ABSTRACT
Catalase (CAT) is a key scavenging enzyme for the degradation of hydrogen peroxide (H2O2) and plays an important role in the tolerance to diverse abiotic stresses in many different organisms. In this study, we characterized the function of a catalase gene (CsCAT3) previously isolated from Cucumis sativus in the defence against a variety of stresses. Protein alignment and phylogenetic analysis revealed that CsCAT3 was clustered in the dicot group and shared 41%–95% identity with other plant CATs. Expression analyses revealed that the expression of CsCAT3 was induced by diverse abiotic stresses such as heat, polyethylene glycol, cold and NaCl treatment, as well as by signalling molecules such as abscisic acid (ABA) and H2O2. An Escherichia coli heterologous expression system was constructed to characterize the function of CsCAT3 in vitro. Its overexpression in E. coli could increase the tolerance to heat, cold, salinity and osmotic conditions. These results indicate that CsCAT3 plays important roles in abiotic stress tolerance and that CsCAT3 confers tolerance in E. coli recombinants against abiotic stresses, which may be due to the increased activities of antioxidant enzymes that reduce the oxidative damage caused by stress conditions.
Introduction
Plants are frequently exposed to various stresses under natural conditions, resulting in the production of reactive oxygen species (ROS), such as the superoxide ion (O2−), hydrogen peroxide (H2O2), hydroxyl radical (OH−), peroxyl radical (HOO−) and ozone (O3) [Citation1]. Excessive production of ROS can result in oxidative stress and can induce oxidative damage to cellular components [Citation2], which adversely affects the growth and productivity of plants. To cope with oxidative stress, plants have developed various antioxidant mechanisms, including ROS-scavenging enzymes such as ascorbate peroxidase (APX), catalase (CAT), superoxide dismutase (SOD), glutathione peroxidase (GPX), as well as non-enzymatic components such as glutathione, ascorbate and carotenoids [Citation3].
As one of the main antioxidant enzymes, CAT (EC 1.11.1.6) serves as an efficient scavenger of ROS and can catalyse the dismutation of two molecules of hydrogen peroxide (H2O2) into oxygen (O2) and water. Genome-wide studies have demonstrated that CAT is encoded by a small gene family which has been reported in many plant species, including Nicotiana plumbaginifolia [Citation4], Hordeum vulgare [Citation5], Zea mays [Citation6], Arabidopsis thaliana [Citation7,Citation8], Solanum lycopersicum [Citation9], Capsicum annuum [Citation10], Oryza sativa [Citation11,Citation12], Saccharum officinarum [Citation13,Citation14 ], and Cucumis sativus [Citation15].
Previous studies have revealed that the expression of plant catalase genes is induced by diverse abiotic stresses and a variety of environmental stimuli [Citation7,Citation8,Citation13,Citation16–18]. In hot pepper, the transcripts of CaCat1 are induced in response to aluminium, NaCl and light treatment [Citation19]. In Arabidopsis, AtCAT2 is activated by cold and drought stress and AtCAT3 is mainly enhanced by abscisic acid (ABA) and oxidative treatments [Citation8]. In ginseng, the PgCat1 gene is enhanced at different levels in response to various stresses, including heavy metals, osmotic agents, plant hormones and high light irradiances [Citation16]. In banana, the transcripts of MaCat2 are increased by cold treatment and physical damage [Citation20]. In sugarcane, the expression of ScCAT1 can be induced by different kinds of stresses, including S. scitamineum challenge, plant hormone [salicylic acid (SA), methyl jasmonate (MeJA), and ABA] treatments, oxidative (H2O2) stress, heavy metal (Cu2+) and hyper-osmotic (polyethyele glycol (PEG) and NaCl) stress [Citation21]. Very recently, two catalase genes were isolated and analyzed in Erianthus arundinaceus and Saccharum officinarum. The expression of these genes, EaCAT-1b and SoCAT-1c, is up- and down-regulated under drought stress, respectively [Citation13].
The catalase genes exhibit different patterns in response to various abiotic stresses, suggesting that catalase may help to protect the plant against oxidative stress [Citation21]. Hence, different attempts have been made to overexpress catalase genes in plants with the aim to increase plant stress resistance. For example, introduction of KatE from E. coli into tobacco and rice resulted in high tolerance to drought and salinity in the crops, respectively [Citation22–24]. Transgenic Arabidopsis containing the broccoli catalase gene BoCAT showed heat tolerance by removing the H2O2 accumulated due to high temperature [Citation25].
Cucumber (Cucumis sativus) is a major vegetable crop consumed worldwide and exhibits a higher transpirational rate and sensitivity to diverse abiotic stresses. Therefore, it is necessary to study the molecular mechanism of stress-related genes and enhance the stress-resistance of cucumber through genetic manipulation. In our previous study, genome-wide gene identification, phylogenetic analysis and expression analysis of CsCAT genes in cucumber were conducted [Citation15]. However, the physiological roles of cucumber CAT genes in response to various stresses remain intricate. In this study, we further investigated the biological function of a cucumber CAT gene, CsCAT3, in greater depth. Our results revealed that the transcription of CsCAT3 can be induced by diverse abiotic stresses such as heat, PEG, cold and NaCl, as well as by signalling molecules such as ABA and H2O2, and overexpression of CsCAT3 in E.coli could confer the tolerance to abiotic stresses including heat, cold, salinity and osmotic conditions. We speculated that CsCAT3 may be involved in the response to various abiotic stress and could play key roles in these processes.
Materials and methods
Plant materials and stress treatments
Cucumis sativus L. cv. Chinese long No. 9930 is used in modern cucumber breeding and the culture practices were described in our previous paper [Citation15]. Two-week-old cucumber seedlings were selected and subjected to different abiotic stresses. For salt, ABA, H2O2 and PEG treatments, cucumber seedlings were grown in liquid Murashige and Skoog (MS) medium containing 200 mmol/L NaCl, 100 μmol/L ABA and 10 mmol/L H2O2 or 300 mmol/L PEG-6000, respectively. For cold and heat treatment, cucumber seedlings in the growth chamber were transferred to 4 and 50 °C under light conditions. Leaf samples were collected at 0, 3, 6, 12 and 24 h, and then immediately frozen in liquid nitrogen and stored at −80 °C until RNA extraction.
Cloning of CsCAT3 gene
CsCAT3 gene was amplified from cDNA templates synthesized from RNA mixtures extracted from cucumber leaves based on the sequence of CsCAT3 (Gene ID: Csa013194) in the cucumber database (http://cucumber.genomics.org.cn). The PCR product was cloned to pGEM-T vector (Promega, USA) following the product manual. After plasmid DNA extraction, the positive final clones were used for sequencing to confirm the CsCAT3 gene (Sangon, China). The primers are listed in Table 1S (Online Supplementary Appendix).
Sequence analysis, multiple sequence alignment and phylogenetic analysis
The theoretical pI, molecular weight (MW) and the grand average of hydropathicity (GRAVY) of the putative protein were determined by the ProtParam software (http://web.expasy.org/protparam/). The catalytic active site and heme-binding site of CsCAT3 were detected by NCBI Conserved Domain Database (https://www.ncbi.nlm.nih.gov/Structure/cdd/wrpsb.cgi), Motif Scan (http://myhits.isb-sib.ch/cgi-bin/motif_scan) and InterPro (http://www.ebi.ac.uk/interpro/). Subcellular localization was predicted using ProtComp (http://www.softberry.com/), CELLO (http://cello.life.nctu.edu.tw/) and Plant-mPLoc (http://www.csbio.sjtu.edu.cn/bioinf/plant-multi/). The three-dimensional (3D) structure of CsCAT3 was predicted using the protein sequences on SWISS-MODEL (http://swissmodel.expasy.org).
Sequence comparison and multiple sequence alignment were performed using the ClustalW software and refined with the software Geneious Pro 4.8.3 (www.geneious.com). For phylogenetic analysis, 49 catalase proteins from other plant species were retrieved from GenBank (http://www.ncbi.nlm.nih.gov/) and literature references. A phylogenetic tree was constructed using the neighbour-joining method with the MEGA 5.0 program and 1000 bootstrap replicates.
RNA extraction and quantitative RT-PCR
Total RNA was isolated using the TRIzol Reagent (Tiangen Biotech Co., Ltd. Beijing, China) according to the manufacturer's instructions. DNase-treated RNA (0.5 μg) was used for synthesizing the first-strand cDNA by using the M-MLV reverse transcriptase (Invitrogen, USA). Quantitative real-time PCR (qRT-PCR) was performed with SYBR Green Master Mix (Tiangen Biotech Co., Ltd. Beijing, China) on LightCycler 480 System (BioRad, USA). PCR amplification was carried out as follows: 94 °C for 5 min, followed by 40 cycles at 94 °C for 5 s, 55 °C for 10 s and 72 °C for 15 s. The relative expression level was calculated using the 2–ΔΔCT method [Citation26]. CsActin was used as the internal control and the primers for qRT-PCR analyses are listed in Table 1S (Online Supplementary Appendix). Three biological replications were performed in all reactions.
CsCAT3 recombinant protein expression and SDS-PAGE analysis
To construct a prokaryotic expression vector of CsCAT3, the open reading frame (ORF) of CsCAT3 was amplified from the cDNA of cucumber leaves, and the amplified product was cloned into pET32a. After sequence confirmation, the resulting pET32a-CsCAT3 construct was transformed into the E. coli BL21 (DE3) strain. The transformant cells harbouring the pET32a-CsCAT3 and pET32a vector were designated as BL/CsCAT3 and BL/pET32a, respectively. The BL/CsCAT3 and BL/pET32a cell lines were grown overnight in Luria–Bertani (LB) liquid medium at 37 °C, and 1 mL of overnight cultures was cultured in 100 mL fresh LB medium until the optical density at 600 nm (OD600) reached 0.6. Then the E. coli cultures were induced with 1 mmol/L isopropyl β-D-1-thiogalactopyranoside (IPTG) for an additional 4 h. The cultures were re-suspended in phosphate-buffered saline (PBS: 137 mmol/L NaCl, 2.7 mmol/L KCl, 10 mmol/L Na2HPO4 and 2 mmol/L KH2PO4, pH 7.4) after centrifugation, and then were detected by 12% sodium dodecyl sulfate polyacrylamide gel electrophoresis (SDS-PAGE) according to standard methods. In order to study the solubility of the expressed fusion protein in recombinant E. coli, the induced cells were sonicated and centrifuged, and the supernatant and the cell pellets were detected by SDS-PAGE.
Abiotic stress tolerance assay of E. coli transformants
To investigate the abiotic stress tolerance of E. coli transformants, BL/CsCAT3 and BL/pET32a were grown in LB liquid medium at 37 °C overnight. The IPTG-induced transformant strain cultures were prepared as described above until the OD600 reached 1.0, and the strains were used to perform abiotic stress tolerance assays, including heat (50 °C), freeze-thawing, sorbitol and NaCl stresses.
For thermotolerance assays, a 1-mL aliquot of the BL/CsCAT3 and BL/pET32a strains was incubated at 50 °C for different periods of time (10, 20, 30, 40 and 50 min), and then the cultures were tenfold diluted. Then, 100 μL of the diluted sample was spread onto LB agar plates with 1 mmol/L IPTG and kept at 37 °C overnight. The freeze-thawing assays were carried out as described previously with modifications [Citation27]. The strains were frozen in liquid nitrogen for 1 min and allowed to thaw at 37 °C for 15 min, which made a freeze-thaw cycle. Aliquots (1 mL) were taken after 3, 6, 9 and 12 cycles successively, and then 100 µL of dilution (1:10) was spread onto IPTG LB agar plates. For osmotic (sorbitol and NaCl) treatments, the IPTG-induced transformant strains were diluted (1:25). A 100-μL aliquot of the diluted BL/CsCAT3 and BL/pET32a strains were spread on IPTG LB agar plates supplemented with 300, 600 and 900 mmol/L concentration gradient of sorbitol, and 100, 200, 300, 400 and 500 mmol/L concentration gradient of NaCl, respectively. Cell viability was measured by counting colony-forming units with three repetitions as described previously [Citation28].
Data analysis
The results are representative or mean values from three independent replicates of three biological samples with standard deviation (±SD). Statistical analysis was performed using SPSS 16.0 software with Student's t-test in a two-tailed analysis. Values of P < 0.05 or P < 0.01 were considered to indicate statistically significant differences.
Results and discussion
Cloning and characterization of CsCAT3 gene
CsCAT3 gene (Gene ID: Csa013194) was identified from cucumber in our previous study [Citation15]. It has an open reading frame (ORF) of 1452 bp that encodes a polypeptide of 483 amino acids. Amino-acid sequence analysis revealed that the predicted polypeptide was a 56.11 kDa protein with a pI of 6.84. The polypeptide had an aliphatic index of 66.81 and the grand average of hydropathicity (GRAVY) of −0.570, indicating that the CsCAT3 protein is hydrophilic.
A search of the NCBI Conserved Domain Database indicated that the predicted protein contained a conserved structural domain for CAT to bind to protoheme (18–485 amino acids) ((A)), implying that CsCAT3 belongs to catalase_clade_1, and probably has the function of breaking down hydrogen peroxide. According to the Motif Scan and InterPro software, the catalase activity motif (CAM) and heme-binding site (HBS) of CsCAT3 were present at the position of 54–70 and 343–351, respectively ((B)). The deduced amino-acid sequence of CsCAT3 consists of the conserved catalytic active site and heme-binding site, which play important roles in catalytic function and the heme ligand, suggesting that CsCAT3 is a typical CAT. The subcellular localization prediction of CsCAT3 based on ProtComp, CELLO and Plant-mPLoc indicated that CsCAT3 is targeted to the peroxisome, and the CsCAT3 sequence indicated the presence of a putative peroxisomal targeting signal (PTS1) motif QKIASRMNVRPNI that contains conserved QKL/I/V amino acid residues at the position of 480–482 ((B)), which was reported previously [Citation29]. These results clearly demonstrated that CsCAT3 is a peroxisomal catalase, which is consistent with the results of previous studies [Citation18,Citation20,Citation30].
Figure 1. Nucleotide and deduced amino-acid sequences of CsCAT3. (A) Size and location of conserved protein domains of CsCAT3. (B) cDNA and deduced amino acid sequences of CsCAT3.
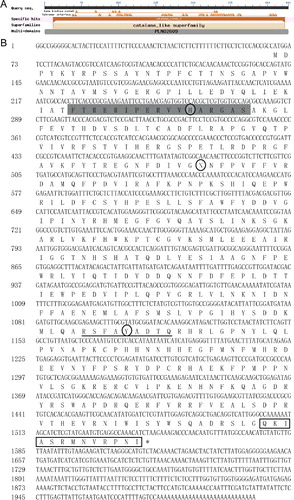
CAT is a tetrameric iron porphyrin protein and contains four small subunits (55–69 kDa, binds to four protoheme IX (protoheme b) groups [Citation31]. In order to understand the structure–function relationship, the putative three-dimensional (3D) structure of CsCAT3 was predicted by SWISS-MODEL. The protein sequence of CsCAT3 was aligned with the template protein 4qol.1.A from amino acid 17–448 with a coverage of 95%. As shown in , the predicted structure of CsCAT3 could be conceptually divided into four subunits, which is in accordance with a pervious study [Citation14]. In addition, The CsCAT3 protein and template protein shared a high degree of homology (49.04%), and the GMQE value and QMEAN Z-Score were 0.77 and −1.08, respectively, suggesting a high reliability of the predicted structure ().
Protein sequence alignment and phylogenetic relationship of CsCAT3 with other plant CATs
Sequence analysis by BLASTp in the NCBI database showed that CsCAT3 shared a 41–95% identity in the deduced amino acid sequence with the CATs from other plants, with the closest match to Cucumis melo (CmCAT3, 95%), Cucurbita moschata (CmCAT, 95%), Luffa aegyptiaca (LaCAT, 94%) and Cucurbita pepo (CpCAT3, 94%; CpCAT2, 93%). Multiple sequence alignment of CsCAT3 was carried out by selecting CATs of several plant species in literature references, including Arabidopsis thaliana (AtCAT3) [Citation8], Nelumbo nucifera (NnCAT) [Citation32], Ipomoea batatas (SPCAT1) [Citation18], Hylocereus undatus (HuCAT3) [Citation30], Brassica oleracea (BoCAT1) [Citation33], Gossypium hirsutum (GhCAT1) [Citation34], Saccharum officinarum (ScCAT1) [Citation21], Erianthus arundinaceus (EaCAT-1b) [Citation13] and Oryza sativa (OsCatB) [Citation12]. We also identified the CAM, HBS and PTS1 motifs from the selected species (). The results showed that there was little difference in the amino-acid composition of the CAM motif, but some differences were observed in the HBS and PTS1 motifs. There were only four conserved amino acids in the HBS motif, including a basic amino acid (R), a neutral amino acid (F), an acidic amino acid (D) and an uncharged catalytic residue (Y). Moreover, in the amino-acid composition of the C-terminal, only an uncharged neutral amino acid (N), two uncharged nonpolar amino acids (A and P), and the QKL/I/V amino acid residues in the PTS1 motif were conserved ().
Figure 3. Alignment of CsCAT3 with related CATs from different plant species, including Arabidopsis thaliana, Nelumbo nucifera, Ipomoea batatas, Hylocereus undatus, Brassica oleracea, Gossypium hirsutum, Saccharum officinarum, Erianthus arundinaceus and Oryza sativa.
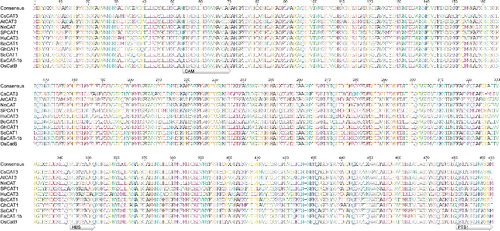
To further investigate the evolutionary relationships between CsCAT3 and other CAT proteins from different species, 49 CAT protein sequences from 33 plant species were collected and phylogenetic analysis was performed. As shown in , these CAT proteins could be grouped into dicot- and mono-cotyledonous plants based on CAT sequences, which reflects the evolution of CAT in these plants, and indicates the presence of CATs in the early evolution of plant species [Citation30]. Additionally, the dicotyledonous plants could be further divided into two clusters. CsCAT3 belongs to the second cluster in the dicot group, and exhibits close association with CmCAT3, CmCAT, LaCAT, CpCAT3 and CpCAT2, followed by HuCAT3 and SPCAT1 (), suggesting their functional similarities.
Transcript profiles of CsCAT3 under different abiotic stresses
Our previous study indicated that the expression of CsCAT3 is slightly increased under salt, drought and ABA stress conditions [Citation15]. To further investigate the expression pattern of CsCAT3 under various stress treatments, 2-week-old cucumber seedlings were subjected to different abiotic stresses including heat, cold, PEG, NaCl, H2O2 and ABA treatments and total RNAs were isolated for qRT-PCR analysis.
qRT-PCR analysis showed that the expression of CsCAT3 was significantly enhanced to different degrees by various stress treatments (). Under PEG treatment, CsCAT3 mRNA began to increase within 3 h, decreased at 6 h, subsequently reached the highest level after 12 h, and ultimately decreased at 24 h ((A)). Under NaCl treatment, the expression of CsCAT3 continued to increase after 6 h treatment, followed by a decrease at 12 h, and finally reached its maximum (18.7-fold) at 24 h ((A)). Under heat stress, the expression of CsCAT3 had two peaks at 6 and 24 h, and its maximum value was observed at 6 h (10.84-fold) ((B)). The transcription of CsCAT3 after cold treatment increased steadily from 0 to 24 h, which was different from the case of the other treatments ((B)). We also examined whether the transcription of CsCAT3 was responsive to H2O2-induced oxidative stress as well as to ABA treatment. As shown in (C), the expression of CsCAT3 gradually increased and reached the highest level (16.1-fold) at 12 h under 10 mmol/L H2O2 stress, followed by a decrease. Similar expression of CAT genes in response to exogenously applied H2O2 have been previously reported in different species, including maize [Citation35,Citation36], Arabidopsis [Citation8], ginseng [Citation16], sugarcane [Citation21], pitaya [Citation30], and Vicia sativa [Citation37]. Interestingly, the transcription of CsCAT3 was induced by ABA treatment and reached the highest level (36.77-fold) at 6 h, which was higher than that under other stresses ((C)), suggesting that CsCAT3 may be involved in ABA-dependent signalling pathways. A previous study revealed that exogenous ABA application can increase the endogenous H2O2 level, leading to the induction of the Cat1 gene expression through the ARE motif in the Cat1 promoter [Citation36]. These results suggested that CsCAT3 might serve as a positive responsive component of abiotic stress in cucumber. Various abiotic stresses can generate H2O2 in plant cells; therefore CsCAT3 might be involved in the scavenging of H2O2 produced by abiotic stresses in cucumber.
Heterologous expression of CsCAT3 protein in E. coli
To express the CsCAT3 gene in E. coli, the CsCAT3 coding sequence was cloned into pET32a and the recombinant CsCAT3 fusion protein was expressed in the E. coli BL21 (DE3) strain by induction of isopropyl β-D-1-thiogalactopyranoside (IPTG) at 37 °C for 4 h. Then the transformant cells of pET32a-CsCAT3 and pET32a were examined by electrophoresis in SDS–polyacrylamide gels. According to the construction vector, the size of the proteins deduced from BL/pET32a and BL/CsCAT3 cells should be 21.1 [Citation27] and 77.2 kDa, respectively. The SDS-PAGE results showed that a specific band about 77 kDa was detected on gels when compared with the control ((A)). The specific protein band was observed in both the supernatant and cell pellets of lysed BL/CsCAT3 cells ((B)), indicating that the CsCAT3 fusion protein was successfully expressed in recombinant E. coli.
Overexpression of CsCAT3 in E. coli enhanced growth under abiotic stress
The expression system of E. coli is a simple, convenient and effective model for determining the protein function by expressing recombinant proteins in cells subjected to stress treatments [Citation28]. Besides, E. coli cells show better growth under stress treatments by introduction of many plant genes related to stresses [Citation21,Citation28,Citation38–40], suggesting that some protective mechanisms might be common for both prokaryote and eukaryote cells under stress conditions. To explore the growth of BL/CsCAT3 and BL/pET32a under different stresses, we first investigated the cell viability of E. coli recombinants under NaCl and sorbitol treatments. Both BL/CsCAT3 and BL/pET32a cells had similar growth on LB medium under normal conditions (Figure 1S(A) in the Online Supplementary Appendix). Although the viability of BL/CsCAT3 and BL/pET32a cells decreased rapidly with NaCl treatment, the BL/CsCAT3 cells exhibited enhanced viability compared with BL/pET32a cells ((A), Figure 1S(B) in the Online Supplementary Appendix). All of the BL/pET32a cells died under 400 mmol/L NaCl treatment, whereas there were still a few BL/CsCAT3 cells alive under the same condition, even under 500 mmol/L NaCl treatment ((A)). The growth was also analysed in the LB medium with different sorbitol concentrations. As shown in (B), no significant difference in cell viability was observed between BL/pET32a and BL/CsCAT3 cells at 300 mmol/L sorbitol treatment. However, the cell viability of BL/CsCAT3 cells was much higher than that of control strains at 600 mmol/L sorbitol treatment ((B), Figure 1S(C) is in the Online Supplementary Appendix). These results revealed that the CsCAT3 gene significantly induced tolerance to salt and osmotic conditions.
Figure 7. Growth performance of BL/CsCAT3 and BL/pET32a cells under various abiotic stresses including NaCl (A), sorbitol (B), heat (C) and freeze-thawing treatment (D).
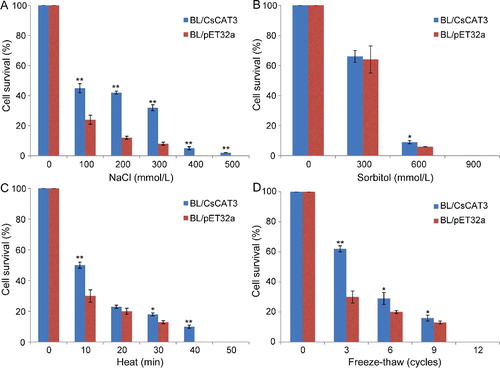
We also examined whether CsCAT3 could confer resistance to heat (50 °C) and freeze-thawing in E. coli. In heat treatment, the viability of BL/CsCAT3 cells was greater than that of BL/pET32a cells under heat (50 °C) treatment for 10 or 30 min ((C), Figure 1S(D) in the Online Supplementary Appendix). Moreover, all of the BL/pET32a cells died after 40 min, whereas about 10% BL/CsCAT3 cells remained viable under the same conditions ((C)), indicating that CsCAT3 could increase the heat tolerance of E. coli recombinants. After freeze-thawing treatment for different cycles, the BL/CsCAT3 cells showed a higher survival rate than the control BL/pET32a cells after cold treatment for 3, 6 or 9 cycles, though the difference in the survival rate decreased as the treatment cycle increased, and the cells from both strains died after 12 cycles ((D), Figure 1S(E) in the Online Supplementary Appendix). These results revealed that CsCAT3 could enhance the viability of E. coli transformants under cold stress. All of these results demonstrated that the recombinant protein of CsCAT3 enhanced the growth ability of prokaryotic E. coli strains under different stress conditions, indicating that CsCAT3 could be significant for conferring tolerance to abiotic stresses.
Previous studies have indicated that the expression of CAT genes and CAT enzyme activity can be induced by abiotic stresses [Citation8,Citation30,Citation33]. In this study, the results of qRT-PCR analysis showed that the expression of CsCAT3 is responsive to different abiotic stress conditions including heat, cold, PEG and NaCl (), implying that CAT enzyme activity is also induced under these stress conditions, and the CAT enzyme can serve as an important scavenger of H2O2, protecting the cell from the damage by H2O2 accumulation. Therefore, the significantly induced tolerance of E. coli to different stress conditions may be ascribed to the increased expression of CsCAT3 and CAT enzyme activity when exposed to these abiotic stresses. Further research is needed to elucidate the functions of CsCAT3 in cucumber.
Conclusions
In this study, a catalase gene named CsCAT3 from cucumber (Cucumis sativus) was isolated, functionally characterized, and successfully expressed in E. coli. The expression of CsCAT3 is induced by diverse abiotic stresses such as heat, cold, oxidative (H2O2) stress, osmotic (PEG and NaCl) stress and plant hormone (ABA) treatment. Overexpression of CsCAT3 in E. coli could facilitate the survival of the host cells under thermal, cold, salt and osmotic stress. Our results show that the protein might function as a positive responsive component of abiotic stresses in cucumber and should be useful for further elucidating its biological role in plants.
Supplementary_Data.pdf
Download PDF (612.6 KB)Acknowledgments
We are grateful to Prof. Zuoxiong Liu (Huazhong Agricultural University) for critical reading of the manuscript.
Disclosure statement
The authors declare that they have no conflict of interest.
Additional information
Funding
References
- Mittler R. Oxidative stress, antioxidants and stress tolerance. Trends Plant Sci. 2002;7:405–410.
- Shigeoka S, Ishikawa T, Tamoi M, et al. Regulation and function of ascorbate peroxidase isoenzymes. J Exp Bot. 2002;53:1305–1319.
- Apel K, Hirt H. Reactive oxygen species: metabolism, oxidative stress, and signal transduction. Annu Rev Plant Biol. 2004;55:373–399.
- Willekens H, Villarroel R, Van Montagu M, et al. Molecular identification of catalases from Nicotiana plumbaginifolia (L.). FEBS Lett. 1994;352:79–83.
- Skadsen RW, Schulze-Lefert P, Herbst JM. Molecular cloning, characterization and expression analysis of two catalase isozyme genes in barley. Plant Mol Biol. 1995;29:1005–1014.
- Guan L, Scandalios JG. Developmentally related responses of maize catalase genes to salicylic acid. Proc Natl Acad Sci USA. 1995;92:5930–5934.
- Frugoli JA, Zhong HH, Nuccio ML, et al. Catalase is encoded by a multigene family in Arabidopsis thaliana (L.) Heynh. Plant Physiol. 1996;112:327–336.
- Du YY, Wang PC, Chen J, et al. Comprehensive functional analysis of the catalase gene family in Arabidopsis thaliana. J Integr Plant Biol. 2008;50:1318–1326.
- Drory A, Woodson WR. Molecular cloning and nucleotide sequence of a cDNA encoding catalase from tomato. Plant Physiol. 1992;100:1605–1606.
- Lee SH, An CS. Differential expression of three catalase genes in hot pepper (Capsicum annuum L.). Mol Cells. 2005;20:247–255.
- Lin A, Wang Y, Tang J, et al. Nitric oxide and protein S-nitrosylation are integral to hydrogen peroxide-induced leaf cell death in rice. Plant Physiol. 2012;158:451–464.
- Ye N, Zhu G, Liu Y, et al. ABA controls H2O2 accumulation through the induction of OsCATB in rice leaves under water stress. Plant Cell Physiol. 2011;52:689–698.
- Liu Y, Hu X, Yao Y, et al. Isolation and expression analysis of catalase genes in Erianthus arundinaceus and sugarcane. Sugar Tech. 2016;18:468–477.
- Liu Y, Yao Y, Hu X, et al. Cloning and allelic variation of two novel catalase genes (SoCAT-1 and SsCAT-1) in Saccharum officina rum L. and Saccharum spontaneum L. Biotechnol Biotechnol Equip. 2015;29:431–440.
- Hu L, Yang Y, Jiang L, et al. The catalase gene family in cucumber: genome-wide identification and organization. Genet Mol Biol. 2016;39:408–415.
- Purev M, Kim YJ, Kim MK, et al. Isolation of a novel catalase (Cat1) gene from Panax ginseng and analysis of the response of this gene to various stresses. Plant Physiol Biochem. 2010;48:451–460.
- Mhamdi A, Queval G, Chaouch S, et al. Catalase function in plants: a focus on Arabidopsis mutants as stress-mimic models. J Exp Bot. 2010;61:4197–4220.
- Chen HJ, Wu SD, Huang GJ, et al. Expression of a cloned sweet potato catalase SPCAT1 alleviates ethephon-mediated leaf senescence and H2O2 elevation. J Plant Physiol. 2012;169:86–97.
- Kwon SI, An CS. Molecular cloning, characterization and expression analysis of a catalase cDNA from hot pepper (Capsicum an nuum L.). Plant Sci. 2001;160:961–969.
- Figueroa-Yáñez L, Cano-Sosa J, Castaño E, et al. Phylogenetic relationships and expression in response to low temperature of a catalase gene in banana (Musa a cuminata cv. “Grand Nain”) fruit. Plant Cell Tiss Organ Cult. 2012;109:429–438.
- Su Y, Guo J, Ling H, et al. Isolation of a novel peroxisomal catalase gene from sugarcane, which is responsive to biotic and abiotic stresses. PLoS One. 2014 [cited 2017 Jan 18];9:e84426. Available from:https://doi.org/10.1371/journal.pone.0084426
- Shikanai T, Takeda T, Yamauchi H, et al. Inhibition of ascorbate peroxidase under oxidative stress in tobacco having bacterial catalase in chloroplasts. FEBS Lett. 1998;428:47–51.
- Moriwaki T, Yamamoto Y, Aida T, et al. Overexpression of the Escherichia coli catalase gene, katE, enhances tolerance to salinity stress in the transgenic indica rice cultivar, BR5. Plant Biotechnol Rep. 2008;2:41–46.
- Nagamiya K, Motohashi T, Nakao K, et al. Enhancement of salt tolerance in transgenic rice expressing an Escherichia coli catalase gene, katE. Plant Biotechnol Rep. 2007;1:49–55.
- Chiang CM, Chen SP, Chen LFO, et al. Expression of the broccoli catalase gene (BoCAT) enhances heat tolerance in transgenic Arabidopsis. J Plant Biochem Biotechnol. 2014;23:266–277.
- Livak KJ, Schmittgen TD. Analysis of relative gene expression data using real-time quantitative PCR and the 2ΔΔCT method. Methods. 2001;25:402–408.
- He S, Tan L, Hu Z, et al. Molecular characterization and functional analysis by heterologous expression in E. coli under diverse abiotic stresses for OsLEA5, the atypical hydrophobic LEA protein from Oryza sativa L. Mol Genet Genomics. 2012;287:39–54.
- Liu Y, Zheng Y. PM2, a group 3 LEA protein from soybean, and its 22-mer repeating region confer salt tolerance in Escherichia coli. Biochem Biophys Res Commun. 2005;331:325–332.
- Kamigaki A, Mano S, Terauchi K, et al. Identification of peroxisomal targeting signal of pumpkin catalase and the binding analysis with PTS1 receptor. Plant J. 2003;33:161–175.
- Nie Q, Gao GL, Fan QJ, et al. Isolation and characterization of a catalase gene “HuCAT3” from pitaya (Hylocereus undatus) and its expression under abiotic stress. Gene. 2015;563:63–71.
- Zámocký M, Koller F. Understanding the structure and function of catalases: clues from molecular evolution and in vitro mutagenesis. Prog Biophys Mol Biol. 1999;72:19–66.
- Dong C, Zheng X, Diao Y, et al. Molecular cloning and expression analysis of a catalase gene (NnCAT) from Nelumbo nucifera. Appl Biochem Biotechnol. 2015;177:1216–1228.
- Lin KH, Huang HC, Lin CY. Cloning, expression and physiological analysis of broccoli catalase gene and Chinese cabbage ascorbate peroxidase gene under heat stress. Plant Cell Rep. 2010;29:575–593.
- Luo X, Wu J, Li Y, et al. Synergistic effects of GhSOD1 and GhCAT1 overexpression in cotton chloroplasts on enhancing tolerance to methyl viologen and salt stresses. PLoS One. 2013 [cited 2017 Jan 18];8:e54002. Available from: https://doi.org/10.1371/journal.pone.0054002
- Polidoros AN, Scandalios JG. Role of hydrogen peroxide and different classes of antioxidants in the regulation of catalase and glutathione S-transferase gene expression in maize (Zea ma ys L.). Physiol Plant. 1999;106:112–120.
- Guan LM, Zhao J, Scandalios JG. Cis-elements and trans-factors that regulate expression of the maize Cat1 antioxidant gene in response to ABA and osmotic stress: H2O2 is the likely intermediary signaling molecule for the response. Plant J. 2000;22:87–95.
- Abu-Romman S. Molecular characterization of a catalase gene (VsCat) from Vicia sativa. Int J Biol. 2016;8:66–76.
- Yadav NS, Rashmi D, Singh D, et al. A novel salt-inducible gene SbSI-1 from Salicornia brachiata confers salt and desiccation tolerance in E. coli. Mol Biol Rep. 2012;39:1943–1948.
- Wu Y, Liu C, Kuang J, et al. Overexpression of SmLEA enhances salt and drought tolerance in Escherichia coli and Salvia miltiorrhiza. Protoplasma. 2014;251:1191–1199.
- Wang H, Wu Y, Yang X, et al. SmLEA2, a gene for late embryogenesis abundant protein isolated from Salvia miltiorrhiza, confers tolerance to drought and salt stress in Escherichia coli and S. miltiorrhiza. Protoplasma. 2017;254:685–696.