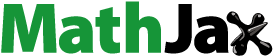
ABSTRACT
The fields in which lead (Pb) finds application in the modern world have increased dramatically in recent years. As a consequence of this intensive utilization of Pb, its toxicity tends to pose more and more environmental problems. The aim of this study was to evaluate the genotoxic potential of Pb and to characterize some physiological parameters in Secale cereale under Pb stress. Plants were subjected to different exposure levels of Pb (0, 100, 200 and 400 µmol/L) for two weeks. At the end of the experimental period, the effects of Pb exposure on the photosynthetic pigments content (chlorophyll a and b, total chlorophyll, chlorophyll a/b and carotenoids) and genetic material of S. cereale were studied. To evaluate the genotoxic effect of Pb, random amplified polymorphic DNA – polymerase chain reaction (RAPD-PCR) was employed. The obtained results showed alteration in the photosynthetic pigments content and RAPD-PCR profiles of S. cereale grown in the presence of Pb. The alterations in the RAPD-PCR profiles following Pb treatments appeared to be losses of normal bands and occurrences of new bands compared to unexposed plantlets. Overall, the content of chlorophyll a, chlorophyll b, total chlorophyll and carotenoids decreased by 6.68%, 6.08%, 2.89% and 8.57%, respectively, under severe Pb stress (400 µmol/L).
Introduction
Heavy metals exert toxic effects on living species, and heavy-metal bioaccumulation is observed with different latency periods, even lasting for decades.[Citation1] The toxicity level mainly depends on the type of heavy metal and its concentration. However, some heavy metals, e.g. Cu, Fe, Mn, Mo, Ni and Zn, are involved in a number of biological processes essential for organisms but, in excessive amounts, can cause many toxicity-related health conditions.[Citation2,Citation3] Other heavy metals, including As, Cd, Cr (for plants), Hg and Pb, even when present in minute quantities, are potentially toxic for organisms.[Citation2,Citation4] In humans, accumulation of heavy metals mainly occurs through contaminated foodstuff, water or air.[Citation5] In the last few decades, the level of heavy metals in the environment has significantly increased, mainly due to anthropogenic factors, e.g. misuse of agricultural, transportation and industrial activities,[Citation6–9] and natural factors, including volcanic activity, leakage from rocks, radon emission and some natural disasters (earthquakes, floods, storms and forest fires etc.),[Citation6,Citation10] causing contamination of the environment.
One of the hazardous heavy metals that exist in different forms in the environment is lead (Pb). It exists in some organic compounds, e.g. tetraethyl lead; inorganic compounds, e.g. lead arsenates, lead chromates, lead chloride, lead oxide, lead sulphate and lead silicates; and in a mineral form [Citation11]. The major sources of Pb pollution are accumulators, batteries, cable sheathing, lead sheets, combustion of lead-containing petrol, solders, pigments, ceramics, photographic materials and explosives manufacturing.[Citation2,Citation12,Citation13] As a consequence, the concentration of Pb as an environmental pollutant is directly correlated with industrialization and urbanization. Because of its highly toxic nature for living systems, regular monitoring of Pb is a necessity in health-related programmes.[Citation6] Pb has been reported to reduce the cognitive/intellectual development and the glomerular filtration rate, and to increase blood pressure and the incidence/risk of cardiovascular disease.[Citation6,Citation11] Pb affects plant growth and development by interfering with plant metabolism, including binding to the sulfhydryl groups of proteins, which causes disruption of protein structure or inhibition of protein activity. The deleterious effects resulting from Pb exposure can also be observed in plants as a consequence of disturbance in the uptake and/or distribution of other essential elements.[Citation14] In addition, ROS can be formed as a result of Pb stress, causing oxidative damages in cells.[Citation14,Citation15].
Secale cereale (rye), belonging to the Triticaceae family, is a wild species mainly distributed in central and eastern Turkey, as well as in neighbouring regions. It is used as a cover crop plant to improve soil fertility/quality and for protection of agricultural fields from the invasive behaviour of weed species.[Citation16]
In the present study, the possible physiological and genotoxic effects of Pb in rye were investigated to gain a better understanding of the effects of Pb pollution on living species. The obtained results may be used to assess the degree of pollution in the environment by comparative analysis of the data from our work and data obtained from screening and monitoring of the environment. This may facilitate the use and planning of possible measures for the detection and prevention of Pb pollution posing risk of detrimental effects on species, especially plants.
Materials and methods
S. cereale plantlets and in vitro growth conditions
The seeds of S. cereale var. Aslim-95 used in this work were obtained from Bahri Dagdas International Agricultural Research Institute. For surface-sterilization, S. cereale seeds were treated with 70% (v/v) ethyl alcohol for 15 min, rinsed three times in deionized water for 5 min, then immersed in 20% (v/v) commercial bleach (ACE®) for 10 min and rinsed three times with deionized water. Sterilized seeds were placed in small vessels containing Murashige and Skoog (MS) medium [Citation17] used for germination. After germination, the 3–4 cm long plantlets were grown in vitro in MS solid medium containing 0, 100, 200 and 400 µmol/L of Pb in the form of Pb(NO3)2. They were incubated in a growth chamber. The temperature and relative humidity were maintained at 23 ± 2 °C and 50%–55%, respectively, and fluorescent tubes were used as a light source providing photosynthetically active radiation of 5000 μmol/(m2 s), with a day/night cycle of 14 h/10 h. The pH value was adjusted to 5.8 in the control and experimental groups. The plantlets were harvested at the end of the 14-day experimental period, and the subsequent measurements were carried out.
Total chlorophyll, chlorophyll a, chlorophyll b and carotenoids content in S. cereale
The amounts of photosynthetic pigments were determined according to Arnon.[Citation18] Briefly, 0.5 g of leaf material from each sample was used for the extraction of photosynthetic pigments in 15 mL of 80% acetone (v/v). Centrifugation was performed at +4 ºC and 3000g for 10 min. Following centrifugation, the volume of supernatant for each sample was measured and the content of photosynthetic pigments in each supernatant was determined spectrophotometrically (PerkinElmer-Optima 7000 DV) at 645, 663 and 470 nm. The total chlorophyll, chlorophyll a, chlorophyll b and carotenoids concentrations were calculated using the following equations:where Ca is the chlorophyll a concentration (mg/mL), Cb is the chlorophyll b concentration (mg/mL), CTotal is the total chlorophyll concentration (mg/mL), Cx+c is the carotenoids concentration (mg/mL), D663, D645 and D470 are the optical densities at 663, 645 and 470 nm, respectively, and V is the volume of the supernatant obtained after the centrifugation (mL).
Determination of total protein in S. cereale roots
The content of total soluble proteins was determined spectrophotometrically, according to Bradford [Citation19]. Briefly, 1 g of fresh root material was homogenized in 3 mL of 0.1 mol/L phosphate solution (pH 7.7). Centrifugation was performed at +4 ºC and 12,000g for 20 min and the supernatants were collected for measurement of the total soluble protein content. Bradford reagent (5 mL) was added to 100 µL of each supernatant sample and, after 5 min, measurements were made at 595 nm and the protein content was determined using a standard curve. The standard curve was prepared with a series of dilutions of bovine serum albumin.
Determination of Pb accumulation in S. cereale
The leaf and root samples of plants subjected to Pb stress and plants not treated with Pb (as controls), were dried in an oven at 80 °C for 48 h and then the samples were pulverized using a micro hammer-cutter mill (porcelain) and passed through a 1.5-mm sieve. Aliquots of 0.2 g from each sample were weighed and placed into Teflon vessels and then, dissolved in 8 mL of 65% HNO3 (v/v). Next, minerilization of the samples was performed in a microwave oven (Berghof -- MWS2) as follows: at 145 °C for 5 min, at 165 °C for 5 min and at 175 °C for 20 min. After cooling, Whatman filters (Macherey-Nagel 125 mm, 2 µm pore size) were used for filtration of the samples and 50 mL of ultra pure water was added to each filtered sample in a volumetric flask. The samples were then stored in falcon tubes. A standard solution was prepared and Pb concentrations were determined using inductively coupled plasma optical emission spectroscopy (PerkinElmer-Optima 7000 DV).
Assessment of the genotoxic potential of Pb in S. cereale
In this study, the RAPD (random amplified polymorphic DNA) technique was employed for assessment of the potential genotoxic effect of Pb exposure. The RAPD profiles of root samples of S. cereale plantlets exposed to Pb were compared to those of untreated (control) samples. DNA was extracted using the Promega Wizard Genomic DNA Purification Kit, according to the supplier's instructions. The concentrations of the obtained genomic DNA were determined using BioSpec-nano Micro-volume UV–Vis Spectrophotometer (Shimadzu Scientific Instruments).
Polymerase chain reaction (PCR) products were obtained by using seven different primers (RAPD İontek®) for RAPD analysis and primer 4 (5'-CTGAGGTCTC-3') produced specific and stable band profiles in our study. The PCR reaction mix contained 2.5 μL of PCR buffer (10X), 1 μL of deoxynucleoside triphosphates (dNTP) mix (2.5 mmol/L), 3 μL of primer (10 mmol/L), 50–100 ng of genomic DNA and 0.5 μL (5 U) of Taq DNA polymerase; the final volume was adjusted to 25 μL with sterile deionized water. A negative control containing all reaction components except template DNA was set up for each reaction. A Techne® Endurance TC-512 Gradient Thermal Cycler was used for the amplifications. The PCR programme was as follows: initial denaturation at 94 °C for 2 min; followed by 45 cycles of denaturation at 94 °C for 30 s, primer annealing at 35 °C for 30 s, elongation at 72 °C for 2 min and a final extension step at 72 °C for 7 min.
Amplification products were separated in a 1% agarose gel electrophoresis. A molecular size marker (Gene Ruler™ 100 bp DNA Ladder, ready-to-use, Thermo Scientific) was used. Following ethidium bromide staining, DNA bands were visualized under UV light. The results were documented using an 0WL EASYCAST B2 system (Thermo Scientific) and the Gel Analyzer 2010 program. The genomic instability was evaluated based on the genomic template stability (GTS) using the following equation: GTS = [1 − (a/n)] × 100, where a is the total number of polymorphic bands in each sample and n is the total number of bands in the controls. DNA variations were detected as alterations including loss and gain of bands in profiles.
Statistical analysis
Data presented in figures and tables are means from eight independent experiments with standard deviations (±SD). Data were analyzed statistically using one-way analysis of variance (ANOVA) with Tukey's post hoc HSD (honestly significant difference) test. IBM SPSS v. 20 software was used for statistical analyses. Means were considered significantly different at P < 0.01 (**) and P < 0.05 (*).
Results and discussion
The toxic effects of Pb on plants depend on its concentration, the salt-forming capacity, the soil properties and the plant species. In plants, toxic levels of Pb cause damage to different cellular functions, including targeting metal ions in functional groups of macromolecules, photosynthesis, regulation of water capacity by altering related enzyme activities and mineral nutrient metabolism.[Citation20]
Effect of Pb treatment on the content of photosynthetic pigments
In the present study, growth of S. cereale seedlings in the presence of different Pb concentrations (0–400 µmol/L) for 14 days was observed to be associated with fluctuations in the chlorophyll a, b, a/b, total chlorophyll and carotenoids content (). The results showed that, at the end of the 14-day experimental period, there was an increase in the content of chlorophyll a (by ∼10.34% and ∼24.39%), chlorophyll b (by ∼10.22% and ∼44.66%), total chlorophyll (by ∼3.24% and ∼27.12%) and carotenoids (by ∼10.10% and ∼28.04%) with 100 and 200 µmol/L Pb treatments, respectively, whereas there was a reduction in the content of chlorophyll a (by ∼6.68%), chlorophyll b (by ∼6.08%), total chlorophyll (by ∼2.89%), carotenoids (by ∼8.57%) with 400 µmol/L Pb treatment, compared with the controls. Also, there was an increase of ∼4.48% in the content of chlorophyll a/b under the level of 100 µmol/L Pb treatment, whereas there was a reduction of ∼18.75% and ∼16.47% in the content of chlorophyll a/b under 200 and 400 µmol/L Pb treatments, respectively, compared with the controls. Overall, the results () showed a trend for an increase in the photosynthetic pigments content (chlorophyll a, chlorophyll b, chlorophyll a/b, total chlorophyll and carotenoids) in S. cereale treated with 100 and/or 200 µmol/L Pb. When the level of Pb was increased to 400 µmol/L, there was a trend for a reduction in the photosynthetic pigments content.
Figure 1. Pigment concentrations in control and Pb-treated S. cereale leaves: chlorophyll a (a), chlorophyll b (b), total chlorophyll (c), chloropyll a/b (d) and carotenoids (e).
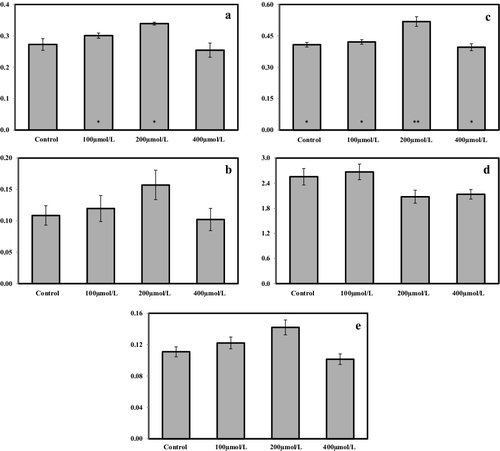
The statistical analysis (ANOVA with Tukey's post hoc HSD test) revealed a significant difference only regarding the chlorophyll a and the total chlorophyll content. For the chlorophyll a content, there was a significant difference (P < 0.05) between the control, 100 and 200 µmol/L Pb treatment groups; therefore, the results of Tukey's post hoc HSD test produced no subsets. Regarding the total chlorophyll content, there were significant differences between the control, 100 and 400 µmol/L Pb treatment groups (P < 0.05) and between the control and 200 µmol/L treatment groups (P < 0.01). With respect to the results from Tukey's post hoc HSD, the control, 100 and 400 µmol/L treatment groups were taken in one subset and the 200-µmol/L treatment group was taken in another subset.
In a similar study in Triticum aestivum,[Citation21] the contents of chlorophyll a, b, total chlorophyll and carotenoids were reduced under Pb stress (0, 500, 1000 and 2500 µmol/L). Similar results were reported in Brassica rapa, where at the end of a six-day experimental period, there was a negative correlation between the content of chlorophyll a, b and carotenoids and the applied Pb levels (0, 0.5, 1, 3 and 5 mmol/L).[Citation22] The reduction rates (%) were 57.4% for chlorophyll a, 68.5% for chlorophyll b and 38.1% for carotenoids under 5 mmol/L Pb(NO3)2 treatment.[Citation22]
Based on our results, it could be speculated that the increase in the content of photosynthetic pigments in the leaves of S. cereale seedlings following exposure to 100 and 200 µmol/L Pb could be considered an attempt to partly compensate the negative effects of Pb by mobilizing appropriate defence mechanisms for alleviation of stress. However, at the level of 400 µmol/L Pb treatment, the photosynthetic pigment content decreased, suggesting that, after a certain point, Pb stress probably becomes insurmountable for the plants. These results are in accordance with the report of Kumar et al. [Citation23], in which Talinum triangulare was used as a model plant and different Pb levels (0, 0.25, 0.5, 0.75, 1.0 and 1.25 mmol/L) were applied. Increments were seen in the content of chlorophyll a, chlorophyll b and carotenoids under 0.25 and 0.5 mmol/L Pb treatment, whereas reductions in the content of these pigments were observed when higher levels of Pb were applied. According to our results, the synthesis of photosynthetic pigments was promoted under tolerable Pb treatment levels (100 and 200 µmol/L) for the plant. The production of photosynthetic pigments is one of the events that occur in most plants exposed to heavy-metal stress. [Citation22–24] The survival and plant development at excess Pb exposure (400 µmol/L) was quite low. Pb adversely affects the mechanisms involved in plant growth and synthesis of photosynthetic pigments.[Citation22,Citation25–27]
Pb accumulation in S. cereale leaves and roots
As a next step in our study, the concentrations of Pb in the leaves and roots of S. cereale grown in the presence of different Pb concentrations were determined. The results showed that the concentration of Pb in the leaves and roots dramatically increased when different amounts of Pb were added in the culture medium (). Progressive accumulation of Pb was observed in all studied plant parts proportionally to the levels of Pb in the culture medium.
Effect of Pb treatment on the protein content
Oxidative stress in cells due to the generation of ROS is known to cause lipid peroxidation, enzyme inactivation and genetic material damage at the biochemical level.[Citation28] In our study, reductions in the total protein content status in the roots of S. cereale were observed at all levels of Pb treatment (). Under 100, 200 and 400 µmol/L Pb treatment, there was a reduction in the content of total protein by ∼46.29%, ∼29.27% and ∼22.02%, respectively, compared with the control. The rate of reduction was most severe under 100 µmol/L Pb treatment. Although there was a decrease in the total protein content at the other treatment levels, the rate of decline decreased compared to control. It could be speculated that these results could be interpreted as evidence that Pb stress activated the SOS response mechanism in the plants, thereby increasing the content of related SOS response proteins in the plant cells.
Genotoxic potential of Pb in S. cereale root tips
To evaluate the possible genotoxic potential of different Pb concentrations on the genetic material of S. cereale root cells, a comparative analysis of the RAPD-PCR profiles of treated and non-treated samples was performed. The RAPD-PCR primer produced different band patterns in Pb-treated and unexposed (control) samples (, ). The comparisons between the RAPD profiles of root-tip cells of Pb-treated and non-treated plants were done on the basis of the changes in the specific RAPD bands. The band patterns and molecular sizes of the amplification products obtained with the primer showed that extra bands (949, 965 and 761 bp) appeared in the 100 and 200 μmol/L Pb treatment variants, whereas some normal bands (311 and 1073 bp) disappeared at 200 and 400 μmol/L treatment levels. This may be related to the level of DNA damage, since there was a positive correlation between the losses and gains of bands and the applied Pb concentrations in our study. GTS was considered as a quantitative measure of the alterations in the RAPD profiles resulting from changes in DNA (). At the highest level of Pb exposure (400 µmol/L), the GTS was decreased, which correlated well with the decrease in the photosynthetic pigments content in the leaves and the total protein content in the roots of S. cereale seedlings ( and ).
Figure 4. Representative RAPD profiles of genomic DNA from root-tips of S. cereale plantlets exposed to different Pb concentrations. Lane M: Gene Ruler™ 100 bp DNA ladder; Lane S1: control plants (unexposed to Pb treatment); Lane S2: 100 µmol/L Pb-treated plants; Lane S3: 200 µmol/L Pb-treated plants; Lane S4: 400 µmol/L Pb-treated plants).
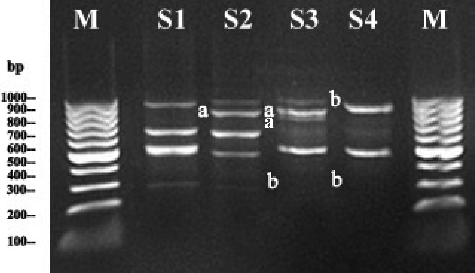
Table 1. Representative results of RAPD profile alterations in the number and molecular size (bp) of appearing/disappearing bands as detected with primer 4 (CTGAGGTCTC) in root-tip cells of S. cereale plantlets exposed to different Pb concentrations and unexposed control plantlets.
Figure 5. Effects of Pb on genomic template stability (%) in S. cereale grown in the presence of different Pb concentrations (0, 100, 200 and 400 μmol/L) for two weeks.
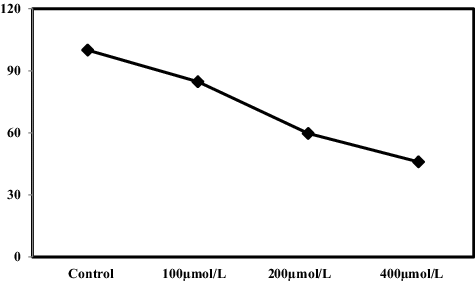
Excessive concentrations of heavy metals can generate different types of DNA damage, including single- and double-strand breaks, modified bases, abasic sites, DNA-protein cross-links and oxidized bases.[Citation29,Citation30] Changes in DNA structure produced by the events involving different types of lesions and mutations can affect the kinetics of PCR.[Citation31,Citation32] New PCR products could be formed owing to the occurrence of new oligonucleotide priming sites complementary to oligonucleotide primers following changes or alterations in DNA sequences due to mutations (deletions, inversions, insertions etc.) and/or homologous recombination, resulting in new annealing events.[Citation33] RAPD profile alterations with other parameters have been used in evaluation of genomic DNA template stability and detection of genotoxic damage induced by bio-accumulation of pollutants.[Citation34,Citation35]
Final remarks
The results from our study showed decreases in the content of total protein and photosynthetic pigments and in genomic DNA stability in S. cereale seedlings grown under Pb stress and there was a correlation between these parameters. Our data showed that Pb itself mainly accumulated progressively in all parts of the plant when different levels of exogenous Pb were applied. The reduction in the photosynthetic pigment levels in S. cereale plantlets correlated well with the observed changes in the RAPD profiles ( and). The data suggest that the extent of the DNA damage seems serious mainly in the cells in leaf tips of S. cereale plantlets. Our findings are in agreement with those reported by other researchers. For example, lead-induced toxicity resulted in reduced photosynthetic pigment content and occurrence of different RAPD-PCR patterns in the leaves of Brassica rapa following Pb exposure.[Citation22] Changes, including reduction of growth, increase in the total soluble protein level and alterations in the RAPD profiles, were observed following ascending cadmium (Cd) exposure in root tips of barley (Hordeum vulgare).[Citation36] When increasing the levels of boron (B) and zinc (Zn) exposures, reductions in GTS and total soluble protein content and increased polymorphism rate in the RAPD profiles were found in Zea mays.[Citation37] The effects of metals (Cd, Pb and Zn) on the biomass, root-shoot length and photosynthetic pigments of Hibiscus rosa sinensis suggested that heavy-metal stress influences the photosynthetic pigment contents and also induces DNA changes during remediation.[Citation38] Significant decreases in photosynthetic pigment contents and genetic alterations were detected in Egyptian clover and Sudan grass after Cd treatment in a dose-dependent manner.[Citation39] Thus, the ever-growing amount of data about the detrimental effects of Pb on plants suggests that Pb toxicity tends to be a problem particularly in the environment due to its extensive utilization in the developing world.
Conclusions
In the present research, the possible physiological and genotoxic effects of Pb in S. cereale were investigated for better understanding of the effects of Pb pollution on living species and assessment of the degree of Pb pollution in the environment based on comparison of the data from this work and those obtained from environmental screening and monitoring. In our study, induced genomic alterations and changes in some physiological parameters were detected in S. cereale grown in the presence of Pb. Furthermore, the obtained results suggest that RAPD-PCR data and physiological parameters can be used together for the estimation of Pb pollution.
Disclosure statement
No potential conflict of interest was reported by the authors.
Additional information
Funding
References
- Mihucz VG, Csog A, Fodor F, et al. Impact of two iron (III) chelators on the iron, cadmium, lead and nickel accumulation in poplar grown under heavy metal stress in hydroponics. J Plant Physiol. 2012;169:561–566.
- Osma E, Ozyigit II, Leblebici Z, et al. Determination of heavy metal concentrations in tomato (Lycopersicon esculentum Miller) grown in different station types. Rom Biotechnol Lett. 2012;17:6962–6974.
- Liu G, Yu Y, Hou J, et al. An ecological risk assessment of heavy metal pollution of the agricultural ecosystem near a lead-acid battery factory. Ecol Indicators. 2014;47:210–218.
- Yoon Y, Lee WM, An YJ. Phytotoxicity of arsenic compounds on crop plant seedlings. Environ Sci Pollut Res. 2015;22:11047–11056.
- Madhu P, Neelam G, Koninika M, et al. Microalgae in removal of heavy metal and organic pollutants from soil. In: Das S, editor. Microbial biodegradation and bioremediation. Waltham (MA): Elsevier; 2014. p. 521–540.
- Das S, Raj R, Mangwani N, et al. Heavy metals and hydrocarbons: adverse effects and mechanism of toxicity. In: Das S, editor. Microbial biodegradation and bioremediation. Waltham (MA): Elsevier; 2014. p. 23–54.
- Yurong Y, Yingying S, Henrik VS, et al. Community structure of arbuscular mycorrhizal fungi associated with Robinia pseudoacacia in uncontaminated and heavy metal contaminated soils. Soil Biol Biochem. 2015;86:146–158.
- Osma E, Ozyigit II, Demir G, et al. Assessment of some heavy metals in wild type and cultivated purslane (Portulaca oleracea L.) and soils in Istanbul, Turkey. Fresen Environ Bull. 2014;23:2181–2189.
- Ugulu I, Dogan Y, Baslar S, et al. Biomonitoring of trace element accumulation in plants growing at Murat Mountain. Int J Environ Sci Technol. 2012;9:527–534.
- Prasad MNV, Freitas H, Fraenzle S, et al. Knowledge explosion in phytotechnologies for environmental solutions. Environ Pollut. 2010;158:18–23.
- Avino RB, Lopez-Moya JR, Navarro-Avino JP. Health implications: trace elements in cancer. In: Prasad MNV, editor. Trace elements as contaminants and nutrients: consequences in ecosystems and human health. Hoboken (NJ): Wiley; 2008. p. 495–522.
- Koz B, Cevik U. Lead adsorption capacity of some moss species used for heavy metal analysis. Ecol Indicat. 2014;36:491–494.
- Zulkali MMD, Ahmad AL, Norulakmal NH. Oryza sativa L. husk as heavy metal adsorbent: optimization with lead as model solution. Bioresource Technol. 2006;97:21–25.
- Liu T, Liu S, Guan H, et al. Transcriptional profiling of Arabidopsis seedlings in response to heavy metal lead (Pb). Environ Exp Bot. 2009;67:377–386.
- Schutzendubel A, Polle A. Plant responses to abiotic stresses: heavy metal-induced oxidative stress and protection by mycorrhization. J Exp Bot. 2002;53:1351–1365.
- Cimmino A, Fernandez-Aparicio M, Avolio F, et al. Ryecyanatines A and B and ryecarbonitrilines A and B, substituted cyanatophenol, cyanatobenzo [1, 3] dioxole, and benzo [1, 3] dioxolecarbonitriles from rye (Secale cereale L.) root exudates: novel metabolites with allelopathic activity on Orobanche seed germination and radicle growth. Phytochemistry. 2015;109:57–65.
- Murashige T, Skoog F. A revised medium for rapid growth and bioassays with tobacco tissue cultures. Physiol Plantarum. 1962;15:473–497.
- Arnon DI. Copper enzymes in isolated chloroplasts, polyphenoxidase in Beta vulgaris. Plant Physiol. 1949;24:1–15.
- Bradford MM. A rapid and sensitive method for quantitation of microgram quantities of protein utilizing the principle of protein dye-binding. Anal Biochem. 1976;72:248–254.
- Lamhamdi M, Bakrim A, Aarab A, et al. Lead phytotoxicity on wheat (Triticum aestivum L.) seed germination and seedlings growth. Comptes Rendus Biologies. 2011;334:118–126.
- Kaur G, Singh HP, Batish DR, et al. Growth, photosynthetic activity and oxidative stress in wheat (Triticum aestivum) after exposure of lead to soil. J Environ Biol. 2012;33:265–269.
- Cenkci S, Cigerci IH, Yildiz M, et al. Lead contamination reduces chlorophyll biosynthesis and genome template stability in Brassica rapa L.. Environ Exp Bot. 2010;67:467–473.
- Kumar A, Prasad MNV, Syta O. Lead toxicity, defense strategies and associated indicative biomarkers in Talinum triangulare grown hydroponically. Chemosphere. 2012;89:1056–1065.
- Shakya K, Chettri MK, Sawidis T. Impact of heavy metals (copper, zinc and lead) on the chlorophyll content of some mosses. Arch Environ Con Toxicol. 2008;54:412–421.
- Sharma P, Dubey RS. Lead toxicity in plants. Braz J Plant Physiol. 2005;17:35–52.
- Sengar RK, Gautam M, Sengar RK, et al. Lead stress effects on physiobiochemical activities of higher plants. Rev Environ Contam Toxicol. 2008;196:73–93.
- Pourrut B, Shahid M, Dumat C, et al. Lead uptake, toxicity, and detoxification in plants. Rev Environ Contam Toxicol. 2011;213:113–136.
- Reddy AM, Kumar SG, Jyothsnakumari G, et al. Lead induced changes in antioxidant metabolism of horsegram (Macrotyloma uniflorum Lam. Verdc.) and bengal gram (Cicer arietinum L.). Chemosphere. 2005;60:97–104.
- Bisova K, Hendrychova J, Cepak V, et al. Cell growth and division processes are differentially sensitive to cadmium in Scenedesmus quadricauda. Folia Microbiol. 2003;48:805–816.
- Hsiao CJ, Stapleton SR. Characterization of Cd-induced molecular events prior to cellular damage in primary rat hepatocytes in culture: activation of the stress activated signal protein JNK and transcription factor AP-1. J Biochem Mol Toxicol. 2004;18:133–142.
- Bowditch BM, Albright DG, Williams JG, et al. The use of randomly amplified polymorphic DNA markers in comparative genome studies. Method Enzymol. 1993;224:294–309.
- Pan J, Yu L. Effects of Cd or/and Pb on soil enzyme activities and microbial community structure. Ecol Eng. 2011;37:1889–1894.
- Atienzar FA, Cordi B, Evenden AJ. Qualitative assessment of genotoxicity using random amplified polymorphic DNA: comparison of genomic template stability with key fitness parameters in Daphnia magna exposed to benzo[a]pyrene. Environ Toxicol Chem. 1999;18:2275–2282.
- Atienzar FA, Cordi B, Donkin ME. Comparison of 442 ultraviolet-induced genotoxicity detected by random amplified polymorphic DNA with chlorophyll fluorescence and growth in a marine macroalgae. Aquat Toxicol. 2000;50:1–12.
- Liu W, Li PJ, Qi XM, et al. DNA changes in barley (Hordeum vulgare) seedlings induced by cadmium pollution using RAPD analysis. Chemosphere. 2005;61:158–167.
- Liu W, Yang YS, Li PJ, et al. Risk assessment of cadmium-contaminated soil on plant DNA damage using RAPD and physiological indices. J Hazard Mater. 2009;161:878–883.
- Erturk, FA, Nardemir G, Ay H, et al. Determination of genotoxic effects of boron and zinc on Zea mays using protein and random amplification of polymorphic DNA analyses. Toxicol Ind Health. 2015;31:1015–1023.
- Bhaduri AM, Fulekar MH. Biochemical and RAPD Analysis of Hibiscus rosa sinensis induced by heavy metals. Soil Sediment Contamin. 2015;24:411–422.
- Aly AA. Application of DNA (RAPD) and ultrastructure to detect the effect of cadmium stress in Egyptian clover and Sudan grass plantlets. J Stress Physiol Biochem. 2012;8:241–257.