, it was not a surprise that MLCC1 was heavily glycosylated. In addition, the molecular weight was higher than the previously reported, perhaps because proline could enhance the extent of O-linked glycosylation.[Citation22,Citation18]
Properties of MLCC1
MLCC1 showed optimum activity with ABTS after 10 min at pH 4.6 and 50 ° (a) and 5(c)), which was basically the same as the native enzyme. The pH had a very strong influence on the activity of both laccase preparations, with very low activity in slightly alkaline conditions (pH > 7), especially for NLCC1, and maximum activity between pH 4 and 5 ( (a) and 5(b)).
Figure 5. Effect of pH on the activity of mutant and native laccase (a) and pH tolerance of the mutant and the native laccase (b) with ABTS as substrate. Effect of temperature on the activity of mutant and native laccase (c) and temperature tolerance of the mutant and the native laccase (d) with ABTS as substrate.
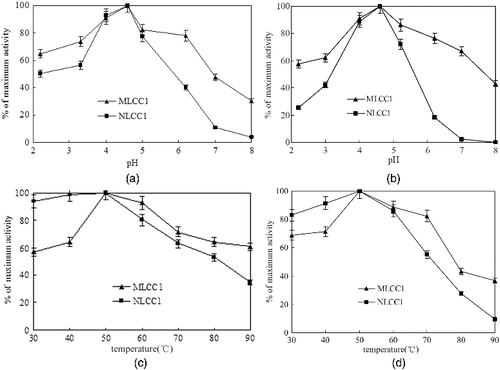
Lower activity was observed at pH 4.6 and 50 °C for 2 h, where 54.9% of the maximum activity was detected for MLCC1, whereas NLCC1 retained about 50% of its maximum activity. NLCC1 was less stable from pH 2.0 to pH 8.0 (except for pH 4.6) compared with MLCC1 and completely inactivated within 2 h at pH 8.0 (b)).
At pH 4.6, MLCC1 was the most stable between 30 °C and 70 °C, and was more stable than NLCC1. More than 20% of the residual activity was detected after up to 120 min at 90 °C for MLCC1, whereas less than 10% of the activity was retained for NLCC1 (d)).
Proline belongs to the hydrophobic amino acids, which could cause polycondensation with lipidic bilayers and effect the production of active laccase at a post-translational step in P. pastoris. The pH tolerance of the mutant laccase was also improved by strengthening the hydrophobic interaction between subunits. MLCC1 showed both improved pH and temperature stability, compared to native laccase ((b) and 5(d)). In laccases, both the overall 3D protein fold and packing, and minor structural modifications, e.g. at the copper sites, are responsible for the thermotolerant response. In addition, thermostability and thermotolerance result from co-action of more than one beneficial factors, such as existence of ionic bonds (salt bridges) and dense hydrogen bonding network.[Citation23] The N-terminal structure of the laccase from T. versicolor coils randomly in a 3D space.[Citation24] The rigid pyrrolidine ring of proline can make the conformation of the surroundings more reasonable by reducing the backbone unfolding entropy of proteins.[Citation25] The rigid structure surrounding proline possibly introduces more glycosyl in the protein, by which the temperature stability was improved. Yao et al. [Citation26] reported a mutant appAQ258N/Q349N which showed over 40% thermostability enhancement (85 °C for 10 min) and 4 °C–5 °C increase in the melting temperatures (Tm), compared with appA-WT, by enhancement of the glycosylation. Silva et al. [Citation27] also indicated that the underglycosylated form was sensitive to high temperatures probably because, in addition to stabilizing the protein conformation, glycosylation may also prevent unfolded or partially folded proteins from being aggregated.
More than 50% of the MLCC1 activity was retained after up to 120 min at 50 °C (). About 50% of the maximum activity was detected after up to 60 min at 60 °C. When the enzyme was incubated at its most stable pH of 4.6 for 1 h, it retained about half of its activity at 70 °C. The thermal stability of MLCC1was higher than that of laccase isoenzymes reported before, whereas the highest stability among four isoenzymes after 20 min of incubation at 70 °C was found for Lccβ with 62% of the residual activity.[Citation28] The MLCC1 activity dropped a little in the period between 1 and 3 h, at the tested temperature, which showed potential application in industrial processes.
MLCC1 was not only able to oxidize ABTS, but was also able to oxidize DMP, guaiacol and SGZ. The activity against these substrates did not increase after the addition of hydrogen peroxide, thereby excluding peroxidase activity. The optimum pH values for ABTS, DMP, guaiacol and SGZ oxidation were 4.6, 5.0, 5.2 and 5.6, respectively, which were a little different from NLCC1 ().
Table 3. Optimal pH values for different MLCC1 substrates.
Typical Michaelis–Menten kinetics was observed for MLCC1 towards the substrates ABTS and SGZ, which was comparable with that of NLCC1 (). Similar Km and Vmax values indicated functional similarity between the Pichia produced enzyme and that of the native fungus. Among the tested substrates, laccase showed higher affinity for ABTS, whereas lower Km values were obtained for SGZ. The catalytic characteristics of MLCC1 were slightly lower than those of NLCC1. This indicated that the better thermostability of MLCC1 was not due to the improved catalytic efficiency, but to its beneficial construction for protecting the functional sites by hindering heat conduction. The results were in accordance with the results from a previous report.[Citation26]
Table 4. Kinetic parameters of MLCC1 and NLCC1.
Conclusions
We have created a mutational laccase gene MLcc1 and expressed it in P. pastoris. The hyperglycosylated mutant protein had a molecular weight of 82 kDa. The recombinant laccase activity reached 194.06 U/L after 54 h by using high cell density fermentation in a 5 L fermenter. The mutant P14D showed more durable activity than the wild-type enzyme, displaying improved stability both at high temperature and at neutral and alkaline pH values. Compared with NLCC1, the kinetic parameters of MLCC1 toward ABTS and SGZ changed little by the introduced mutation.
Acknowledgements
Liang Huang and Yihan Liu contributed equally to this study.
Disclosure statement
No potential conflict of interest was reported by the authors.
Additional information
Funding
References
- Riva S. Laccases: blue enzymes for green chemistry. Trends Biotechnol. 2006;24:219–226.
- Giardina P, Faraco V, Pezzella C, et al. Laccases: a never-ending story. Cell Mol Life Sci. 2010;67:369–385.
- Baldrian P. Fungal laccases – occurrence and properties. FEMS Microbiol Rev. 2006;30:215–242.
- Asgher M, Iqbal HM, Irshad M. Characterization of purified and xerogel immobilized novel lignin peroxidase produced from Trametes versicolor IBL-04 using solid state medium of corncobs. BMC Biotechnol. 2012;12:46–54.
- Garg N, Bieler N, Kenzom T, et al. Cloning, sequence analysis, expression of Cyathus bulleri laccase in Pichia pastoris and characterization of recombinant laccase. BMC Biotechnol. 2012;12:75–87.
- Vieille C, Zeikus GJ. Hyperthermophilic enzymes: sources, uses, and molecular mechanisms for thermostability. Microbiol Mol Biol Rev. 2001;65:1–43.
- Christensen NJ, Kepp KP. Stability mechanisms of a thermophilic laccase probed by molecular dynamics. PLoS One. 2013;8:1371–1388.
- Vogt G, Argos P, Woell S. Protein thermal stability, hydrogen bonds, and ion pairs. J Mol Biol. 1997;269:631–643.
- Piscitelli A, Pezzella C, Giardina P, et al. Heterologous laccase production and its role in industrial applications. Bioeng Bugs. 2010;1:252–262.
- Piscitelli A, Giardina P, Mazzoni C, et al. Recombinant expression of Pleurotus ostreatus laccases in Kluyveromyces lactis and Saccharomyces cerevisiae. Appl Microbiol Biotechnol. 2005;69:428–439.
- Colao MC, Lupino S, Garzillo AM, et al. Heterologous expression of lcc1 gene from Trametes trogii in Pichia pastoris and characterization of the recombinant enzyme. Microb Cell Factories. 2006;5:31–36.
- Arana-Cuenca A, González-Becerra AE, Viniegra-González G, et al. Expression of a heterologous laccase by Aspergillus niger cultured by solid-state and submerged fermentations. Enzyme Microb Technol. 2006;38:665–669.
- Guo M, Lu F, Liu M, et al. Purification of recombinant laccase from Trametes versicolor in Pichia methanolica and its use for the decolorization of anthraquinone dye. Biotechnol Lett. 2008;30:2091–2096.
- Yano A, Kikuchi S, Nakagawa Y, et al. Secretory expression of the non-secretory-type Lentinula edodes laccase by Aspergillus oryzae. Microbiol Res. 2009;164:642–649.
- Li JF, Hong YZ, Xiao YZ, et al. High production of laccase B from Trametes sp. in Pichia pastoris. World J Microbiol Biotechnol. 2007;23:741–745.
- Ogino H, Ishikawa H. Enzymes which are stable in the presence of organic solvents. J Biosci Bioeng. 2001;91:109–116.
- Guex N, Peitsch MC, Schwede T. Automated comparative protein structure modeling with SWISS-MODEL and Swiss-PdbViewer: A historical perspective. Electrophoresis. 2009;30:S162–S173.
- Guo M, Lu F, Pu J, et al. Molecular cloning of the cDNA encoding laccase from Trametes versicolor and heterologous expression in Pichia methanolica. Appl Microbiol Biotechnol. 2005;69:178–183.
- Enguita FJ, Martins LO, Henriques AO, et al. Crystal structure of a bacterial endospore coat component. J Biol Chem. 2003;278:19416–19425.
- García-Ruiz E, Maté D. Ballesteros A, et al. Evolving thermostability in mutant libraries of ligninolytic oxidoreductases expressed in yeast. Microb Cell Factories. 2010;9:17.
- Maté D, García-Burgos C, García-Ruiz E, et al. Laboratory evolution of high-redox potential laccases. Chem Biol. 2010;17:1030–1041.
- Gemmill TR, Trimble RB. Overview of N- and O-linked oligosaccharide structures found in various yeast species. Biochimica Biophysica Acta. 1999;1426:227–237.
- Hildén K, Hakala TK, Lundell T. Thermotolerant and thermostable laccases. Biotechnol Lett. 2009;31:1117–1128.
- Bertrand T, Jolivalt C, Briozzo P, et al. Crystal structure of a four-copper laccase complexed with an arylamine: insights into substrate recognition and correlation with kinetics. Biochemistry. 2002;41:7325–7333.
- Zhu G, Teng M, Wang Y. Contribution of prolines to protein thermostability. Prog Biotechnol. 2000;20:48–51.
- Yao MZ, Wang X, Wang W, et al. Improving the thermostability of Escherichia coli phytase, appA, by enhancement of glycosylation. Biotechnol Lett. 2013;35:1669–1676.
- Silva TM, Almeida FB, Damásio AR, et al. Tunicamycin inhibition of N-glycosylation of α-glucosidase from Aspergillus niveus: partial influence on biochemical properties. Biotechnol Lett. 2010;32:1449–1455.
- Koschorreck K, Richter SM, Swierczek A, et al. Comparative characterization of four laccases from Trametes versicolor concerning phenolic C-C coupling and oxidation of PAHs. Arch Biochem Biophys. 2008;474:213–219.