ABSTRACT
A novel xylanase gene of family 10 (xyn27) was obtained from the genomic DNA of frozen soil of Daxinganling in China by Touch-down polymerase chain reaction (PCR) and thermal asymmetric interlaced (TAIL) PCR methods. Xyn27 contained a putative signal peptide (20 residues) and a catalytic motif of GH10 (327 residues) and shared the highest similarity (83%) with the reported GH10 xylanase (XM_003662144). The recombinant xyn27 was successfully expressed in Pichia pastoris GS115 and the optimal induction condition was 30 °C for 48 h. Xyn27 was demonstrated to be a cold-active xylanase, which shows its highest activity at 35 °C and still had 60.25%, 38.70% and 10.8% relative activity at 20 °C, 10 °C and 0 °C. Further analysis showed that Xyn27 has fewer arginine and more alanine residues compared with its mesophilic or thermophilic counterparts. The optimal pH of Xyn27 was 7.0, and it was stable after incubating under the pH range from 3.0 to 9.0 for 1 h. Besides, Xyn27 exhibited superior metal ion tolerance than other GH10 cold-active xylanases, being tolerant to most metal ions and organic solvents, and significantly enhanced by Ca2+, Mn2+ and Zn2+ metal ions. In addition, the Km, Vmax, kcat and kcat Km−1 against beechwood xylan were 13.42 mg mL−1, 9.07 μmol min−1 mg−1, 192.98 min−1 and 14.38 mL min−1 mg−1, and Xyn27 could completely degrade xylan into xylobiose. The features of cold activity and metal-ion tolerance suggested that xylanase Xyn27 could have potential application in basic research and various industries like food possessing.
Introduction
Hemicelluloses are the second most abundant plant polysaccharides in nature, which are attracting increasing attention due to their potential role as sustainable energy sources [Citation1]. The major component of hemicellulose is xylan, which is constituted of xylose units bound together through β-1,4-linkage and usually substituted with varying levels of L-arabinofuranosyl, galactosyl, acetyl, glucuronyl and 4-O-methylglucuronyl groups [Citation2]. Complete hydrolysis of xylan can only be achieved via the cooperative action of a battery of various enzymes because of its heterogeneity and complexity [Citation2]. Among them, endo-1,4-β-D-xylanase (EC 3.2.1.8) plays a crucial role in the hydrolysis of the xylan back-bone by cleaving the β-1,4-glycosidic bond between xylose residues to release short xylooligosaccharides and xylose [Citation1]. Based on the primary structure and amino-acid sequence, xylanases are classified into many glycoside hydrolase (GH) families, and xylanases belonging to GH10 and GH11 are mostly reported [Citation3]. Xylanases have been attracting extensive attention because they have potential applications in a wide range of industrial processes, including juice clarification, baking, brewing industry, dough processing, animal feed preparation, textile treatment and pulp bleaching [Citation4]. To avoid spoilage, nutritional value reduction and flavour change of the products, some industries such as juice extraction and dough handling prefer to perform under low temperature [Citation1,Citation5]. However, most xylanases characterized from fungi and bacteria show optimal temperature between 40 °C and 60 °C, and low temperature strongly inhibits the rates of enzyme-catalysed reactions. Interestingly, cold-active xylanases are an exception, as they are most active at low and intermediate temperature, and thermolabile at high temperature, the use of cold-active xylanases at the industrial level could not only avoid alterations in the ingredient and product quality, but will also reduce energy consumption and will avoid microbial development and fermentation [Citation1,Citation6]. To date, only several GH10 cold-active xylanases have been reported, including those from Paraglaciecola mesophila KMM241 [Citation7], Flavobacterium sp. MSY2 [Citation8], Bacillus sp. HJ2 [Citation9], Sphingobacterium sp. TN19 [Citation10], Penicillium chrysogen [Citation11] and environmental DNA of goat rumen contents [Citation12]. Hence, novel cold-active xylanases are still needed to be explored and studied. Psychrotrophic or psychrophilic environments are ideal resources for exploring cold-active enzymes, and some cold-active enzymes have been obtained from them [Citation7,Citation13]. Xylan degradation is a key step of the carbon cycle in the soil environment. Previous studies have also shown the diversity and presence of novel xylanase genes in different soil environments [Citation14]. So far, studies focusing on xylanases from the frozen soil in Daxinanling (China) remain limited; hence more bioprospecting studies in search of microbes producing cold-adapted xylanases merit scientific attention.
We have previously reported a novel cold-active GH43 xylosidase AX543 with optimal temperature of 25 °C from frozen soil in Daxinanling [Citation15]. The aim of the present study was to explore cold-active xylanases from frozen soil in Daxinanling. A novel GH10 family xylanase gene, xyn27, was cloned, expressed in Pichia pastoris and characterized. The recombinant xylanase Xyn27 showed excellent cold-active and chemical-resistant properties with great potential application in the food industry.
Materials and methods
Strains, plasmids and culture conditions
Escherichia coli DH5α and the vector PMD-19T (TaKaRa, Dalian, China) were used for gene cloning. P. pastoris GS115 and vector pPIC9 (Invitrogen, Carlsbad, CA, USA) were used for gene expression. Restriction enzymes, T4 DNA ligase and Fast Pfu DNA polymerase used for DNA manipulation were purchased from ThermoFisher Scientific (Shanghai, China). Yeast Nitrogen Base (YNB) with amino acids was purchased from Solarbio (Beijing, China). E. coli cells were grown in Luria–Bertani (LB) medium (teast extact 5 g L−1, peptone 10 g L−1, NaCl 10 g L−1) at 37 °C on a rotary shaker at 220 r min−1. Yeast extract peptone dextrose (YPD) medium and buffered glycerol-complex (BMGY) medium used for P. pastoris growth, and buffered methanol-complex (BMMY) used for gene expression were prepared according to the standard procedure described in the Pichia expression kit (Invitrogen, Carlsbad, USA). All other chemicals/reagents used were of analytical grade.
Metagenomic DNA extraction and gene cloning and analysis of Xyn27
Genomic DNA was extracted from frozen soil by the cetyltrimethylammonium bromide (CTAB)–sodium dodecyl sulfate (SDS) method according to Brady et al. [Citation16] The crude DNA was purified by a DNA purification kit (Solarbio, Beijing, China) and was detected by gel electrophoresis. Using the genomic DNA of frozen soil as a template and the primers in , the xylanase gene xyn27 was cloned by Touch-down polymerase chain reaction (PCR) and thermal asymmetric interlaced (TAIL) PCR [Citation17,Citation18], and then sequenced by Biomed (Beijing, China). The open reading frame (ORF) was analyzed by Vector NTI 7.0 (Informax Inc., Bethesda, Md., USA). The full-length nucleotide sequence was further aligned by BlastX and BlastP (http://blast.ncbi.nlm.nih.gov/Blast.cgi). The signal peptide, glycosylation sites and the intron were predicted according to Teng et al. [Citation19] and the multiple-protein-sequence alignment with other GH10 xylanases, including XynA from Humicola insolens (AGG68962), XynC01 from Achaetomium sp. Xz-8 (AHE13927), Xyl10A from Talaromyces cellulolyticus (GAM37231) and 10b from Thermotoga maritima (1VBU), was carried out using the ClustalW (http://www.ebi.ac.uk/clustalW).
Table 1. Primers used in this study.
Heterologous expression and purification of Xyn27
The full-length gene sequence of xyn27 was digested with EcoRI and NotI, ligated with a pPIC9 vector and transformed into E. coli DH5α. The correct recombinant plasmid verified by restriction digestion was then linearized by PmeI and transformed into P. pastoris GS115 competent cells by electroporation. The transformants were screened and induced by methanol according to the manufacturer's protocol (Invitrogen, Carlsbad, USA), and were subjected to the enzyme activity assay [Citation20]. The positive transformants with the highest xylanase activity were incubated in BMGY medium at 30 °C for 48 h with constant shaking (220 r min−1) and then cultured in BMMY medium at 30 °C for protein induction. For continuous expression, methanol was added every 24 h in order to constantly keep the final methanol concentration at 0.5%. The recombinant protein Xyn27 was purified by nickel affinity chromatography (GE Health-care, Uppsala, Sweden) with 10–500 mmol L−1 imidazole in buffer [Citation20] (20 mmol L−1 Tris-HCl, 50 mmol L−1 NaCl, pH 8.0). The protein fractions corresponding to different imidazole concentrations were collected and subjected to sodium dodecyl sulfate (SDS)-polyacrylamide gel electrophoresis (PAGE; 12% separation gel and 5% spacer gel), and enzyme activity assay. The protein concentration was determined using a Bradford protein assay kit (BioRad, Shanghai, China). Besides, in order to increase the expression level of recombinant proteins in P. pastoris, the growth and induction conditions such as time (12, 24, 36, 48, 60 and 72 h) and temperature (20, 25, 30 °C) was optimized. The experiments were carried out three times, and each experiment was performed in triplicate.
Enzyme activity assays and biochemical characterization
Enzymatic activity was measured by colorimetric assay using beechwood xylan as substrate. The reaction mixture (0.9 mL substrate (1% w/v) in 100 mmol L−1 sodium acetate buffer, pH 7.0, and 0.1 mL enzyme solution) was incubated at 35 °C for 10 min. The reaction was stopped by adding 1.5 mL of 3,5-dinitrosalicylic acid (DNS) and boiling for 5 min. The quantification of the reducing sugars released as a result of enzyme activity was done by measurements at 540 nm (A540) [Citation21]. One unit of xylanase activity was defined as the amount of protein that released 1 μmol of xylose per minute at 35 °C and pH 7.0.
The optimum temperature for Xyn27 activity was determined at different temperatures (10, 20, 30, 35, 40, 50, 65 and 80 °C) at pH 7.0. The thermal stability of the enzyme was determined by measurement of the residual enzyme activity after pre-incubation at 35, 40 and 45 °C without substrate for various time periods. The optimum pH for enzyme activity was measured at the optimum temperature under 0.2 mol L−1 citric acid-Na2HPO4 buffer (pH 4.0, 5.0, 6.0, 7.0 and 8.0). The pH stability was investigated by measurement of the residual enzyme activity at optimum conditions after pre-incubation in various buffers with pH (3.0, 4.0, 5.0, 6.0, 7.0, 8.0 and 9.0) at 35 °C for 1 h without substrate. The purified xylanases were incubated in 5 mmol L−1 solution of MgCl2, KCl, LiCl, MnCl2, CuCl2, ZnCl2, FeCl3, CaCl2, NiCl2, ethylenediaminetetraacetic acid (EDTA) and SDS for 30 min at room temperature in sodium citrate buffer (0.1 mol L−1, pH 7.0). The residual enzyme activity was determined under the standard conditions using beechwood xylan as a substrate. The reaction system without any additive was used as a control. The experiments were carried out three times, and each experiment included triplicate measurements.
Determination of kinetic parameters
According the DNS assay, the specific activity of xylanse Xyn27 was assayed using 1% beechwood xylan in 100 mmol L−1 sodium citric acid buffer (pH 7.0) at 50 °C for 10 min. The reaction was stopped by addition of 1.5 mL DNS reagent and the absorbance was measured at 540 nm. The kinetic parameters (Km and Vmax) were determined for recombinant Xyn27 at 35 °C for 10 min in 100 mmol L−1 sodium citric acid buffer (pH 7.0) using beechwood xylan in the range of 0.1, 0.5, 1.0, 2.0, 5.0 and 10.0 mg mL−1. A Lineweaver–Burk plot was used for kinetic parameter determination.
Analysis of enzymatic products
The hydrolytic properties of Xyn27 against beechwood xylan were determined by incubating 5 U purified Xyn27 and 0.5 mL beechwood xylan in citric acid-Na2HPO4 buffer at 35 °C for 24 h. The final enzymatic products were analyzed by the Waters High Performance Liquid Chromatography (HPLC) apparatus equipped with an Aminex HPX-87H Column (300 × 7.8 mm) and Waters 2414 Refractive Index Detector. The flow phase is 5 mmol L−1 H2SO4 with a flow rate of 0.6 mL per minute. The temperature of the column was 63 °C, and the detection was performed at 45 °C. Xylose, xylobise, xylotriose, xylotetraose and xylopentose were used as standards.
Nucleotide sequence accession number
The nucleotide sequence of xyn27 is available in GenBank (https://www.ncbi.nlm.nih.gov/genbank/) under Gene ID of KY352354.
Results and discussion
Cloning and sequence analysis of the xylanse gene xyn27
A partial gene fragment of 250 bp was amplified by Touch-down PCR, and was cloned into pMD-19T for sequencing. The flanking region was amplified with TAIL-PCR using the nested internal primers which were designed based on the 250 bp core region. The complete ORF of xyn27 consisted of 1101 bp encoding 346 amino-acid residues with a calculated molecular mass of 40.41 kDa. Xyn27 was predicted to have a signal peptide with the first 20 amino acids, but not an N-glycosylation site. BlastP search indicated that Xyn27 belongs to GH family 10 and its amino acid sequence showed the highest similarity (83%) to the reported GH10 xylanase (XM_003662144) from Myceliophthora thermophila ATCC 42464.
Expression and purification of recombinant enzyme
The full-length sequence of gene xyn27 was cloned into pPIC9 to obtain the recombinant plasmid pPIC9-xyn27. Through enzyme activity screening using substrate of 1% (w/v) beechwood xylan, the positive transformant with the highest activity was selected and further fermented in a 1-L flask. After induction by 0.5% methanol for different durations, the highest activity of Xyn27 was reached at 48 h (). The recombinant protein Xyn27 was purified to homogeneity as shown in . It was a little larger than the calculated molecular mass, which might be attributed to the His6-tag. Due to the importance of time and temperature for protein production in the P. pastoris expression system [Citation22], a study on the effects of these factors in Xyn27 production was performed. The results showed that the optimal induction temperature was 30 °C, and the activity reached the highest point after 48 h induction at optimum conditions (pH 7.0, 30 °C and 0.5% methanol concentration).
Figure 1. Optimization of time (A,B) and temperature (C,D) for the expression of Xyn27. (A) SDS-PAGE analysis with different induction time: (M) protein marker (Thermo Fisher Scientific, Shanghai, China), (1) 12 h, (2) 24 h, (3) 36 h, (4) 48 h, (5) 60 h, (6) 72 h; (B) enzyme activity assays under different induction time. (C) SDS-PAGE analysis with different induction temperature: (M) protein marker, (1) 20 °C, (2) 25 °C, (3) 30 °C; (D) enzyme activity assays under different induction temperatures. Note: The highest activity at 60 h was used as 100% (B). The experiments were carried out three times, and each experiment included triplicates. Standard errors of the means (±SEM) were calculated from three independent experiments.
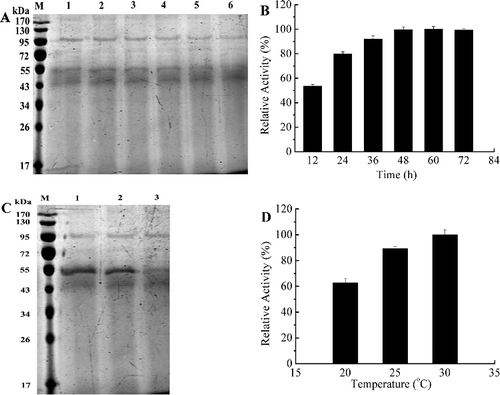
Effects of pH and temperature on the activity of recombinant Xyn27
The pH activity profile of Xyn27 was determined in different buffers (pH 4.0–9.0). From the pH profile, the optimal pH for Xyn27 activity was 7.0, and the relative activity remained above 50% from pH 4.0 to pH 6.0 ((A)). When preincubated in different pH buffers for 60 min at 35 °C, Xyn27 retained about 36% maximal activity over the pH range from pH 3.0 to pH 9.0 ((C)). The enzyme showed xylanase activity in the temperature range between 0 °C and 80 °C, with an optimum at 35 °C ((B)), and still had 60.25%, 38.70% and 10.8% relative acitivity at 20, 10 and 0 °C, respectively. The purified Xyn27 enzyme was thermolabile but retained about 70% of its initial activity after 60 min incubation at 35 °C ((D)). Many microorganisms isolated from mesophilic or psychrophilic environments have been described as mesophilic or psychrophilic, and diverse cold-active enzymes have been reported from them [Citation12]. The frozen soil of Daxinganling is a typical cold environment, and recently we have identified a cold-active xylosidase AX543 from an Acremonium sp. strain isolated from frozen soil in Daxinganling [Citation15]. These results from the study corroborate previous reports on psychrotrophic or psychrophilic environment or microorganisms being good sources for discovering cold-active enzymes.
Figure 3. Ezymatic characterization of recombinant Xyn27. Effect of pH (A) on xylanase activity at 35 °C; effect of temperature (B) on xylanase activity at pH 7.0; pH stability (C) at 37 °C for 1 h in buffers of pH 3.0 to 10.0; thermostability (D) at 45 °C, 55 °C and 60 °C. Note: The enzyme activity at pH 7.0 (A), at 35 °C (B) or without any treatment (C,D) was taken as 100%. The experiments were carried out three times, and each experiment included triplicates. Standard errors of the means (±SEM) were calculated from three independent experiments.
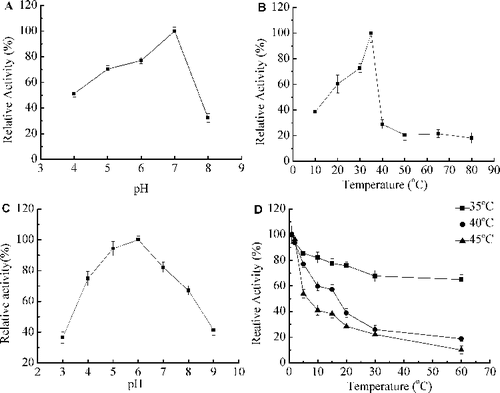
In this study, we focused on mining the cold-active xylanases from frozen soil in Daxinanling, and a novel cold-active xylanase-encoding gene, xyn27, was obtained and expressed in P. pastoris. Xyn27 was predicted to have low similarity (<34.9%) to other cold-active xylanases, which indicated that Xyn27 is a novel GH10 cold-active xylanase.
Similar to most other cold-active enzymes, cold-active xylanases share the common features of low temperature optimum, high catalytic activities at low temperatures and poor stability [Citation1]. Among these reported cold-active xylanases, xylanases A and B from Antarctic krill (Euphausia superba Dana) have the highest optimum temperature (37–40 °C) [Citation23] and Xyn8 from an environmental DNA library, the lowest optimum temperature (20 °C) [Citation8]. Xyn27 in the present study showed optimal activity at 35 °C, which is the same as xylanases XynAHJ2 from Bacillus sp. HJ2, and a little higher than most other cold-active xylanases (30 °C), such as XynA from Glaciecola mesophila KMM241 [Citation7], Xyn10 from Flavobacterium sp. MSY2 [Citation8] and XynGR40 from environmental DNA of goat rumen contents [Citation12]. Xyn27 was also verified to be thermolabile: it retained 18.91% relative activity after 60 min at 40 °C and only 1.8% relative activity after 1 min at 45 °C. These results clearly indicated that r-Xyn27 displays the typical features of cold-active xylanases, and thus is defined as a cold-active xylanase. To the best of our knowledge, this is the first report of a cold-active xylanase from frozen soil in Daxinanling.
Compared with mesophilic or thermophilic counterparts, the high catalytic efficiency of cold-active enzymes at low temperature might be correlated with their highly flexible structures, which allows for molecular motions necessary for activity at low temperatures, and consequently results in the thermolability of cold-active enzymes [Citation24]. Several structural features of cold-active enzymes have been observed, including, but not limited to, more glycine residues, more small amino acids, fewer proline residues, a lower arginine/lysine ratio, and a reduction of salt bridges, aromatic interactions, hydrogen bonds and/or disulphide bridges [Citation6]. Thus, the amino-acid composition and putative structure of Xyn27 and other GH10 cold-active xylanases were further analyzed, and Xyn27 was shown to contain fewer arginine (3.17%) and more alanine residues (11.82%) compared to its mesophilic and thermophilic counterparts ( and ). Previous studies have also reported that cold-active xylanase XynHJ2 has fewer arginine redidues and salt bridges [Citation25]; cold-active xylanase XynA from Sorangium cellulosum So9733-1 has fewer salt bridges and hydrogen bonds [Citation26]; and cold-active xylanase XynGR40 from the metagenomic DNA of rumen content has fewer hydrogen bonds and salt bridges, and lengthened loops in the catalytic domain [Citation12].
Figure 4. Sequence alignment of Xyn27 with other mesophilic and thermophilic GH10 xylanases. Note: Identical residues are shaded in black; conserved residues are shaded in gray; putative catalytic residues are marked by a triangle. The dotted-line frames indicate the designed degenerate primers sequence. The boldface A, G, R, K are the putative amino-acid sites responsible for the cold-active property of Xyn27.
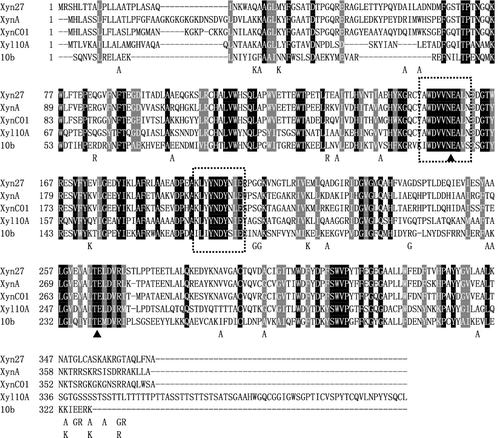
Table 2. Factors affecting the stability and flexibility of Xyn27 compared with other mesophilic or thermophilic counterparts.
Effects of metal ions and chemical compounds on the activity of Xyn27
The xylanase activity of Xyn27 in the presence of 5 mmol L−1 of different metal ions or chemical reagents is shown in . The activity of Xyn27 was strongly enhanced by 23.33%, 29.13% and 6.45% in the presence of Mn2+, Ca2+ or Zn2+, respectively. Besides, Ni2+, Fe2+, Cu2+ and Fe3+ only slightly inhibited the activity of Xyn27 (94.28%, 89.98%, 85.50% and 70.93%, respectively). The residual activity of Xyn27 was 93.23% and 83.07% in the presence of EDTA and SDS, respectively. However, previous studies have shown that heavy metal ions, such as Cu2+, Mn2+ and Co2+, could thus strongly inhibit the xylanase activity, including that of the reported cold-active xylanases, because of binding irreversibly with the main polypeptide or side chains. For example, XynA from Sorangium cellulosum So9733 only retained 5.8%, 26.4% and 18.6% relative activity in the presence of 1 mmol L−1 Cu2+, Zn2+ and Ni2+, respectively [Citation26], and XynA from Glaciecola mesophila KMM 241 exhibited 11.4% and 76.2% relative activity under 1 mmol L−1 of SDS and Mn2+, respectively [Citation14]. Hence, compared with these reported cold-active xylanases, Xyn27 showed superior tolerance to most of the tested metal ions and reagents.
Determination of specific activity and kinetic parameters of recombinant Xyn27
The specific activity of Xyn27 was 10.41 U mg−1 at 35 °C and pH 7.0. In order to determine the kinetic parameters of Xyn27, beechwood xylan substrate was used over a concentration range from 5.0 to 10 mg mL−1 in 100 mmol L−1 sodium acetate buffer, pH 7.0, and the activities were determined under standard conditions. The Km, Vmax, kcat and kcat Km−1 of the recombinant Xyn27 against beechwood xylan were 13.42 mg mL−1, 9.07 μmol min−1 mg−1, 192.98 min−1 and 14.38 mL·min−1 mg−1, respectively. The catalytic efficiency of Xyn27 was higher than that of cold-active xylanase XynA from Sorangium cellulosum So9733-1, but lower than those of other cold-active xylanases as shown in .
Table 3. Kinetic parameters of recombinant Xyn27 and other cold-active xylanases.
Analysis of enzymatic products
The enzymatic products of beechwood xylan degradation by Xyn27 were analyzed by the HPLC technique (). As shown in , the main enzymatic product from beechwood was xylobiose after incubation at 35 °C for 24 h, which suggests the xylan was degraded completely by Xyn27. Thus, the recombinant Xyn27, with its cold-active properties and superior tolerance to a range of metal ions and reagents, could be considered a promising candidate for use in food industry or other industries under harsh conditions such as metal ion contamination.
Conclusions
A novel GH family 10 xylanase Xyn27 from frozen soil genomic DNA was cloned and successfully expressed in P. pastoris GS115. The deduced protein showed low similarity to known fungal xylanases (83%). Xyn27 is a cold-active xylanase which shows its highest activity around 35 °C and pH 7.0, and retains about 70% of its initial activity after 60 min incubation at 35 °C, and 70% maximal activity over the pH range from 3.0 to 9.0. The xylanase activity remained unaffected in the presence of most metal ions tested and EDTA and SDS. These properties make Xyn27 a good candidate for use in food industry and other industries, particularly under conditions of metal ion contamination.
Disclosure statement
The authors declare no conflict of interest.
Additional information
Funding
References
- Collins T, Gerday C, Feller G. Xylanases, xylanase families and extremophilic xylanases. FEMS Microbiol Rev. 2005;29(1):3–23.
- Bastawde KB. Xylan structure, microbial xylanases, and their mode of action. World J Microbiol Biotechnol. 1992;8:353–368.
- Finn RD, Bateman A, Clements J, et al. Pfam: the protein families database. Nucleic Acids Res. 2014;42(Database issue):D222–D230.
- Khandeparker R, Numan MT. Bifunctional xylanases and their potential use in biotechnology. J Ind Microbiol Biotechnol. 2008;35(7):635–644.
- Georlette D, Bentahir M, Claverie P, et al. Cold adapted enzymes. Trends Biotechnol. 2001;15(9):177–196.
- Sarmiento F, Peralta R, Blamey JM. Cold and hot extremozymes: industrial relevance and current trends. Front Bioeng Biotechnol. 2015;3:148.
- Guo B, Li PY, Yue YS, et al. Gene cloning, expression and characterization of a novel xylanase from the marine bacterium, Glaciecola mesophila KMM241. Mar Drugs. 2013;11(4):1173–1187.
- Lee CC, Smith M, Kibblewhite-Accinelli RE, et al. Isolation and characterization of a cold-active xylanase enzyme from Flavobacterium sp. Curr Microbiol. 2006;52(2):112–116.
- Zhou J. Molecular and biochemical characterization of a novel intracellular low-temperature-active xylanase. J Microbiol Biotechnol. 2012;22(4):501–509.
- Zhou J, Huang H, Meng K, et al. Molecular and biochemical characterization of a novel xylanase from the symbiotic Sphingobacterium sp. TN19. Appl Microbiol Biotechnol. 2009;85(2):323–333.
- Hou YH, Wang TH, Long H, et al. Novel cold-adaptive Penicillium strain FS010 secreting thermo-labile xylanase isolated from Yellow Sea. Acta Biochim Biophys Sin. 2006;38(2):142–149.
- Wang G, Luo H, Wang Y, et al. A novel cold-active xylanase gene from the environmental DNA of goat rumen contents: direct cloning, expression and enzyme characterization. Bioresour Technol. 2011;102(3):3330–3306.
- Petrescu I, Lamottebrasseur J, Chessa JP, et al. Xylanase from the psychrophilic yeast Cryptococcus adeliae. Extremophiles. 2000;4(3):137–144.
- Guo B, Chen XL, Sun CY, et al. Gene cloning, expression and characterization of a new cold-active and salt-tolerant endo-beta-1, 4-xylanase from marine Glaciecola mesophila KMM 241. Appl Microbiol Biotechnol. 2009;84(6):1107–1115.
- Zhang MH, Li ZY, Qiu HY, et al. Cloning and characterization of a novel cold-active bifunctional xylosidase/arabinfuranosidease AX543. Biotechnol Bull. 2016;32(11):215–223.
- Brady SF. Construction of soil environmental DNA cosmid libraries and screening for clones that produce biologically active small molecules. Nat Protoc. 2007;2(5):1297–1305.
- Wang G, Meng K, Luo H, et al. Phylogenetic diversity and environment-specific distributions of glycosyl hydrolase family 10 xylanases in geographically distant soils. PLoS One. 2012;7(8):e43480.
- Li Z, Xue X, Zhao H, et al. A C-terminal proline-rich sequence simultaneously broadens the optimal temperature and pH ranges and improves the catalytic efficiency of glycosyl hydrolase family 10 ruminal xylanases. Appl Environ Microbiol. 2014;80(11):3426–3432.
- Teng C, Jia H, Yan Q, et al. High-level expression of extracellular secretion of a beta-xylosidase gene from Paecilomyces thermophila in Escherichia coli. Bioresour Technol. 2011;102(2):1822–1830.
- Tseng MJ, Yap MN, Ratanakhanokchai K, et al. Purification and characterization of two cellulase free xylanases from an alkaliphilic Bacillus firmus. Enzyme Microb Technol. 2002;30(5):590–595.
- Berrin JG, Juge N. Factors affecting xylanase functionality in the degradation of arabinoxylans. Biotechnol Lett. 2008;30(7):1139–1150.
- Goncalves AM. Pichia pastoris: a recombinant microfactory for antibodies and human membrane proteins. J Microbiol Biotechnol. 2013;23(5):587–601.
- Woodward AM, Senchyna M, Williams R, et al. Characterization of the interaction between hydroxypropyl guar galactomannan and galectin-3. Biochem Biophys Res Commun. 2012;424(1):12–17.
- Spiwok V, Lipovová P, Skálová T, et al. Cold-active enzymes studied by comparative molecular dynamics simulation. J Mol Model. 2007;13(4):485–497.
- Zhou J, Dong Y, Tang X, et al. Molecular and biochemical characterization of a novel intracellular low-temperature-active xylanase. J Microbiol Biotechnol. 2012;22(4):501–509.
- Wang SY, Hu W, Lin XY, et al. A novel cold-active xylanase from the cellulolytic myxobacterium Sorangium cellulosum So9733-1: gene cloning, expression, and enzymatic characterization. Appl Microbiol Biotechnol. 2011;93(4):1503–1512.
- Gallardo Ó, Javier Pastor FI, Polaina J, et al. Structural insights into the specificity of Xyn10B from Paenibacillus barcinonensis and its improved stability by forced protein evolution. J Biol Chem. 2010;285(4):2721–2733.
- Du Y, Shi P, Huang H, et al. Characterization of three novel thermophilic xylanases from Humicola insolens Y1 with application potentials in the brewing industry. Bioresour Technol. 2013;130:161–167.
- Zhao L, Meng K, Shi P, et al. A novel thermophilic xylanase from Achaetomium sp. Xz–8 with high catalytic efficiency and application potentials in the brewing and other industries. Process Biochem. 2013;48(12):1879–1885.
- Ihsanawati, Kumasaka T, Kaneko T, et al. Structural basis of the substrate subsite and the highly thermal stability of xylanase 10B from Thermotoga maritima MSB8. Proteins. 2005;61(4):999–1009.
- Wang GZ, Wang YR, Yang PL, et al. Molecular detection and diversity of xylanase genes in alpine tundra soil. Appl Microbiol Biotechnol. 2009;87:1383–1393.
- Liu XS, Huang ZQ, Zhang XN, et al. Cloning, expression and characterization of a novel cold-active and halophilic xylanase from Zunongwangia profunda. Extremophiles. 2014;18:441–450.