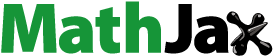
ABSTRACT
The aim of the present study was to evaluate the protective effects of free and encapsulated quercetin against oxidative stress injury in neuronal SH-SY5Y cells and isolated rat brain synaptosomes. Quercetin (QR) was encapsulated in nanoparticles consisting of sodium alginate and chitosan and the ratio between both biopolymers was 10:1 or 1:10, respectively. The nanoparticles formulated with higher amount of sodium alginate were negatively charged, whereas those with higher chitosan amount were positively charged. The protective effects of free and encapsulated quercetin were studied in two in vitro models: H2O2-induced oxidative stress in human neuroblastoma SH-SY5Y cells and 6-hydroxydopamine (6-OHDA) induced neurotoxicity in isolated rat brain synaptosomes. Comparing to free quercetin, quercetin encapsulated in the nanoparticles formulated with higher chitosan concentration exerted more pronounced neuroprotective activity in a model of H2O2-induced (1 mmol/L H2O2, 15 min) oxidative stress in neuroblastoma SH-SY5Y cells. A similar trend was observed in a model of 6-OHDA induced neurotoxicity in rat brain synaptosomes. In conclusion, encapsulation of quercetin in nanoparticles based predominantly on chitosan improved its neuroprotective activity in vitro.
Introduction
The high incidence of neuronal pathologies and the lack of specific treatments are important key points for searching effective protective agents for prevention and treatment of neurodegenerative diseases. Among the natural compounds, flavonoids have been reported to exert neuroprotective properties. Quercetin (QR; 3,3′,4′,5,7-pentahydroxyflavone) is a flavonol possessing a wide spectrum of pharmacological activities including antioxidant, anti-inflammatory, anticancer, anti-angiogenic, antibacterial activities, etc. Its capacity to scavenge free radicals is associated with protection of cells from oxidative stress which is closely associated with various diseases. Oxidative stress has been proposed to play an important role in the pathogenesis of different neurodegenerative diseases [Citation1–3]. In Parkinson's disease, for example, oxidative stress may occur after increased dopamine turnover, thus raising the excessive levels of reactive oxygen species (ROS) such as hydrogen peroxide, superoxide anion (O2·−) and hydroxyl radical (OH·) [Citation4]. Some reports have pointed out the role of quercetin as an agent capable of attenuating the overproduction of ROS [Citation5] as well its capacity to prevent glutathione (GSH) oxidation [Citation6]. Treatment with quercetin has been reported to reverse the increased lipid peroxidation and decreased GSH, superoxide dismutase (SOD) and catalase levels in ethanol-intoxicated mice [Citation7]. Pretreatment of primary rat neuronal cells with low doses of quercetin has demonstrated protective effects against amyloid beta-peptide induced toxicity by modulating oxidative stress [Citation8]. In addition, quercetin could be therapeutically effective because of its anti-inflammatory properties [Citation9].
However, the clinical use of quercetin is hindered due to its hydrophobic properties, instability, poor oral bioavailability and limited permeability through the blood-brain barrier. The development of nanoparticulate delivery systems could overcome some of these limitations. Solid lipid nanoparticles were developed aiming to improve the permeation of quercetin across the blood-brain barrier and eventually to improve its therapeutic efficacy in Alzheimer's disease [Citation10]. Quercetin encapsulated in polylactide-co-glycolide nanoparticles has demonstrated a significant level of protection in different brain regions after ischemia-reperfusion-induced neuronal damage in rats [Citation11].
The aim of the present study was to evaluate the possibility to improve the neuroprotective effects of quercetin by its encapsulation in chitosan-alginate nanoparticles. The neuroprotective effects of free and encapsulated quercetin were studied in two in vitro models: H2O2-induced oxidative stress in neuroblastoma SH-SY5Y cells and 6-hydroxydopamine (6-OHDA) induced neurotoxicity in rat brain synaptosomes. To evaluate the effects of the treatment, SH-SY5Y cells viability, synaptosomal viability and intra-synaptosomal content of GSH were used as markers of oxidative damage.
Materials and methods
Reagents
Sodium alginate (very low viscosity) was supplied by Alfa Aesar GmBH & CoKG (Karlsruhe, Germany). Chitosan (Mv 110 000–150 000), quercetin, HEPES (anhydrous ≥99.5%), sodium pyruvate, L-glutamine, fetal bovine serum (FBS), RPMI-1640, tripsin/ethylenediaminetetraacetic acid (EDTA), dimethylsulfoxide (DMSO), penicillin, streptomycin, hydrogen peroxide solution 30% (w/w), Percoll (pH 8.5–9.5) and 3-[4,5-dimethylthiazol-2-yl]-2,5diphenyl-tetrazolium bromide were purchased from Sigma–Aldrich (Germany). D-glucose, NaCl, KCl, 6-hydroxydopamine (6-OHDA) and 2,2'-dinitro-5,5'-dithiodibenzoic acid (DTNB) were provided by Merck (Darmstadt, Germany).
Encapsulation of quercetin in chitosan–alginate nanoparticles
The nanoparticles were prepared by electrostatic gelation of both biopolymers adapted from a previously reported study [Citation12]. The nanoparticles were prepared applying a ratio of 1:10 or 10:1 (wt/wt) between the polymers.
Chitosan–alginate nanoparticles (QR-NP1)
Sodium alginate solution (3 mg/mL) was incubated with 400 µL ethanol solution of quercetin (0.825 mg/mL) for 30 min. After that, a solution of calcium chloride (3.35 mg/mL) was added to their mixture under ultrasound for 1 min at 20 kHz (Bandelin Sonopuls, Germany). Finally, the solution of chitosan (0.75 mg/mL) was added drop by drop under ultrasound for 2 min in order to achieve gelation between both polymers. Finally, the dispersion was gently stirred for 4 h (700 r/min), centrifuged (14 000 r/min, MICRO 120, Hettich, Germany) and rinsed with purified water.
Chitosan–alginate nanoparticles (QR-NP2)
Acidic solution of chitosan (3 mg/mL) was incubated with the respective concentration of quercetin for 30 min. After that, a solution of calcium chloride was slowly added to their mixture. Then, the solution of alginate (0.75 mg/mL) was added drop by drop in order to achieve gelation between the two polymers. In both stages, the dispersion was treated with ultrasound as described above (20 kHz, Bandelin Sonopuls, Germany). Finally, the resulting dispersion was gently stirred for 4 h (700 r/min), centrifuged (14 000 r/min, MICRO 120, Hettich, Germany) and rinsed with purified water.
Characterization of the nanoparticles
The size and zeta potential were determined by photon correlation spectroscopy and electrophoretic laser doppler velocimetry (Zetamaster analyzer, Malvern Instruments, UK). The freshly prepared dispersions were measured at 25 °C with a scattering angle of 90o.
The encapsulation of quercetin was evaluated by determination of the difference between its initial concentration and the concentration found in the supernatants obtained by centrifugation of freshly prepared nanoparticulate dispersions. The amount of non-encapsulated drug was measured by spectrophotometry at λ = 375 nm (Thermo Scientific, Madison, USA). The standard curve was made in the range of 4–20 µg/mL (r = 0.9997). The encapsulation efficiency (EE) was calculated using the following equation:
Cell-based assays
Human neuroblastoma cells SH-SY5Y were obtained from Sigma Aldrich (ECACC cell lines). Cells were routinely cultured in RPMI containing 10% (v/v) fetal calf serum, 2 mmol/L glutamine, 50 mg/mL penicillin and 100 mg/mL streptomycin and were kept at 37 °C in humidified 5% CO2.
The cell viability was evaluated after incubation of SH-SY5Y cells with the samples for 24 h. Briefly, SH-SY5Y cells were seeded in 96-well microplates at a density of 2 × 104 cells/well and were allowed to attach to the well surface for 24 h at 37 °C in a humidified atmosphere with 5% CO2. Thereafter, empty nanoparticles, free and encapsulated quercetin were added to cells and incubated for a period of 24 h. For each concentration, a set of at least 8 wells were used. The cell viability was estimated by MTT-dye (3-(4,5-dimethylthiazol-2-yl)-2,5-diphenyltetrazolium bromide) reduction assay [Citation13]. After 24 h of incubation, 10 µL MTT solution (5 mg/mL in phosphate buffered saline; PBS) aliquots were added to each well. After 3 hours of incubation, intracellularly formed formazan crystals were dissolved in 100 µL DMSO and the absorbance was measured in a plate reader (Synergy 2, BioTek Instruments) at 570 and 690 nm for background absorbance.
In the oxidative stress model, SH-SY5Y cells at a density of 5 × 104 cells/well were exposed to 1 mmol/L H2O2 for 15 min to obtain submaximal cytotoxicity. In order to estimate protection, cells were pretreated with free or encapsulated quercetin (10 µg/mL) and empty nanoparticles for 2 h before treatment with H2O2. The cell viability was evaluated after 24 h by MTT-dye reduction assay as described above. Moreover, the cells were photographed under a phase contrast microscope Leica DMI400B (Leica microsystem CMA GmbH, Mannheim, Germany).
Evaluation of protective effect on rat brain synaptosomes
Male Wistar rats (200–250 g, provided by National Breeding Centre, Sofia, Bulgaria) were housed in plexiglass cages (3 per cages) in a 12/12 light/dark cycle, temperature 20 ± 2 °C. Food and water were provided ad libitum. All experiments were performed after at least one week of adaptation to this environment. The experimental procedures were approved by the Institutional Animal Care and Use Committee at the Medical University of Sofia, Bulgaria. The guidelines stated in the Principles of Laboratory Animal Care (NIH publication #85-23, revised in 1985) were followed strictly throughout the experiment.
Synaptosomes were prepared from the brains of the rats, as previously described by Taupin et al. [Citation14]. The brains were homogenized with a Teflon-glass homogenizer in 10 volumes (w/v) of cold Buffer A, containing: 5 mmol/L HEPES and 0.32 mol/L sucrose (pH = 7.4). The brain homogenate was centrifuged twice at 1000g for 5 min at 4 °C. The supernatant was collected and centrifuged 3 times at 10 000g for 20 min at 4 °C. The pellet was resuspended in ice-cold Buffer A. The synaptosomes were isolated by using Percoll reagent to prepare the gradient. Synaptosomes were resuspended and incubated in Buffer B, containing: 290 mmol/L NaCl, 0.95 mmol/L MgCl2.6H2O, 10 mmol/L KCl, 2.4 mmol/L CaCl2.H2O, 2.1 mmol/L NaH2PO4, 44 mmol/L HEPES, 13 mmol/L D-glucose. Incubations were performed in a 5% CO2 + 95% O2 atmosphere. The content of synaptosomal protein was determined according to the method of Lowry et al. [Citation15], using serum albumin as a standard. Isolated rat synaptosomes were exposed to free quercetin (10 µg/mL); empty particles (100 µg/mL) or quercetin-loaded particles (QR-NP1 and QR-NP2) (final quercetin concentration 10 µg/mL). After the pretreatment with the samples, 6-hydroxydopamnine (6-OHDA) (150 µmol/L) was used for induction of neurotoxicity.
Synaptosomal viability assay
Synaptosomal viability was determined by MTT-assay, as described by Mungarro-Menchaca et al. [Citation16]. After treatment with samples and 6-OHDA, synaptosomes were treated with MTT solution (0.5 mg/mL) for 1 h at 37 °C and were centrifuged at 15 000g for 1 min. The formed formazan crystals were dissolved in solubilizing solvent DMSO. The absorbance was measured spectrophotometrically at λ = 580 nm. The viability data were expressed as percentage of control value of untreated synaptosomes.
Glutathione (GSH) content determination
The levels of glutathione (GSH) were determined in rat brain synaptosomes with the Ellman's reagent (DTNB), which forms coloured complexes with –SH groups at pH = 8 with maximum absorbance at 412 nm [Citation17]. Statistical analysis was performed by the MEDCALC program. The results are expressed as mean values with standard error (±SEM) from 7 experiments. Three parallel samples were used. The significance of the data was assessed using the non-parametric Mann–Whitney test.
Data analysis
The results from the viability tests are expressed as means ±SD from at least three independent experiments. The cell survival data were normalized as percentage of the untreated control (set as 100% viability). The statistical analysis included the Student's t-test, whereby values of p < 0.05, p < 0.01 and p < 0.001 were considered statistically significant.
Results and discussion
Physicochemical characterization of nanoparticles
In the present study, two types of chitosan-alginate nanoparticles were prepared by variation of the concentrations of sodium alginate and chitosan. The different ratios of both biopolymers suggested eventual difference in the physicochemical properties of the resulting nanoparticles. presents the mean diameter and zeta potential of the two types of nanoparticles. As seen, the nanoparticles prepared with a higher concentration of chitosan (NP2) were of a larger size than those formulated with a higher concentration of alginate (NP1). The increase in nanoparticle size with an increase in chitosan concentration has been reported in previous studies [Citation18,Citation19]. This phenomenon could be due to protonation of primary amino groups of chitosan leading to repulsion between the chitosan chains and increase of the nanoparticle size [Citation20]. The nanoparticles prepared with a higher concentration of alginate possessed a negative zeta-potential, whereas those prepared with higher concentration of chitosan had a positive one. As stated before, the reason for differences in the zeta-potential is the different availability and neutralization of both the amino-groups of chitosan and the carboxyl groups of alginate depending on their initial concentration [Citation19].
Figure 1. Mean hydrodynamic diameter and zeta potential of the formulated chitosan-alginate nanoparticles: NP1, nanoparticles with higher concentration of alginate; NP2, nanoparticles with higher concentration of chitosan.
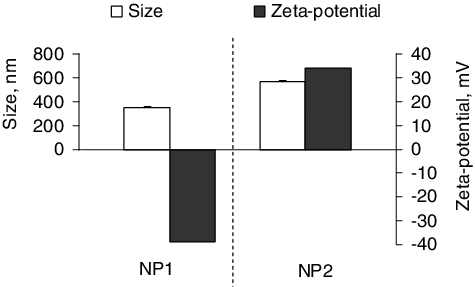
Successful encapsulation of quercetin was observed in both types of nanoparticles comparing their appearance to that of non-encapsulated quercetin in equimolar concentration ((A)). The ultraviolet–visible (UV-Vis) spectra of free and encapsulated quercetin showed absorption bands at 255 and 375 nm, which confirmed the presence of the drug in the nanoparticles (data not shown). The encapsulation efficiency ranged within 87% in QR-NP1 and 93% in QR-NP2 ((B)). Similar high encapsulation efficiency of approximately 96% has been reported for loading of quercetin in lecithin/chitosan nanoparticles [Citation21]. In our study, the high encapsulation efficiency of quercetin might be due to hydrogen bonding between its hydroxyl groups and the functional groups of both biopolymers. This is in agreement with studies reporting formation of hydrogen bonds when quercetin is loaded in polylactide-co-glycolide nanoparticles and liposomes [Citation22,Citation23].
Neuroprotective effects of free and encapsulated quercetin in H2O2-induced oxidative stress in neuroblastoma cells
Neuroblastoma SH-SY5Y cells were selected in this study, since their relevance for evaluation of the processes of neurotoxicity in neurodegenerative diseases has been well documented [Citation24,Citation25]. The study started with determination of the potential cytotoxic effects of free quercetin, encapsulated quercetin (QR-NP1 and QR-NP2) and empty nanoparticles (NP1 and NP2) on the cells after 24-h incubation. The results showed that all tested samples did not present any cytotoxic effect at the concentrations used in the study ((A)). Microscopic observations revealed that the monolayer integrity and cell morphology were preserved (data not shown). Thus, the results indicated the biocompatibility of free, encapsulated quercetin and empty nanoparticles with the selected cell line. These results were consistent with a study where quercetin (10 µmol/L) did not affect the cell viability of SH-SY5Y cells, as measured by MTT-assay and lactate dehydrogenase release [Citation26]. At low concentrations, quercetin exerted protection in a model of 6-OHDA toxicity in SH-SY5Y cells, which was related to the antioxidant and ROS-scavenging properties of quercetin.
Figure 3. Effect of free and encapsulated quercetin (10 µg/mL) on the viability of neuroblastoma SH-SY5Y cells (A) and SH-SY5Y viability in a model of H2O2-induced toxicity (B).
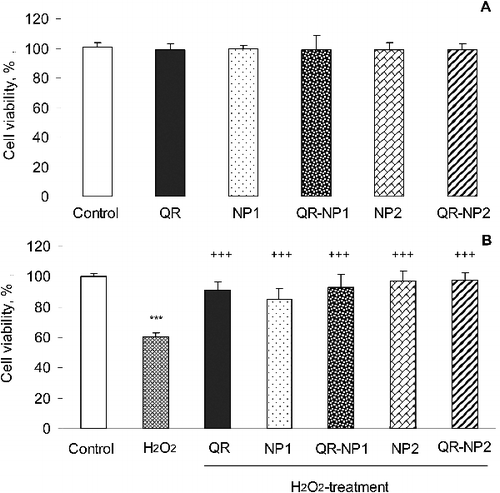
In our study, the neuroprotective effects were explored in a model of H2O2-induced oxidative stress in SH-SY5Y cells. H2O2 is commonly used as an inducer of oxidative stress in in vitro models. The mechanism of H2O2-induced cell damages includes production of reactive hydroxyl radicals and byproducts by Fenton's reaction that further interact directly with cellular components to damage proteins, lipids and DNA. These effects can presumably be blocked by antioxidants. In our study, the protection against H2O2-induced loss of cell viability was evaluated by pre-incubating the cells with free and encapsulated quercetin for 2 h, followed by treatment with 1 mmol/L H2O2 for 15 min. The cell viability was measured 24 h after the H2O2-induced damage. When SH-SY5Y cells were treated with H2O2, a statistically significant decrease (by 40%) in the cell viability was observed, compared to untreated controls ((B)), indicating significant cell damage. The pretreatment with free (QR, 10 µg/mL) and encapsulated quercetin (QR-NP1 and QR-NP2; quercetin concentration corresponding to 10 µg/mL) substantially reduced the H2O2-induced cell damage. A significant increase in the cell viability was observed (p < 0.001) particularly, by 31%, 33% and 37% for the three forms of quercetin, respectively, compared to H2O2 treatment. Thus, the protective effect of quercetin encapsulated into nanoparticles with a higher concentration of chitosan (QR-NP2) was superior. It should be noted that unloaded nanoparticles NP1 and NP2 also showed good protection towards oxidative stress damage. The morphology observations of the cells treated with both nanoparticle formulations also confirmed these results (). As seen, H2O2-treatment led to dramatic decrease in the number of viable cells ((B)), whereas encapsulated quercetin in both types of nanoparticles showed an increased number of viable cells ((C,D)). However, the protective effect was more evident with QR-NP2, which correlated with the results from the viability study ((B)).
Neuroprotective effects of free and encapsulated quercetin in a model of 6-OHDA neurotoxicity in rat brain synaptosomes
6-Hydroxidopamine (6-OHDA) is used as a model neurotoxin for modelling dopaminergic neurogeneration in neurogenerative disorders (i.e. Parkinson's disease). The mechanism of 6-OHDA toxicity involves selective uptake by plasma membrane dopamine transporters and subsequent mitochondrial accumulation [Citation27]. The neurotoxicity of 6-OHDA is mediated mainly by non-enzymatic oxidative stress and excessive generation of free reactive radicals [Citation28], so presumably it could be expected that oxidative lesions caused by 6-OHDA could be prevented by antioxidants.
The neuroprotective effect of quercetin encapsulated into both types of nanoparticles was studied in a model of 6-OHDA induced neurotoxicity in rat brain synaptosomes, which was previously adapted for evaluation of protective effects of curcumin loaded in polymeric microparticles [Citation29]. For the aim of this study, the synaptosomes were pretreated with empty nanoparticles, free quercetin and quercetin-loaded nanoparticles (concentration equivalent to 10 µg quercetin/mL) and the effects were explored by MTT-test after treatment with 6-OHDA ((A)). The results showed that the incubation of synaptosomes with 150 µmol/L 6-OHDA significantly reduced their viability by approximately 40%, which was in agreement with a previous study [Citation30]. Further, both types of empty nanoparticles (NP1 and NP2) demonstrated protective effects in a model of 6-OHDA-induced toxicity, showing an increase in synaptosomal viability by 9% and 30%, respectively, vs. 6-OHDA treatment. The pretreatment of brain synaptosomes with quercetin loaded into nanoparticles with a higher concentration of alginate (QR-NP1) increased the synaptosomal viability by 14% vs. 6-OHDA. In fact, this effect was similar to that of free quercetin. The pretreatment with quercetin loaded into the particles with the higher concentration of chitosan (QR-NP2) increased significantly the synaptosomal viability by 34% vs. 6-OHDA (p < 0.001). Thus, the effect of these nanoparticles was more pronounced, which correlated with their highest protective effect in H2O2-induced oxidative stress in SH-SY5Y cells ((B)).
Figure 5. Effect of free and encapsulated quercetin (10 µg/mL) on synaptosomal viability (A) and intra-synaptosomal total GSH level in a model of 6-OHDA-induced toxicity (B).
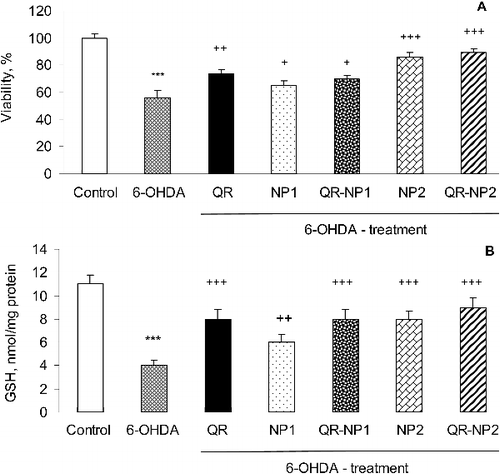
The content of GSH in rat brain synaptosomes was measured as an index of nonenzymatic antioxidant defence mechanisms. The treatment with 6-OHDA (150 µmol/L) evoked a significant decrease in GSH content by 64% (p < 0.001) vs. that in the untreated control ((B)). This effect was overcome by a pretreatment of synaptosomes with all formulations. Treatment with the samples, including free quercetin, empty and quercetin-loaded nanoparticles, demonstrated higher glutathione concentrations compared to that in the 6-OHDA group. However, the highest glutathione concentration (GSH increase by 123%, p < 0.001, vs. the 6-OHDA treated group) was observed when the pretreatment was performed with quercetin loaded in the particles with the higher concentration of chitosan (QR-NP2). Thus, the observed increase in the synaptosomal viability by pretreatment with encapsulated quercetin could be partially explained by its protective effects and/or stimulation of nonenzymatic antioxidant defence mechanisms, since an increased level of GSH was detected. The brain synaptosomes were, hence, better protected against oxidative stress induced by 6-OHDA. Other possible mechanisms of quercetin antioxidant activity include participation in intracellular signalling, inhibiting phosphatidylinositol-3 kinase, protein kinase C, xanthine oxidase and NADPH (nicotinamide adenine dinucleotide phosphate) diaphorase [Citation31,Citation32]. Nevertheless, it is assumed that the neuroprotective effects of quercetin are mainly explained by its antioxidant capacity and ability to scavenge free ROS generated from dopamine metabolism [Citation33–37].
The results from this study are in accordance with other in vitro and in vivo reports. For example, it has been reported that dietary quercetin suppresses the lipid peroxidation and increases the levels of GSH in rat brain tissue [Citation34]. Magalingam et al. [Citation38] reported that quercetin glycosides rutin and isoquercitrin significantly increased the activities of some antioxidant enzymes (catalase, superoxide dismutase and glutathione peroxidase) and the glutathione content, and were effective in suppressing lipid peroxidation in 6-OHDA induced PC-12 cells. The neuroprotective mechanism included quercetin-induced changes in the gene expression in a cellular model of Parkinson's disease [Citation39]. Quercetin has been shown to inhibit the ROS-generating activity of monoamine-oxidase A (MAO A) activity in mouse brain mitochondria [Citation40]. Haleagrahara et al. [Citation41] reported that systemic administration of quercetin protects striatal dopaminergic neurons in an in vivo model of 6-OHDA induced oxidative stress in rats. The neuroprotective role of quercetin against toxic agents, such as 1-methyl-4-phenyl pyridinium (MPP+), ammonium chloride and nocodazole was proposed by different mechanisms of protection, like apoptosis and autophagy [Citation42]. Summarizing, the results from the present study revealed an increase in the antioxidant effect of the quercetin-loaded nanoparticles against oxidative stress in two in vitro models of neuronal toxicity. In addition, the quercetin loaded in chitosan-based nanoformulations better protected against oxidative stress in both experimental models in vitro.
Conclusions
Quercetin was efficiently loaded in chitosan-alginate nanoparticles that were negatively or positively charged depending on the ratio between both biopolymers. The studies revealed that free and encapsulated quercetin provided significant protection against H2O2-induced oxidative stress damage in neuroblastoma SH-SY5Y cells and 6-OHDA induced neurotoxicity in rat brain synaptosomes. The highest protective effects were registered with quercetin loaded in the positively charged nanoparticles that were formulated with a higher concentration of chitosan. Thus, the incorporation of quercetin in nanoparticles based predominantly on chitosan might enhance the antioxidant effects of the drug].
Disclosure statement
The authors report no conflicts of interest. The authors alone are responsible for the content and writing of the article.
Additional information
Funding
References
- Fahn S, Cohen G. The oxidant stress hypothesis in Parkinson's disease: evidence supporting it. Ann Neurol. 1992;32(6):804–812.
- Mecocci P, MacGarvey U, Beal MF. Oxidative damage to mitochondrial DNA is increased in Alzheimer's disease. Ann Neurol. 1994;36(5):747–751.
- Butterfield DA, Reed T, Newman SF, et al. Roles of amyloid beta-peptide-associated oxidative stress and brain protein modifications in the pathogenesis of Alzheimer's disease and mild cognitive impairment. Free Radic Biol Med. 2007;43(5):658–677.
- Jenner P, Olanow CW. Oxidative stress and the pathogenesis of Parkinson's disease. Neurology. 1996;47(6 Suppl 3):161–170.
- Ishige K, Schubert D, Sagara Y. Flavonoids protect neuronal cells from oxidative stress by three distinct mechanisms. Free Radic Biol Med. 2001;30(4):433–446.
- Bastianetto S, Quirion R. Natural extracts as possible protective agents of brain aging. Neurobiol Aging. 2002;23(5):891–897.
- Singh A, Naidu PS, Kulkarni SK. Reversal of aging and chronic ethanol-induced cognitive dysfunction by quercetin a bioflavonoid. Free Radic Res. 2003;37(11):1245–1252.
- Ansari MA, Abdula HM, Joshia G, et al. Protective effect of quercetin in primary neurons against Aβ (1-42): relevance to Alzheimer's disease. J Nutr Biochem. 2009;20(4):269–275.
- Juurlink BH, Paterson PG. Review of oxidative stress in brain and spinal cord injury: suggestions for pharmacological and nutritional management strategies. J Spinal Cord Med. 1998;21(4):309–334.
- Dhawan S, Kapil R, Singh B. Formulation development and systematic optimization of solid lipid nanoparticles of quercetin for improved brain delivery. J Pharm Pharmacol. 2011;63(3):342–351.
- Ghosh A, Sarkar S, Mandal AK, et al. Neuroprotective role of nanoencapsulated quercetin in combating ischemia-reperfusion induced neuronal damage in young and aged rats. PLoS ONE. 2013 [cited 2017 Jun 21];8(4):e57735. DOI: 10.1371/journal.pone.0057735
- Rajaonarivony M, Vouthier C, Couarrze G, et al. Development of a new drug carrier made from alginate. J Pharm Sci. 1993;82(9):912–917.
- Mosmann T. Rapid colorimetric assay for cellular growth and survival: application to proliferation and cytotoxicity assays. J Immunol Methods. 1983;65(1–2):55–63.
- Taupin P, Zini S, Cesselin F, et al. Subcellular fractionation on Percoll gradient of mossy fiber synaptosomes: morphological and biochemical characterization in control and degranulated rat hippocampus. J Neurochem. 1994;62(4):1586–1595.
- Lowry OH, Rosebrough NJ, Farr AL, et al. Protein measurement with the Folin phenol reagent. J Biol Chem. 1951;193(1):265–275.
- Mungarro-Menchaca X, Ferrera P, Moran J, et al. Beta-Amyloid peptide induces ultrastructural changes in synaptosomes and potentiates mitochondrial dysfunction in the presence of ryanodine. J Neurosci Res. 2002;68(1):89–96.
- Robyt JF, Ackerman RJ, Chittenden CG. Reaction of protein disulfide groups with Ellman's reagent: a case study of the number of sulfhydryl and disulfide groups in Aspergillusoryzae – amylase, papain, and lysosome. Arch Biochem Biophys. 1971;147(1):262–269.
- Sarmento B, Ribeiro AJ, Veiga F, et al. Insulin-loaded nanoparticles are prepared by alginate ionotropic pre-gelation followed by chitosan polyelectrolyte complexation. J Nanosci Nanotechnol. 2007;7(8):2833–2841.
- Motwani SK, Chopra S, Talegaonkar S, et al. Chitosan–sodium alginate nanoparticles as |submicroscopic reservoirs for ocular delivery: formulation, optimisation and in vitro characterization. Eur J Pharm Biopharm. 2008;68(3):513–525.
- Aydin Tigli RST, Pulat M. 5-Fluorouracil encapsulated chitosan nanoparticles for pH-stimulated drug delivery: evaluation of controlled release kinetics. J Nanomater. 2012 [cited 2017 Jun 21];42:313961. DOI 10.1155/2012/31396
- Souza MP, Vaz AFM, Correia MTS, et al. Quercetin-loaded lecithin/chitosan nanoparticles for functional food applications. Food Bioprocess Technol. 2014;7(4):1149–1159.
- Pool H, Quintanar D, Figueroa JD, et al. Antioxidant effects of quercetin and catechin encapsulated into PLGA nanoparticles. J Nanomater. 2012; [cited 2017 Jun 21];86:145380. DOI: 10.1155/2012/313961
- Pawlikowska-Pawlęga B, Dziubińska H, Król E, et al. Characteristics of quercetin interactions with liposomal and vacuolar membranes. Biochim Biophys Acta. 2014;1838(1):254–265.
- Jämsä A, Hasslund K, Cowburn RF, et al. The retinoic acid and brain-derived neurotrophic factor differentiated SH-SY5Y cell line as a model for Alzheimer's disease-like tau phosphorylation. Biochem Biophys Res Commun. 2004;319(3):993–1000.
- Cheung Y-T, Lau WK, Yu MS, et al. Effects of all-trans-retinoic acid on human SH-SY5Y neuroblastoma as in vitro model in neurotoxicity research. Neurotoxicology. 2009;30(1):127–135.
- Kääriäinen TM, Piltone M, Ossola B, et al. Lack of robust protective effect of quercetin in two types of 6-hydroxydopamine-induced parkinsonian models in rats and dopaminergic cell cultures. Brain Res. 2008;1203:149–159.
- Glinka YY, Youdim, MB. Inhibition of mitochondrial complexes I and IV by 6-hydroxydopamine. Eur J Pharmacol Environ Toxicol Pharmacol. 1995;292(3–4):329–332.
- Schober A. Classic toxin-induced animal models of Parkinson's disease: 6-OHDA and MPTP. Cell Tiss Res. 2004;318(1):215–224.
- Yoncheva K, Kondeva-Burdina M, Tzankova V, et al. Curcumin delivery from poly(acrylic acid-co-methylmethacrylate) hollow microparticles prevents dopamine-induced toxicity in rat brain synaptosomes. Int J Pharm. 2015;486(1):259–267.
- Stokes AH, Freeman WM, Mitchell SG, et al. Induction of GADD45 and GADD153 in neuroblastoma cells by dopamine-induced toxicity. Neurotoxicology. 2002;23(6):675–684.
- Chang WS, Lee YJ, Lu FJ, et al. Inhibitory effects of flavonoids on xanthine oxidase. Anticancer Res. 1993;13(6A):2165–2170.
- Agullo G, Gamet-Payastre L, Manenti S, et al. Relationship between flavonoid structure and inhibition of phosphatidylinositol 3-kinase: a comparison with tyrosine kinase and protein kinase C inhibition. Biochem Pharmacol. 1997;53(11):1649–1657.
- Esposito E, Rotilio D, Di Matteo V, et al. A review of specific dietary antioxidants and the effects on biochemical mechanisms related to neurodegenerative processes. Neurobiol Aging. 2002;23(5):719–735.
- Mukai R, Kawabata K, Otsuka S, et al. Effect of quercetin and its glucuronide metabolite upon 6-hydorxydopamine-induced oxidative damage in Neuro-2a cells. Free Radic Res. 2012;46(8):1019–1028.
- Costa LG, Garrick, JM, Roquè, PJ, et al. Mechanisms of neuroprotection by quercetin: counteracting oxidative stress and more. Oxid Med Cell Longev. 2016; [cited 2017 Jun 21];2016:2986796. DOI: 10.1155/2016/2986796
- Dajas F, Abin-Carriquiry JA, Arredondo F, et al. Quercetin in brain diseases: potential and limits. Neurochem Int. 2015;89:140–148.
- Lakroun Z, Kebieche M, Lahouel A, et al. Oxidative stress and brain mitochondria swelling induced by endosulfan and protective role of quercetin in rat. Environ Sci Pollut Res. 2015;22(10):7776–7781.
- Magalingam KB, Radhakrishnan A, Haleagrahara N. Protective effects of quercetin glycosides, rutin, and isoquercetrin against 6-hydroxydopamine (6-OHDA)-induced neurotoxicity in rat pheochromocytoma (PC-12) cells. Int J Immunopathol Pharmacol. 2016;29(1):30–39.
- Magalingam KB, Radhakrishnan A, Ramdas P,. et al. Quercetin glycosides induced neuroprotection by changes in the gene expression in a cellular model of Parkinson's disease. J Mol Neurosci. 2015;55(3):609–617.
- Yoshino S, Hara A, Sakakibara H, et al. Effect of quercetin and glucuronide metabolites on the monoamine oxidase-A reaction in mouse brain mitochondria. Nutrition. 2011;27(7):847–852.
- Haleagrahara N, Siew CJ, Mitra NK, et al. Neuroprotective effect of bioflavonoid quercetin in 6-hydroxydopamine-induced oxidative stress biomarkers in the rat striatum. Neurosci Lett. 2011;500(2):139–143.
- Ahn TB, Jeon BS. The role of quercetin on the survival of neuron-like PC12 cells and the expression of α-synuclein. Neural Regener Res. 2015; [cited 2017 Jun 21];10(7):1113. DOI: 10.4103/1673-5374.160106.