ABSTRACT
The evolution of starch synthase (SS) has been extensively analysed in the model plant rice, but there have been few detailed studies directed towards exploring the intron splicing variant effect on the divergence of the SS gene. We report here an example that the alternative splicing of a GC-AG intron induced a new splicing isoform of SSII-1 expressed in Oryza sativa japonica cultivar Zhonghua-15. In addition, a GT-AG intron flanking the alternative splicing GC-AG intron was simultaneously spliced at an incorrect position, inducing another intron fragment gained in mature SSII-1 RNA. Moreover, when a GT-AG intron from SSII-3 was artificially mutated into the GC-AG intron, a 28 bp fragment from the intron added to the mature RNA could be observed in transgenic rice. In this study, the alternative intron splicing of the GC-AG intron might offer more variants for genetic divergence of the starch synthase gene family in rice.
Introduction
Starch is one of the most abundant polysaccharides in higher plants and consists of αα-1,4-linked gulcan chains linked through αα-1,6 branch bonds. Starch synthases (SSs) transfer the glucosyl moiety of adenosine diphosphate (ADP)-glucose to the non-reducing end of a pre-existing αα-1,4-glucan to extend these polysaccharides [Citation1–3]. The evolution of SS genes may follow a ‘simple to complex’ rule as starch becomes a complex and orderly product from simple eukaryote cells to higher plants. Observation of the evolution of the SS gene family could provide evidence of how a protein gave rise to a protein family.
The presence of multiple spliceosomal introns is a characteristic feature of eukaryotic genes [Citation4,Citation5]. However, the origins of introns and the process of their evolution are poorly understood. More and more people want to explain the relationship between the intron splicing and the evolution of gene family through analysing the characteristics of introns using statistics [Citation6–9]. Here we attempt to offer a direct example of how alternative intron splicing could have contributed to the expansion of the SS gene family in rice.
It is not clear what determines the introns position and their sequence content [Citation10–12]. Some evidence supports the idea that introns existed before any eukaryote–prokaryote divergence, and the prokaryotic lineage completely lost its introns, whereas they were retained in the eukaryotes [Citation10,Citation13,Citation14]. In contrast, some evidence suggests that introns appeared relatively late in the evolution of eukaryotes [Citation15,Citation16], but where the introns got inserted is not clear. In fact, a large number of introns are lost and gained frequently in the evolution of genes. It is known that there exist variants to standard form GT-AG introns [Citation17,Citation18]. Most of these variants are GC-AG, AT-AC and GT-GG. The GC splice sites account for 0.6% of the annotated donor sites [Citation19] in humans. It is believed that the human genes can have alternative exon–intron structure not only with changed GT-AG introns [Citation20,Citation21], but also with GC-AG introns [Citation22]. Thanaraj and Clark [Citation22] obtained evidence that 60% of GC-AG introns are alternatively spliced. Alternative splicing in Arabidopsis root tissues can create novel gene regulations in adaptation to changing environments [Citation23,Citation24]. However, very little is known about the evolution of alternative splicing, other than that the naturally occurring GC splice donor serves as a weak splice donor that is both evolutionarily conserved and required for regulated splicing [Citation25]. In this work, when the GT-AG intron from SSII-3 was mutated into a GC-AG intron, additional sequence from the intron added to mature RNA could be observed in the transgenic plants. Nevertheless, the GC-AG ‘wrong’ splicing to GT-AG also generates a fragment of intron conjunct into the mRNA sequence of SSII-1, indicating that the GC-AG intron is more adaptable to the regulation of alternative splicing than the GT-AG intron in the rice SSII-1 gene. Irrespective of whether the GC-AG introns are normal or artificial isoforms, they tend to be spliced as a GT-AG intron perhaps for its weaker consensus sequence at their donor sites than the GT-AG intron in the SSII gene.
Materials and methods
Plant material
Kernels of rice (Oryza sativa japonica cultivar Zhonghua-15) were harvested from the experimental field at 15 days after pollination, frozen in liquid nitrogen and then stored at −80 °C.
Isolation of total RNA
Total RNA was extracted from rice kernels using the improved method of Ma and Yang with TRIzol™ reagent (Invitrogen, CA, USA) as described [Citation26,Citation27]. It was then digested by DNase (Promega, WI, USA) and was kept at −20 °C until used.
Sequence searches and phylogenetic analysis
To identify the members of the SS gene family, a search for OsSS cDNA was performed using the BLAST program in the GenBank database (https://www.ncbi.nlm.nih.gov/genbank/). We searched for OsSSII genes cDNA whose intron-exon structures could be confirmed by the knowledge of their DNA sequences. The presence and location of introns were manually evaluated and then mapped onto their DNA sequence using Vector NTI software.
Multi-sequence alignment analysis for the deduced amino-acid sequence was carried out using the CLUSTAL X/W program. The alignment analysis results were performed with TreeView software.
Northern blots
To make an end-labelled probe, short oligonucleotides were synthesized on Applied Biosystems 3400 DNA Synthesizer and the sequences were designed as follows:
Probe 1: 5’-GTACATAAATTGAAAATACAAGATTACTATGAACTTCCCAAA-3’
Probe 2: 5’-AAGATCATAATAGCCACATACCTTCTAATTTTTTCAGTTTCC-3’
Probe 3: 5’-TGCTGGTAGAAGCGTGTGCTGCCCGCATGAGGTTGAGAGCGT-3’
OsSSII-1 3’UTR: 5’-TCAGTCCAAGAAGACCTCGACGGTTGTTCAGTCATTCATGAC-3’
AtSSII 3’UTR: 5’-GTTAAATAAAAGTTACCTTTTTTACTGTCTATAAAGAACATG-3’
Added Exon: 5’-GCATTACTCCTTTTTTCTCTCCTTCATG-3’
Then, the oligonucleotides were 5’-32P labelled with an oligonucleotide 5' end labelling system (Perkin–Elmer, Norwalk, CT) using the gamma phosphate of adenosine triphosphate (ATP) containing 32P and were used for hybridization.
Total RNA (20 μg) was subjected to electrophoresis in a 1% agarose gel containing 37% formaldehyde and was then transferred onto a Hybond-N+ membrane (Amersham Pharmacia Biotech, Buckinghamshire, UK) and hybridized in ExpressHyb™ hybridization solution (Clontech Laboratories Inc. CA, USA) for 2 h at 68 °C. The membrane was washed twice in 2 × SSC buffer (0.15 mol/L NaCl plus 0.015 mol/L sodium citrate, pH 7.0)–0.05 sodium dodecyl sulphate (SDS) for 20 min at room temperature. It was then washed twice in 0.1 × SSC–0.1 SDS for 20 min at 40 °C and finally in 0.1 × SSC–0.1 SDS for 2 min at 55 °C. Then the signal was detected by an imaging system Storm 860 (Amersham Biosciences, NJ, USA).
Production of transgenic rice plants
Artificial OsSSII-3 gene containing an intron-3 mutation (a single point mutation in the splice site of intron-3 to construct the mutation of GT-AG to GC-AG) was synthesized. It harboured BamHI and SalI sites at both ends. The fragments were inserted in the pCAMBIA-1205 plasmid under the cauliflower mosaic virus 35S promoter. These plasmids were introduced into Agrobacterium tumefaciens LB4404 and transformed into rice plants (Oryza sativa japonica cultivar Zhonghua-15). Regenerated plants were transferred to soil and were grown to maturity. Transgenic lines were selected on the basis of resistance to a phosphinothricin-based herbicide. Phosphinothricin acetyl transferase, encoded by the bar gene, detoxifies phosphinothricin herbicides.
Determination of transgenic rice plants
The rice kernel genomic DNA was isolated from two transgenic rice plants and one non-transformed control plant. Genomic DNA was amplified with the primers S1 and S2 (5’-TGCGGTAGAAGAGGAGAC-3’, 5’-TGTAAGAATGGAAGAAACATC-3’) set specifically for the OsSSII-3 fragment with the following polymerase chain reaction (PCR) cycling conditions: 94 °C/45 s, 53 °C/45 s, 72 °C/1 min for 35 cycles (GeneAmp PCR System 2700; Applied Biosystems, CA, USA). The amplified products were analysed by electrophoresis in 1.0% agarose gels containing ethidium bromide.
Results and discussion
Structural polymorphisms in SS genes
The members of rice SS genes have been summarized by Hirose et al. [Citation28]. For classification of the OsSS gene family members, cDNA sequences containing the entire coding region were used, and a multi-sequence alignment analysis was carried out. The result from the CLUSTAL W analysis is shown in (A). The deduced OsSS peptide sequences could be clearly grouped into five classes. For example, soluble SSI, SSII, SSIII, SSIV and granule-bound starch synthase (GBSS). In the 10 types of rice starch synthases, one isoform existed in the SSI class, two isoforms existed in SSIII, SSIV and GBSS classes, and even more, three isoforms existed in the SSII class.
Figure 1. Evolution of SS genes. (A) Phylogenetic trees derived from deduced SS amino-acid sequences. The GenBank accession numbers for the SS genes are: rice (Oryza sativa) SSI, D16202; SSII-1, AF383878; SSII-2, AF395537; SSII-3, AF419099; SSIII-1, AF432915; SSIII-2, AY100469; SSIV-1, AY100470; SSIV-2, AY100471; GBSSI, X65183; GBSSII, AY069940; the scale bar represents the branch length (number of amino-acid substitutions/10 residue). (B) Exon–intron diagram of SSII genes. The exons are indicated as dark black boxes and the introns are shown as thick grey lines. The exons diagram only represents the SSII CDSs (coding sequences) in the genomic DNA. The GenBank accession numbers for the SSII genomic sequences are: rice (Oryza sativa) SSII-1, AAAA02029759; SSII-2, AAAA02007387; SSII-3, AAAA02018632.
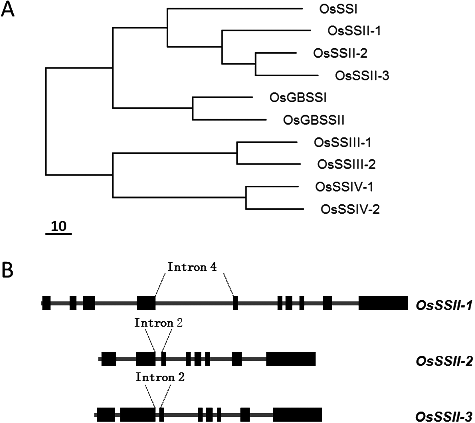
The exon–intron structure of three OsSSII genes was constructed by aligning the cDNA sequences and their genomic sequences. A schematic representation of the position of introns is shown in (B) for representative SSIIs. Generally, some exons are located in the 5’- and 3’-untranslated regions (UTRs) of genes. In this work, we excluded these exons and named the exon from ATG exon-1. Thus, the OsSSII genes were found to contain eight to ten exons from the ATG to the TGA codon. From the conservative exon fragment, intron-4 of SSII-1 showed the same location as intron-2 from SSII-2 and SSII-3. SSII-1 contains more exons than SSII-2 and SSII-3, and its intron-4 is longer than the corresponding intron-2 from SSII-2 and SSII-3.
In SSII, three different soluble isoforms are present, where the time of divergence of SSII-1 is earlier than that of SSII-2 and SSII-3 ((A)). Similarly, the exon–intron structure of the SSII genes sub-family showed that SSII-1 was different from other SSIIs, for SSII-1 contains more and longer introns. This suggests that the change of intron length and splice cite may have been the driving force behind the division of the SSII sub-family in rice. Jiang et al. [Citation29] also suggested that the evolution of the SSII gene is associated with the replication of a larger genomic fragment. It could be speculated that rice SSII-2 and SSII-3 have diverged after they got separated from SSII-1. The intron-2 was more highly conserved between SSII-2 and SSII-3 than the intron (intron-4) located at same splice site in SSII-1. In this case, alterations in intron-4 sizes maybe a new factor to promote further division the SSII sub-family into new isoforms.
Alternative splicing in OsSSII-1
The NCBI (National Center for Biotechnology Information) database reported two types of SSII-1 gene, with accession numbers AF383878.1 and NM_196673.1, respectively. AF383878.1 exists in both indica and japonica rice variety [Citation29], and NM_196673.1 is found in an indica rice variety [Citation30]. To test whether NM_196673.1 transcripts are also expressed in japonica rice variety, Zhonghua-15 (japonica rice) was chosen as plant material. Of these two different splicing variants, we were able to clone the AF383878.1 complete sequence gene but failed to clone the full-length sequence of NM_196673.1 in Zhonghua-15. The intron-4 of SSII-1 (AF383878.1) was shown to have two different characteristics as compared to intron-2 in other SSII genes: being ‘longer’ and of a ‘GC-AG’ form ((B) and (A)). Since these two characteristics are unique in SSII, we observed that intron-4 of NM_196673.1 is a GT-AG form with ‘added’ exons. At the same time, another upstream GT-AG intron (intron-3) seems to have been affected, in which the AG│GT conservative splicing point may have shifted forward into a A│GGT, consequently transforming its corresponding intron into a GG-CA form. Moreover, a fragment of intron-3 has most probably become an exon sequence, while the exon flanking the 5' side of intron-3 is missing a short fragment ((B)).
Figure 2. Schematic representation of the alternative splicing in the GC-AG intron (intron-4) of the rice SSII-1 gene. (A) SSII-1 gene with accession number AF383878.1 and (B) SSII-1 gene with accession number NM_196673.1.
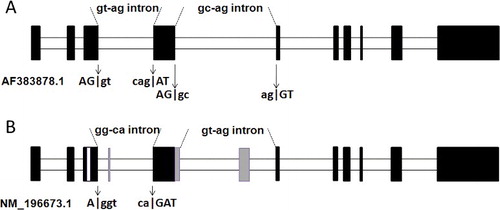
In order to clarify whether NM_196673.1 and AF383878.1 splicing variants appeared in our experimental material together (Zhonghua-15), we designed an additional exon as a northern hybridization probe: prob-1, -2 and -3 ((A)). The Northern blotting results showed that the NM_196673.1 isoform existed with AF383878.1 in kernels of rice Zhonghua-15((B)).
Figure 3. Alternative splicing in OsSSII-1. (A) Exon–intron diagram of SSII-1 genes. Black boxes correspond to the gene CDS from AF383878.1; grey boxes represent the added exon from NM_196673.1; all ‘new’ exons are designed probes for northern blots, respectively. (B) Northern blots. The added exon probes and 3’UTR probe could be detected in the total RNA.
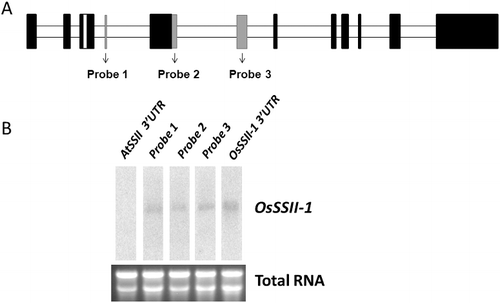
The GC-AG intron presented in AF383878.1, which rarely appeared in OsSS introns, could have resulted in shifted splicing and could have led to the appearance of a GT-AG intron; and exon-3 and -4 of SSII-1 were changed in NM_196673.1. Yet, if intron-3 (GT-AG form) was incorrectly spliced as a GG-CA form, NM_196673.1 must have gained or lost exons during splice site changes (). The frequency of this rare class of GC-AG introns is only 0.6% in humans [Citation19] and C. elegans [Citation25], and alternative GC-AG introns have also been reported by Thanaraj et al. previously [Citation31]. The GC-AG intron in rice SSII-1 could be considered to give us a case in which a rare intron may have formed a ‘new’ splice site sequence, and this splice signal site may have migrated or remained as a consensus sequence (GT-AG form) for stabilizing the new transcripts.
A comprehensive review of spliceosomal intron gain models has been well summarized by Yenerall and Zhou [Citation17], including intron transposition, transposon insertion, tandem genomic duplication, intron gain during double-strand break repair, insertion of a Group II intron and intron transfer mechanisms. Up to now, only three models have been proposed for the mechanism of intron loss [Citation32–35]. The first is that introns are deleted by recombination between the genomic DNA and homologous cDNA. The second model is that introns are deleted with the flanking exonic sequences. The third one is that introns are lost during non-homologous end-joining repair of DNA double-strand breaks. In our study, like the second model, direct attempts on identification of intron lost (fused into exon) by northern blot were successful in producing some substantial clues that an intron could be lost due to the alteration of the splice site of a GC-AG intron. Whereas the mechanisms of intron loss mostly remain unknown, the possibility that the splice site of GC-AG may have been altered, with this change causing the intron to fuse with the exon, is an interesting hypothesis that could explain the alternative splice variants driving the evolution of the SSII gene family.
Alternative splicing in artificial GC-AG intron
Although the deduced amino acid sequences of exon-5 of OsSSII-1 (GLGDVAGALPKALARRGHRVM), exon-2 of OsSSII-2 (GLGDVVGALPKALARRGHRVM) and exon-2 of OsSSII-3 (GLGDVAGALPKALARRGHRVM) were highly similar, the types of upstream flanking introns were different. Intron-2 of OsSSII-2 was predicted to be a GT-AG type, but intron-2 of OsSSII-3, like intron-4 of OsSSII-1, is also a GC-AG type. In terms of intron types, OsSSII-3 was observed to be closer to OsSSII-1 than OsSSII-2 during the evolution of the OsSS superfamily. Therefore, OsSSII-3 was used for this experiment. OsSSII-3 of the t→c mutation into Zhonghua-15 was successfully transformed into rice using an Agrobacterium-mediated transformation system (online supplementary Figure 1S). Preliminary observations did not find the OsSSII-3 (t→c) mutant phenotype affected. Field observations and surveys showed that transgenic OsSSII-3 (t→c) plants grew well, in a neat line, without any observed phenotypic variation. To screen the target transgenic plant, the key is to ensure that the transgenic plants contain the true positive OsSSII-3 (t→c) mutation gene. Using primers S1 and S2, a 430-bp fragment was amplified from non-transgenic plants, whereas the vast majority of the transgenic plants yielded a 430-bp and 330-bp fragment ((F)). The 430-bp fragment represents the endogenous template, a homologous competitive template, whereas the 330 bp fragment represents the OsSSII-3 (t→c) mutation gene. The extra 100 bp are intron-2 of the endogenous OsSSII-3 gene.
When the transgenic rice with the GT-to-GC mutation was constructed in the third intron of the OsSS-3 gene, the splicing form of intron-3 was found to have changed. It is possible that the intron-3 splice sites did not account for proper GC-AG cutting, but pushed back about 28 bp to form a new GT-AG splicing pattern ((A)). Here, for the determination of a new cleavage site, the 28-bp fragment was designed as northern probes, and the northern blot experimental results showed that the 28-bp fragment of the intron does appear in the total RNA from rice kernel ((B)). Because the OsSSII-3 PCR fragment was undetected in the cDNA of transgenic plants with primers S1 and S2 (the S2 primer tightly annealed to the 28-bp fragment downstream), the 28-bp fragment was most probably exactly transformed from an intron into an exon, and the region downstream of the 28-bp fragment remains to be an intron sequence after alternative splicing. It could be speculated that, when the GT-AG changes into a GC-AG intron, the intron of the splicing pattern can change, thus possibly forming the new GT-AG intron.
Figure 5. Alternative splicing in intron-3 of SSII-3. (A) Schematic representation; black boxes represent the exon; grey boxes represent the added 28-bp exon. (B) Northern blots. The 28-bp probes could be detected in total RNA separated from transgenic rice line 2.
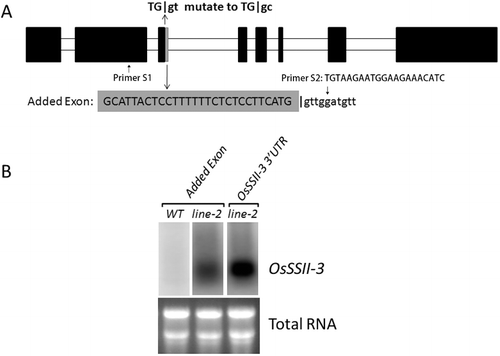
In this study, a short fragment flanking the intrinsic intron cleavage site was proved to have merged with the exon. Here we provide evidence of alternative splicing in artificial GC-AG intron inducing the appearance of alternative splice variants in the rice SSII gene.
Conclusions
The results of our study suggest that AF383878.1 and NM_196673.1, the different transcripts of OsSSII-1, not only both existed in indica rice, but also both existed in a japonica rice variety Zhonghua-15. Through comparing these two splice isoforms, we suggested that a GC-AG intron in rice SSII-1 may have formed a ‘new’ splice site sequence, and this splice signal site may have migrated or remained fixed as a consensus sequence (GT-AG form) to stabilize the new transcripts. Furthermore, when intron-3 of OsSSII-3 was constructed as a GT-AG shift into a GC-AG model, the alternative splicing site was detected in the transgenic rice. Unfortunately, since no phenotypic alterations were found in the OsSSII-3 (t→c) mutants, it is not possible to discuss any functional and evolutional aspects of the induced splicing variant. We propose that more intron (t→c) mutants, when larger data sets are examined, may provide such information.
Supplementary_Data.pdf
Download PDF (509.6 KB)Acknowledgments
The authors are grateful to Dr Zhang Tiegang (Beijing Preventive Medicine Research Center, Beijing Center for Disease Prevention and Control, Beijing, China) for providing the plasmid pCAMBIA1205.
Disclosure statement
The authors have declared that no competing interests exist.
Additional information
Funding
References
- Ball SG, Morell MK. From bacterial glycogen to starch: understanding the biogenesis of the plant starch granule. Annu Rev Plant Biol. 2003;54:207–233.
- James MG, Denyer K, Myers AM. Starch synthesis in the cereal endosperm. Curr Opin Plant Biol. 2003;6:215–222.
- Pfister B, Zeeman SC. Formation of starch in plant cells. Cell Mol Life Sci. 2016;73:2781–2807.
- Roy SW, Irimia M. Diversity and evolution of spliceosomal systems. Methods Mol Biol. 2014;1126:13–33.
- Dacks JB, Doolittle WF. Reconstructing/deconstructing the earliest eukaryotes: how comparative genomics can help. Cell. 2001;107:419–425.
- Boutz PL, Bhutkar A, Sharp PA. Detained introns are a novel, widespread class of post-transcriptionally spliced introns. Genes Dev. 2015;29:63–80.
- Mercer TR, Clark MB, Andersen SB, et al. Genome-wide discovery of human splicing branchpoints. Genome Res. 2015;25:290–303.
- Hsiao Y-HE, Bahn JH, Lin X, et al. Alternative splicing modulated by genetic variants demonstrates accelerated evolution regulated by highly conserved proteins. Genome Res. 2016;26:440–450.
- Tran VDT, Souiai O, Romero-Barrios N, et al. Detection of generic differential RNA processing events from RNA-seq data. RNA Biol. 2016;13:59–67.
- Fedorov A, Cao X, Saxonov S, et al. Intron distribution difference for 276 ancient and 131 modern genes suggests the existence of ancient introns. Proc Natl Acad Sci USA. 2001;98:13177–13182.
- Bondarenko VS, Gelfand MS. Evolution of the exon-intron structure in ciliate genomes. PloS One. 2016 [cited 2017 Feb 25];11:e0161476. DOI:10.1371/journal.pone.0161476
- Keane PA, Seoighe C. Intron length coevolution across mammalian genomes. Mol Biol Evol. 2016;33:2682–2691.
- de Souza SJ, Long M, Klein RJ, et al. Toward a resolution of the introns early/late debate: only phase zero introns are correlated with the structure of ancient proteins. Proc Natl Acad Sci USA. 1998;95:5094–5099.
- Roy SW, Nosaka M, de Souza SJ, et al. Centripetal modules and ancient introns. Gene. 1999;238:85–91.
- Logsdon JM. The recent origins of spliceosomal introns revisited. Curr Opin Genet Dev. 1998;8:637–648.
- Zhaxybayeva O, Gogarten JP. Spliceosomal introns: new insights into their evolution. Curr Biol. 2003;13:R764–766.
- Yenerall P, Zhou L. Identifying the mechanisms of intron gain: progress and trends. Biol Direct. 2012 [cited 2017 Feb 25];7:29. DOI:10.1186/1745-6150-7-29
- Srinivasan A, Jiménez-Gómez JM, Fornara F, et al. Alternative splicing enhances transcriptome complexity in desiccating seeds. J Integr Plant Biol. 2016;58:947–958.
- Chong A, Zhang G, Bajic VB. Information for the coordinates of exons (ICE): a human splice sites database. Genomics. 2004;84:762–766.
- Croft L, Schandorff S, Clark F, et al. ISIS, the intron information system, reveals the high frequency of alternative splicing in the human genome. Nat Genet. 2000;24:340–341.
- Modrek B, Lee C. A genomic view of alternative splicing. Nat Genet. 2002;30:13–19.
- Thanaraj TA, Clark F. Human GC-AG alternative intron isoforms with weak donor sites show enhanced consensus at acceptor exon positions. Nucleic Acids Res. 2001;29:2581–2593.
- Swarup R, Crespi M, Bennett MJ. One gene, many proteins: mapping cell-specific alternative splicing in plants. Dev Cell. 2016;39:383–385.
- Li S, Yamada M, Han X, et al. High-resolution expression map of the Arabidopsis root reveals alternative splicing and lincRNA regulation. Dev Cell. 2016;39:508–522.
- Farrer T, Roller AB, Kent WJ, et al. Analysis of the role of Caenorhabditis elegans GC-AG introns in regulated splicing. Nucleic Acids Res. 2002;30:3360–3367.
- Mornkham T, Wangsomnuk PP, Fu Y-B, et al. Extractions of high quality RNA from the seeds of Jerusalem artichoke and other plant species with high levels of starch and lipid. Plants Basel Switz. 2013;2:302–316.
- Ma XB, Yang J. An optimized preparation method to obtain high-quality RNA from dry sunflower seeds. Genet Mol Res. 2011;10:160–168.
- Hirose T, Terao T. A comprehensive expression analysis of the starch synthase gene family in rice (Oryza sativa L.). Planta. 2004;220:9–16.
- Jiang H, Dian W, Liu F, et al. Molecular cloning and expression analysis of three genes encoding starch synthase II in rice. Planta. 2004;218:1062–1070.
- Rice Chromosome 10 Sequencing Consortium. In-depth view of structure, activity, and evolution of rice chromosome 10. Science. 2003;300:1566–1569.
- Thanaraj TA. A clean data set of EST-confirmed splice sites from Homo sapiens and standards for clean-up procedures. Nucleic Acids Res. 1999;27:2627–2637.
- Rodríguez-Trelles F, Tarrío R, Ayala FJ. Origins and evolution of spliceosomal introns. Annu Rev Genet. 2006;40:47–76.
- Farlow A, Meduri E, Schlötterer C. DNA double-strand break repair and the evolution of intron density. Trends Genet. 2011;27:1–6.
- Roy SW, Gilbert W. The evolution of spliceosomal introns: patterns, puzzles and progress. Nat Rev Genet. 2006;7:211–221.
- Ma M-Y, Che X-R, Porceddu A, et al. Evaluation of the mechanisms of intron loss and gain in the social amoebae Dictyostelium. BMC Evol Biol. 2015 [cited 2017 Feb 25];15:286. DOI:10.1186/s12862-015-0567-y