ABSTRACT
Development of effective pathogen detection systems is critical for human health. In this study, a micro-gapped interdigitated electrode array-based immunosensor chip is constructed for the detection of a typical pathogen, Escherichia coli (E. coli) O157:H7. The immunosesnor chip was prepared via photolithography and characterized by a digital camera, optical microscope and scanning electron microscope. The E. coli O157:H7 detection mechanism is based on an enzyme-linked, sandwiched immunoassay with enzymatic silver deposition. Our results revealed that the proposed immunosensor chip showed selective electrical response towards E. coli O157:H7 over other bacteria including Hemolytic streptococcus, Staphylococcus aureus, Salmonella, Pseudomonas aeruginosa, Chronobacter sakazakii and Bacillus cereus. Our assay exhibited potential practical applications for E. coli O157:H7 determination in water and our methodology might be applied to detect different pathogens by simply selecting specific antibodies.
Abbreviations | ||
AAP | = | ascorbic acid phosphate enzyme |
ALP | = | alkaline phosphatase |
APTES | = | 3-aminopropyl triethoxysilane |
BSA | = | bovine serum albumin |
EDS | = | energy-dispersive spectroscopy |
ELISA | = | enzyme-linked immunosorbent assay |
LSV | = | linear sweep voltammetry |
PBS | = | phosphate-buffered saline |
SEM | = | scanning electron microscope |
TB | = | Tris-HCl buffer |
PBST | = | phosphate-buffered saline tween |
Introduction
According to the statistics of the World Health Organization, more than 1.8 million people worldwide died from diarrhoeal diseases in 2005, mostly caused by microbiologically contaminated food or drinking water [Citation1]. Therefore, early detection and an identification method of threatening pathogens are of great importance for public health and safety. In particular, Escherichia coli O157:H7 is one of the most dangerous pathogens [Citation2]. E. coli O157:H7 can cause serious food-borne illnesses and leads to enormous economic losses. For example, E. coli O157:H7 may cause bloody diarrhoea, haemorrhagic colitis and hemolytic uremic syndrome. An infection dose of ∼100 cells can cause disease or even death, especially for children and elderly people [Citation3]. The Center for Disease Control and Prevention reported that an estimated 73,000 infection cases and 61 deaths associated with E. coli O157:H7 occur in the United States each year [Citation4]. Outbreaks of intestinal infections caused by E. coli O157:H7 occurred in Shandong, Shanghai and Beijing in 1987. Several events of food-borne outbreaks of E. coli O157:H7 were also reported in England and Wales in 1992–2002 [Citation5]. Therefore, developing a selectively sensitive E. coli O157:H7 detection method is of significant importance to prevent outbreaks of food-/water-borne illnesses.
Towards this goal, many approaches have been developed for E. coli O157:H7 detection, such as culture-based method, polymerase chain reaction (PCR)-based technique, gold nanoparticle-labelled lateral flow immunoassay and matrix-assisted laser desorption ionization mass spectrometry [Citation6–12]. For instance, MacConkey medium containing sorbitol, not lactose, is widely used to score E. coli O157:H7 counts [Citation9], followed by serological verification with O157- and H7-specific antisera, which is one of the traditional laboratory methods. However, for some strains, failure to produce β-glucuronidase would cause false negatives. Zhang et al. [Citation13] utilized a polyclonal antibody and a monoclonal antibody against intimin γ1 to develop a double antibody sandwich enzyme-linked immunosorbent assay (ELISA) to measure E. coli O157:H7 with a detection limit of 1 × 103 cells/mL in culture. Still, the sensitivity of the ELISA technique is not satisfactory, although a lot of efforts have been devoted to improving it [Citation14,Citation15]. Electrochemical approaches have also been developed to determine E. coli O157:H7, but they involved modification of electrodes and signal amplification. For example, Li et al. [Citation16] proposed an electrochemical impedance immunosensor for E. coli O157:H7 detection in the range of 102–107 cells/mL. Guo et al. [Citation17] designed an electrochemical immunosensor assay for highly sensitive and specific detection of E. coli O157:H7 with a detection limit of 34 cells/mL by modifying the electrodes with antibody–nanoparticle composite. Chips are very small and integrated units of sample pre-treatment, purification, enrichment and detection, which are also used for detection of pathogens. By using antibodies to capture E. coli O157:H7 and bench-top real-time quantitative PCR to enumerate [Citation18], even six cells can be counted. Most of these approaches exhibit favourable results; notwithstanding, there are some shortcomings such as requirement of complicated procedures and poor reproducibility. Thus, to develop sensitive detection of E. coli O157:H7 is still appealing.
Recently, immunity gap interpolation microarray chips, a combination of micro-machining technology and biological immunity, have attracted growing attention because of their high sensitivity and throughput detection capabilities [Citation19]. Facing with huge challenges of food safety bacterium testing and modern clinical bacterium medicine, the core mission of chip research and development is how to use advanced micro-processing means to manufacture microarrays and combine antigen–antibody reactions to develop sensors. Currently, the base material used for the production of interdigitated microarray chips includes silicon, silica, polydimethylsiloxane, polymethyl methacrylate, etc. [Citation20–22]. The immunity gap interpolation microarray technology coupled with nanomaterials provides considerable sensitivity and simplicity compared to traditional methods [Citation23,Citation24]. Hence, the immunity gap interpolation microarray technology shows promising potential in the development of sensitive E. coli O157:H7 detection methods.
In this paper, a micro-gapped interdigitated electrode array-based electronic biosensing system was constructed for the detection of E. coli O157:H7. The sensing principle is based on the fact that E. coli in tap water promotes the binding of alkaline phosphatase labelled E. coli O157:H7 polyclonal antibody, which catalyses the deposition of silver and subsequently enhances the electrochemical signals. The proposed system exhibited high specificity towards E. coli O157:H7 over other bacteria due to the antigen–antibody immunoreaction. To the best of our knowledge, this is the first work showing micro-gapped interdigitated electrode array-based E. coli O157:H7 sensors through this specific immunoreaction, which can minimize interference from other bacteria. The effects of parameters on the sensitivity of the micro-gapped interdigitated electrode array for the immunoreaction were investigated in the paper. The applicability of this system was further validated by detecting E. coli O157:H7 in water samples.
Materials and methods
Bacteria and chemicals
Phosphate-buffered saline (PBS) and glutaraldehyde were purchased from Beijing Ding States Biological Reagent Company (Beijing, China). Bovine serum albumin (BSA), alkaline phosphatase (ALP), 3-aminopropyl triethoxysilane (APTES) and ascorbic acid phosphate (AAP) were purchased from Sigma (Milwaukee, WI, USA). E. coli O157:H7 polyclonal antibody was purchased from Shanghai Hui Yun Biotechnology Company (Shanghai, China). E. coli O157:H7 monoclonal antibody was purchased from Promab Inc. (Richmond, VA, USA). Other chemical reagents were of analytical grade purity. The buffer solutions used in this experiment were 10 mmol/L PBS (pH7.4, 0.1 mol/L NaCl); 20 mmol/L Tris-HCl buffer (TB, pH8.0) AZ4330 positive photoresist and developer were purchased from Microchem Company (Milwaukee, WI, USA). Glass slides (3 inches) were purchased from chrome version Changsha Shaoguang Ltd (Shanghai, China). ELISA 96-well plates were purchased from NEST companies (Jiangsu, China); 96-hole and 24-hole cell culture plates were provided by CORNING Company in the United States. E. coli O157:H7 (NCTC 12 900), hemolytic Streptococcus (CMCC(B)32 210), Staphylococcus aureus (ATCC 6538), Salmonella (CMCC(B)50 115), Pseudomonas aeruginosa (CMCC(B) 50 115), Chronobacter sakazakii (ATCC 29 004) and Bacillus cereus (CMCC(B)63 303) were obtained from the corresponding ATCC, CMCC, NCTC agents. These bacterial strains were kept in semi-solid medium at 4 ˚C. During the whole experiment, all of the bacteria separation steps were performed in a biological safety cabinet, and the waste was sterilized at 121 ˚C for 20 min before discarding.
Apparatus
Photographs of micro-gapped interdigitated electrode array were obtained using a D7100 digital camera (Nikon, Japan). Images of the electrode under optical microscopy were taken using a Nikon Ni-U optical microscope (Nikom, Japan), which was equipped with a 100-W halogen tungsten lamp, a 10 × objective and a colour charge-coupled device (Olympus, Japan). Scanning electron microscope (SEM) images and energy-dispersive spectroscopy (EDS) were collected with a Quanta 650 scanning electron microscope (FEI, Hillsboro Oregon, USA). The electric signals were obtained using a ChI 760C electrochemical workstation (Huachen Ltd., Shanghai, China).
Preparation of lithographic microelectrode chip
The lithographic microelectrode chip was prepared based on a previously reported protocol [Citation19], as illustrated in . First, the commercial glass slide was cleaned as described in [Citation25] and dried in a Class 100 clean oven. Then, a 300-nm-thick gold film was coated onto the surface via sputtering coating method. Second, the gold-plated glass surface was covered with positive resist AZ4330 by spin coating and the glass sides were kept at 90 ˚C for 30 min. The designed electrode patterns were formed via ultraviolet (UV) exposure for 60 s, then the non-pattern-covered positive resist was removed through a development process for 2 min. Third, the cleaned chip was held at 130 ˚C for 30 min to solidify the pattern and all uncovered gold film was removed. Next, the glass was cut into the designed size and shape with a numerical control cutting machine to obtain gold electrode substrate. Finally, 96-well ELISA plates and gold electrode substrate were combined through physical adhesion under 37 ˚C for 1 h to form a stable chip.
Fabrication of antibody-modified microelectrode immunosensor chip
Antibody-modified microelectrode immunosensor chip was constructed based on a previously reported one-pot approach with slight modifications [Citation19,Citation26,Citation27]. Briefly, the cleaned microelectrode chip was immersed in absolute ethanol containing 2.5% APTES and 1% H2O for 24 h. After being rinsed thoroughly with absolute ethanol, the microelectrode chip was dried under nitrogen flow and stored at 4 °C for 1 h. The silanized microelectrode chip was immersed in 5.0% glutaraldehyde solution for 1 h, followed by a rinse with water. Next, the microelectrode chip was incubated in a PBS solution of E. coli O157:H7 monoclonal antibody (0.1 mg/mL) for 1 h at 37 ˚C. In order to remove excess antibody, the microelectrode chip was then washed twice with PBS. Then the microelectrode chip was incubated in 1% BSA PBS solution for another 1 h at 37 ˚C to block non-specific adsorption sites. The antibody-modified microelectrode chip was washed with ultrapure water and stored at 4 ˚C for further use.
Preparation of ALP-labelled E. coli O157:H7 polyclonal antibody
ALP-labelled E. coli O157:H7 polyclonal antibody was prepared as follows: E. coli O157:H7 polyclonal antibody (2 mg) and ALP (5 mg) were solubilized in 1 mL PBS solution, and 20 μL 2.5% glutaraldehyde solution was added into the PBS solution. The mixture was incubated at room temperature for 2 h. The mixture was then dialysed for 12 h with PBS and TB to remove unreacted chemicals and reactants. Then, the reaction mixture was dialysed with PBS and TB solution for 12 h. Finally, the reaction mixture was diluted with 4 mL TB containing 1% BSA, and then 1.6 mL of glycerol was added. The alkaline phosphatase-labelled E. coli O157:H7 polyclonal antibody solution was stored at −20 ˚C.
Detection of E. coli O157:H7
E. coli O157:H7 was cultured in semi-solid medium. In our experiment, the semi-solid medium was set prepared as follows: 1 g peptone, 0.3 g meat extract, 0.5 g NaCl, and 0.35–0.4 g agar were dissolved in 100 mL distilled water, pH 7.4.
For the detection of E. coli O157:H7, monoclonal antibody-modified microelectrode chip was mixed with 20 μL sample solution containing varying concentrations (103–108 CFU/mL) of E. coli O157:H7 incubated at 37 °C for 30 min, and then 40 μL ALP-labelled E. coli O157:H7 polyclonal antibody solution and incubated at 37 °C for another 30 min. After PBS washing, 25 μL silver enhancement solution (0.1 mol/L glycine–NaOH buffer, containing 1 mmol/L AAP and 5 mmol/L AgNO3, pH 9.0) was added onto the microelectrode surface, and maintained in the dark for 10 min at 37 °C to reduce silver ions into metallic silver. Then, the silver-deposited microelectrode chip was washed twice with ultrapure water and then dried. E. coli O157:H7 with various concentrations were tested using the same protocol. And the E. coli O157:H7 samples were prepared exactly following the standard methods. The concentration of microbial cells was determined based on optical density.
Electrochemical signal measurements
We performed electrochemical measurements at room temperature using a 760C CHI electrochemical system. The microelectrode chip was placed in the air, one pole was connected to the working electrode and the other pole was connected to the reference and counter electrodes. Linear sweep voltammetry (LSV) measurements were conducted over an electric potential range of 0–50 mV at the scan rate of 1 mV/S and with an interval of 1 mV. The curves of current versus potential of different samples were recorded. The electrical conductivity could be calculated by the slope of the curve and acted as the analytical signal for quantitative purposes.
Results and discussion
Preparation of microelectrode immunosensor chip
The detailed preparation procedure for constructing microelectrode immunosensor chips followed the earlier report with slight modification [Citation19]. They were characterized by digital camera, optical microscope and SEM. The lithographic microelectrode chip was prepared following the design shown in . To prevent the connection of nearby microelectrode elements, the length and width of the microelectrode strip were designed to be 77.0 and 17.8 mm, respectively. After combining with 96-well ELISA plates, the gap between adjacent electrode elements was around 0.2 mm. The photographs of as-prepared 96-well combined microelectrode chip are shown in . It can be seen that each well combined with a single microelectrode element. The actual structure of a single microelectrode element is displayed in (C); the adjacent microelectrodes from two electrodes were isolated, which is essential for the assay.
Principle of E. coli O157:H7 detection
The microelectrode immunosensor working principle was based on an enzyme-linked sandwiched immunoassay with enzymatic silver deposition for electrical detection of E. coli O157:H7. The mechanism for E. coli O157:H7 detection consists of two parts as shown in . E. coli O157:H7 monoclonal antibody was covalently immobilized on the gap of adjacent microelectrodes through a silanization layer for capturing the analyte. The introduction of an E. coli O157:H7 sample together with ALP-labelled E. coli O157:H7 polyclonal antibody results in the formation of a sandwiched complex of E. coli O157:H7 with the capture E. coli O157:H7 monoclonal antibody and the detection of ALP-labelled E. coli O157:H7 polyclonal antibody on the surface of the microelectrode gap due to the specific immunoreaction. Then, the bound ALP catalyses the hydrolysis of AAP and generates ascorbic acid. The resulting ascorbic acid acts as a reducing agent and changes silver ions into metallic silver.
Figure 4. Schematic illustration of the microelectrode-based immunosensor for the detection of E. coli O157:H7.
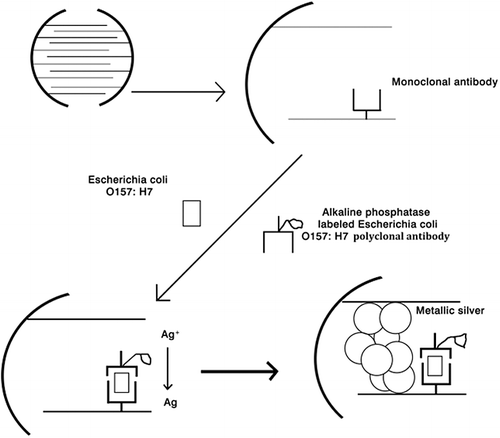
(A,B) show the micrographs obtained by using an optical microscope for the microelectrode array-based immunosensor in response to control and 104 CFU/mL E. coli O157:H7 in the presence of silver enhancement solution, respectively. In (A), the gold fingers are shown as clean, and the adjacent microelectrodes are independent. In contrast, in (B), it was seen clearly that part of the microelectrodes were covered, suggesting deposition of metallic silver on the microelectrode surface. Further evidence for this hypothesis was supported by SEM images. As shown in (C), the surface of microelectrodes was clean and there was no deposition of silver. However, huge particles on the surface of microelectrodes were observed when E. coli O157:H7 was added, as shown in (D), indicating the formation of metallic silver. Moreover, the EDS spectra also proved the formation of metallic silver. The small range from (D) was selected to measure the EDS spectra. As shown in (B), the EDS spectrum showed the composition of gold on the adjacent microelectrodes. And the composition of these huge particles was primarily silver ((C)). These observations demonstrated that the ALP-based enzymatic reaction provides an efficient route to produce a large number of dispersed silver particles over the gap.
Figure 5. Clean (A), sediment appearance in gap (B) under ordinary optical microscope × 10. Clean (C), sediment appearance in gap (Dt) under scanning electron microscope.
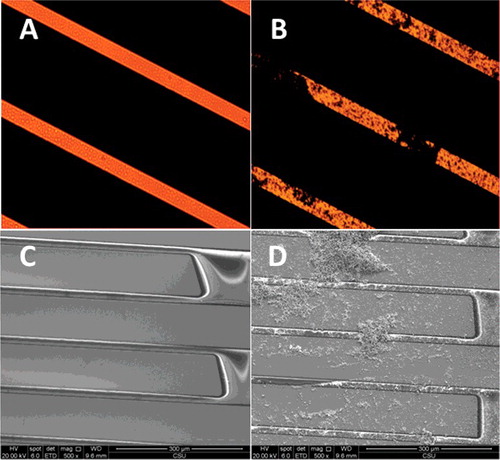
Figure 6. Sediment appearance in gap (A), scanning electron microscope. Electrode element display of Au (B) under energy-dispersive spectroscopy. Sediment appearance display of Ag (C) under energy-dispersive spectroscopy.
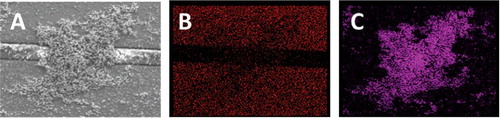
These produced silver particles not only connected the adjacent microelectrodes, but also increased the electrical conductance of the microelectrode chip and caused the variation of current. Such an increasing of electrical conductance is proportional to the concentration of E. coli O157:H7, according to Ohm's law. LSV analysis of the immunosensor over a potential range from 0 to 50 mV was also done in the detection of 108 E. coli O157:H7 and in controlled experiments (data not shown) after the introduction of AAP and silver ion solution. All the control experiments were conducted at the same condition. The modification of the electrodes by monoclonal antibody did not lead to the production of current even increasing the potential of the electrode. No obvious electrical signal was observed without E. coli O157:H7 or ALP-labelled E. coli O157:H7 polyclonal antibody solution. It was found that a strong signal was measured only in the presence of both E. coli O157:H7 and ALP-labelled E. coli O157:H7 polyclonal antibody solution. This is because the sandwich structure formed only with the addition of E. coli O157:H7 and ALP-labelled E. coli O157:H7 polyclonal antibody solution and only the caught ALP can catalyse the hydrolysis of AAP and formation of silver particles. The LSV curve was found to exhibit linearity in the potential range, indicating that the immunosensor behaves as an ideal physical resistor under the measurement conditions.
Performance of the E. coli O157:H7 detection system
The LSC measurements were also conducted with a series of increasing E. coli O157:H7 concentrations (). At a fixed electric potential, the electrical current intensity increased gradually with increasing the E. coli O157:H7 concentration from 103 to 108 CFU/mL. For a constant concentration of E. coli O157:H7, the increasing electric potential led to the elevation of the electrical current intensity. This indicated that increasing electric potential would reduce the limit of detection (LOD), while too high electric potential would be harmful for the electrodes. In addition, the electrical current intensity displayed a good linear relationship versus E. coli O157:H7 concentrations ranging from 103 to 108 CFU/mL. Note that a calibration curve must be newly constructed when changing the polyclonal antibody because of its diversity. The lowest LOD was 200 CFU/mL with a signal-to-noise ratio of 3. Based on these results, the high sensitivity might be attributed to the enzymatic silver deposition that provides specific deposition on the microelectrode surface and amplifies the electrical signals. Although the selectivity is not as high as that of the method reported in the literature [Citation16,Citation17], our assay still has great application value because, in real sample detection cases, enrichment of bacteria is normally necessary. In particular, bacteria usually divide every 20 min and the cell counts could reach 521 after 3 h of culture for one bacterial cell, which could finally exceed the LOD of our assay.
To investigate whether the aggregation induced by E. coli O157:H7 is specific, the LSV measurements were conducted by adding various bacteria such as hemolytic Streptococcus, S. aureus, Salmonella, P. aeruginosa, C. sakazakii and B. cereus. None of these bacteria (108 CFU/mL) could cause a conspicuous current change as E. coli O157:H7 (108 CFU/mL) did at 50 mV (). In fact, the current intensity in the presence of E. coli was almost 100-fold higher than that in the presence of other bacteria. The present technique provided better selectivity compared to other E. coli O157:H7 sensing assays [Citation9]. Such a high selectivity of the microelectrode immunosensor can contribute to the specific immunoreaction. Taken together, the results obtained showed that the microelectrode immunosensor could provide an effective electrochemical platform for the analysis of E. coli O157:H7. Our chips showed many advantages when compared to ELISA. In ELISA, chromogenic reactions are conducted by several steps in solution and should be inhaled by adding other chemicals. Moreover, the reactants solution could not be stored for a long time. In contrast, our method does not need chromogenic steps. The results could be repeatedly measured after several months because the silver deposited on the surface will not change. Now, we are also working towards development of a set of equipment to eliminate the need of manual operation to make the production convenient and the chip cheaper. Therefore, for E. coli O157:H7 analysis, the microelectrode immunosensor is simple, with comparable or better sensing performance as compared to other assays, especially ELISA [Citation13–15,Citation28,Citation29].
Practical application of the E. coli O157:H7 detection system
To investigate the potential application of the designed system in real cases, we tested tap water of Chagnsha with the microelectrode immunosensor. The tap water did not need pre-treatment and 20 μL sample solution and 40 μL ALP-labelled E. coli O157:H7 polyclonal antibody solution were added to the micropore and incubated at 37 °C for 30 min. After PBS washing, 25 μL silver enhancement solution containing 0.1 mol/L glycine–NaOH buffer, 1 mmol/L AAP and 5 mmol/L AgNO3 (pH 9.0) was added on the microelectrode surface, and then it was maintained in the dark for 10 min at 37 °C to reduce silver ions into metallic silver. No E. coli O157:H7 was detected. By adding E. coli to the tap water with ultimate 103 CFU/mL, the current at 50 mV was of 0.85 pA. The concentration of E. coli was 9.8 × 102 CFU/mL. Since E. coli O157:H7 in water is not permitted by the World Health Organization, the high sensitivity suggests that the microelectrode array-based immunosensors can be applied to practical clinical analysis.
Conclusions
To the best of our knowledge, this study is the first to report a new electrochemical method to detect E. coli O157:H7 via micro-gapped interdigitated electrode array. Highly specific E. coli O157:H7 detection was achieved based on a well-known immunoreaction, which endows the immunosensors with satisfying interference rejection of over 100-fold compared to other bacteria. Our study demonstrated a combination of the specific immunoreaction and silver deposition signal amplification; thus new avenues for the design of micro-gapped interdigitated electrode array immunosensors for other pathogens based on a similar strategy might open up in the clinical and related fields. The device of the micro-gapped interdigitated electrode array may find convenient and wide applications in everyday life.
Disclosure statement
The authors declare no conflict of interest.
Additional information
Funding
References
- Newell DG, Koopmans M, Verhoef L, et al. Food-borne diseases – the challenges of 20 years ago still persist while new ones continue to emerge. Int J Food Microbiol. 2010;139(1):S3–S15.
- Su C, Brandt LJ. Escherichia coli O157:H7 infection in humans. Ann Intern Med. 1995;123(9):698–707.
- Fagerquist CK, Garbus BR, Miller WG, et al. Rapid identification of protein biomarkers of E scherichia coli O157:H7 by matrix-assisted laser desorption ionization-time-of-flight−time-of-flight mass spectrometry and top-down proteomics. Anal Chem. 2010;82(7):2717–2725.
- Rangel JM, Sparling PH, Crowe C, et al. Epidemiology of Escherichia coli O157:H7 outbreaks, united states, 1982–2002. Emerging Infect Dis. 2005;11(4):603–609.
- Gillespie IA, O''Brien SJ, Adak GK, et al. Foodborne general outbreaks of shiga toxin-producing E scherichia coli O157 in England and Wales 1992-2002: where are the risks? Epidemiol Infect. 2005;133(5):803–808.
- Lopez-Velasco G, Tomas-Callejas A, Sbodio AO, et al. Factors affecting cell population density during enrichment and subsequent molecular detection of Salmonella e nterica and Escherichia coli O157:H7 on lettuce contaminated during field production. Food Control. 2015;54(1):165–175.
- Jokerst JC, Adkins JA, Bisha B, et al. Development of a paper-based analytical device for colorimetric detection of select foodborne pathogens. Anal Chem. 2012;84(6):2900–2907.
- Ganz K, Gill A. Inhibition of polymerase chain reaction for the detection of Escherichia coli O157:H7 and Salmonella e nterica on walnut kernels. Food Microbiol. 2013;35(1):15–20.
- Kim SR, Yoon Y, Seo MK, et al. Modification of methods for detection of Escherichia coli O157:H7 on produce. Food Sci Biotechnol. 2014;23(4):1349–1356.
- Donhauser SC, Niessner R, Seidel M. Sensitive quantification of Escherichia coli O157:H7, Salmonella enterica, and Campylobacter jejuni by combining stopped polymerase chain reaction with chemiluminescence flow-through DNA microarray analysis. Anal Chem. 2011;83(8):3153–3160.
- Won JY, Min J. Highly sensitive Escherichia coli O157:H7 detection in a large volume sample using a conical polymer tube chamber consisting of micro-glass beads. Biosens Bioelectron. 2010;26(1):112–117.
- Gomez A, Miller NS, Smolina I. Visual detection of bacterial pathogens via PNA-based padlock probe assembly and isothermal amplification of DNAzymes. Anal Chem. 2014;86(24):11992–11998.
- Zhang X, Meng L, Zhang B, et al. Development of a sandwich ELISA for EHEC O157:H7 intimin γ1. Plos One. 2016;11(9):e0162274. DOI: 10.1371/journal.pone.0162274.
- Qi G, Han JJ, Shan S, et al. DNA-based hybridization chain reaction and biotin–streptavidin signal amplification for sensitive detection of Escherichia coli O157:H7 through ELISA. Biosens Bioelectron. 2016;86(1):990–995.
- Shan S, Liu D, Qi G, et al. Sensitive detection of Escherichia coli O157:H7 based on cascade signal amplification in ELISA. J Dairy Sci. 2016;99(9):7025–7032.
- Li Z, Fu Y, Fang W, et al. Electrochemical impedance immunosensor based on self-assembled monolayers for rapid detection of Escherichia coli O157:H7 with signal amplification using lectin. Sensors. 2015;15(8):19212–19224.
- Guo Y, Wang Y, Liu S, et al. Electrochemical immunosensor assay (EIA) for sensitive detection of E. coli O157:H7 with signal amplification on a SG-PEDOT-AUNPS electrode interface. Analyst. 2014;140(2):551–559.
- Dharmasiri U, Witek MA, Adams AA, et al. Enrichment and detection of Escherichia coli O157:H7 from water samples using an antibody modified microfluidic chip. Anal Chem. 2010;82(7):2844–2849.
- Huang Y, Wang TH, Jiang JH, et al. Prostate specific antigen detection using microgapped electrode array immunosensor with enzymatic silver deposition. Clin Chem. 2009;55(5):964–971.
- Silva LB, Baptista P, Raniero L, et al. Novel optoelectronic platform using an amorphous/nanocrystalline silicon biosensor for the specific identification of unamplified nucleic acid sequences based on gold nanoparticle probes. In: Proceedings from TRANSDUCERS and EUROSENSORS '07, 4th International Conference on Solid-State Sensors, Actuators and Microsystems; 2007 Jun 6–10; Lyon. IEEE, Netherlands: Elsevier; 2007. p. 935–938.
- Li Y, Yan X, Feng X, et al. Agarose-based microfluidic device for point-of-care concentration and detection of pathogen. Anal Chem. 2014;86(21):10653–10659.
- Moralesnarváez E, Naghdi T, Zor E, et al. Photoluminescent lateral-flow immunoassay revealed by graphene oxide: highly sensitive paper-based pathogen detection. Anal Chem. 2015;87(16):8573–8577.
- Li D, Feng Y, Zhou L, et al. Label-free capacitive immunosensor based on quartz crystal Au electrode for rapid and sensitive detection of Escherichia c oli O157:H7. Anal Chim Acta. 2011;687(1):89–96.
- Yuan Z, Du Y, Tseng Y-T, et al. Fluorescent gold nanodots based sensor array for proteins discrimination. Anal Chem. 2015;87(8):4253–4259.
- Xu D, He Y, Yeung ES. Direct imaging of transmembrane dynamics of single nanoparticles with darkfield microscopy: improved orientation tracking at cell sidewall. Anal Chem. 2014;86(7):3397–3404.
- Avrameas S. Coupling of enzymes to proteins with glutaraldehyde: use of the conjugates for the detection of antigens and antibodies. Immunochemistry. 1969;6(11):43–52.
- Sheppard NF, Tucker RC, Wu C. Electrical conductivity measurements using microfabricated interdigitated electrodes. Anal Chem. 2002;65(9):1199–1202.
- Feng M, Yong Q, Wang W, et al. Development of a monoclonal antibody-based ELISA to detect Escherichia coli O157:H7. Food Agric Immunol. 2013;24(4):481–487.
- Song C, Liu C, Wu S, et al. Development of a lateral flow colloidal gold immunoassay strip for the simultaneous detection of Shigella boydii and Escherichia coli O157:H7 in bread, milk and jelly samples. Food Control. 2016;59(1):345–351.