ABSTRACT
The purpose of this study was to evaluate the effect of photombiomodulation (PBM) and locally applied simvastatin on bone formation in critical size defects of the experimental rat model calvarium. A total of 28 Spraque-Dawley male rats, mean age of 18 weeks, were used. Bilateral 5 mm critical sized calvarial defects were created. Then 0.5 mg/mL simvastatin solution was inoculated into gelatin sponge and locally applied on one side. The other side served as control. Half of the animals were subjected to PBM treatment and the remaining half were left for spontaneous healing. The animals were sacrificed by guillotine at days 8 and 15. The specimens were histopathologically analysed. The histopathologic evaluation revealed that the new bone formation levels were significantly higher at the 15th day compared to the 8th day. The bone formation levels with PBM and simvastatin treatment either combined or applied individually were significantly higher than the control ones. PBM and local simvastatin applications had favourable effects on the early phase of new bone formation and reduced the bone healing period in the rat model. Further human trials must be performed for evaluation of the reliability of this protocol.
Introduction
Current investigations are challenged daily by the need to recover bone loss due to surgical procedures, trauma or pathological conditions. Several techniques for the repair of bone defects have been studied with the use of bone grafts and photobioengineering [Citation1–3]. The use of bone grafts for repairing the maxillofacial deformities is a relatively common procedure. Autologous bone is widely regarded as the gold standard graft material [Citation4]. However, the procedure has significant limitations and disadvantages. Owing to such issues, various materials have been developed as substitutes to autologous bone grafts [Citation5]. The studies focus on cost effective biological compounds [Citation6]. Recombinant human bone morphogenic protein-2 (BMP-2) and vascular endothelial growth factor (VEGF) have proved to be the most effective anabolic factors to stimulate osteoblactic activity in bone defects [Citation7]. BMPs are important regulators of osteogenic differentiation and BMP-2 is the most potent osteoinductive factor. VEGF is an angiogenic cytokine and may induce both proliferation and differentiation of osteoblasts by stimulating endothelial cells to produce osteoanabolic factors [Citation8,Citation9]. However, short shelf life and high prices are the main limitations to use these materials in clinical practise [Citation10]. Eliminating these disadvantages using pharmacologic compounds that can upregulate the necessary autogenous growth factors is a cost-effective way to correct bone defects.
The 3-hydroxy-3-methylglutaryl coenzyme A (HMG-CoA) reductase inhibitors, statins, are widely prescribed drugs to prevent cardiovascular disorders [Citation11]. Statins inhibit the synthesis of mevalonate by HMG-CoA reductase, the limiting step in the cholesterol synthesis pathway [Citation12]. In addition to their cholesterol lowering activity, statins have different beneficial effects. Except their specific effects, all their influences are due to pleiotropic effect. Statins have a wide range of pleiotropic effects, including increased angiogenesis, anti-inflammatory effect, antioxidant effect and anabolic effect [Citation11]. A role for statins on bone metabolism was first proposed by Mundy et al. [Citation13]. They suggested that statins could have anabolic effect on bone formation by BMP-2 and VEGF activation. The second hypothesis on bone metabolism suggested that statins inhibit osteoclastic activity [Citation13]. Simvastatin, which is a type of a lipophilic statin, shows high efficacy on bone cells unlike hydrophilic statins. The lipophilic feature, low price, extended shelf life, simple preparation and application are the main reasons to use simvastatin.
Laser light has been used for accelerating bone repair in autologous bone grafts [Citation1], dental implants [Citation14] and different types of bone defects [Citation15]. Low-intensity light therapy, ordinarily referred to to as photombiomodulation (PBM) by light in the far-red to near-infrared (NIR) region of the spectrum (630–1000 nm), stimulates several cellular functions. PBM therapy has been shown to downregulate osteoclastogenesis, increase the cell proliferation including that of fibroblasts and to promote angiogenesis thus accelerating the bone repair process [Citation1,Citation2,Citation16]. The use of light-emitting diodes (LEDs) and low level lasers (LLLs) are favourable therapeutic approaches in the treatment of infected and hypoxic wounds [Citation17,Citation18].
Despite the growing number of successful applications of LED-PBM in many fields, there are limited reports concerning their effects on bone repair. Several studies have suggested that the use of PBM is one of the most suitable protocols for improving bone repair due to its larger penetration depth in the bone tissue when compared to visible light [Citation19]. Although several studies have demonstrated positive results in the healing of bone tissue with the use of PBM, there are yet few reports on the use of combinations of PBM and biomaterials [Citation20,Citation21]. The effects of PBM in bone repair associated with graft biomaterials needs to be further studied as each material may cause different responses.
As light has been proved to speed up bone repair, it would be desirable to develop a technique to enhance and shorten the time of bone repair in cases of the use of biomaterials [Citation20]. The aim of this study was to histopathologically evaluate the effect of PBM and locally applied simvastatin on bone formation in 5 mm critical sized defects (CSDs) formed in rat calvaria.
Materials and methods
Animal care
The protocol for the study was approved by the Institutional Review Board of Yeditepe University Laboratory Animals Ethics Committee (No: 285). Twenty-eight 13-week-old Sprague-Dawley male rats (mean weight 281 g) were kept under natural conditions of light and temperature at the Laboratory of Yeditepe University Faculty of Medicine Research Laboratories. The animals were fed with standard laboratory diet and had water ad libitum. Controlled day/night light cycle and temperature were maintained during the experiment. The animals were randomly divided into two groups (Group I and Group II) and then subdivided into two subgroups (Group A and Group B) according to the day of sacrificing ().
Table 1. Distribution of the rat groups.
Sample preparation
Simvastatin solution (0.5 mg/mL) as prepared in phosphate buffered saline and was kept at +4 °C in darkness [Citation22]. A colloid silver ingredient resorbable collagen sponge carrier (Gelatamp®; Coltene/Whaledent, Ohio, USA) was used to release the simvastatin solution slowly on the operation site. The collagen sponge was cylinder shaped by a soft tissue punch bur 4 × 10 mm sized and 0.02 g weight. The cylinder shaped collagen sponge absorbed 0.2 mL simvastatin solution at room temperature.
Study protocol
All procedures were performed in a surgical operating room under sterile conditions. All Sprague–Dawley rats were sedated with an intramuscular injection of ketamine (Ketalar ®, Pfizer, Turkey, 50 mg/mL) and 2% xylazine (Rompun®, Bayer, Canada, 20 mg/mL, 2 mL/kg). A semilunar incision was made at the scalp in the anterior region of the calvarium and full-thickness flap was reflected towards the posterior region of the skull. Bilateral 5-mm diameter CSDs were made with a trephine (3i Implant Innovations Inc., FL, USA) used in a low-speed handpiece under continuous sterile saline irrigation to avoid thermal injury at parietal bones. During the operation, bregma point and sagittal suture were identified as the landmarks. The defect located on the left side was filled with simvastatin impregnated collagen sponges; the right side defects were left empty and served as the control group. Periosteal and subcutaneous tissues were sutured with resorbable 4/0 polyglactin sutures (Pegelak®; Doğsan, Trabzon, Turkey). The skin was then sutured with non-resorbable 3/0 silk sutures (İpek; Doğsan; Trabzon, Turkey). All the wound areas were cleaned with antiseptic solution after surgery. Group I received PBM therapy and Group II did not receive any LED therapy.
PBM protocol
PBM was carried out using a LED array with a wavelength of 625 nm and with 20 mW/cm2 (OsseoPulse® AR 300; Biolux, Vancouver, Canada) of output power. The LED device was stabilized at a 10-cm height to the operation site. All the rats were sedatized during the section. PBM was started immediately after the surgery and transcutaneously applied for 20 min at 24-h intervals for 7 and 14 consecutive days. The resulting total dose to the surface of the test site was 24 J/cm2 per day, with the treatment over a surface area of 3.6 cm2. To avoid dehidratation and to support the animal metabolism, 5 mL 5% dextrose and 5 mL 0.9% saline solutions were injected subcutanously after all PBM sections. The animals were sacrificed on the 8th and 15th day. Sacrificing was done 24 h after the final irradiation day (7th and 14th day) to observe the effect of the last day irradiations. The area of the surgical defects and the surrounding tissues were removed in block. A silk suture was fixed through a hole made by a bur at the neighbourhood of the empty defect in order to differentiate the defects. No postoperative complications were observed in any of the 28 rats and 56 bone defects for final analysis. No weight loss, no sign of infection on the calvarial soft tissue and no side effects, such as behavioural changes or signs of pain, were observed.
Histopathologic and histomorphometric analysis
The parietal bone specimens were were fixed in 10% neutral formalin solution for 48 h at room temperature. All materials were decalcified in the solution of 0.5% formic acid and 0.2% sodium citrate for the decalcification procedure and remained at constant temperature for 2–4 days. Bone samples were embedded in melted paraffin wax and stored at −4 °C. Paraffin blocks were obtained with a conventional microtome at 5–7 µm thickness. The histological sections were stained with hematoxylin eosin (H&E).
The examination of bone preparations was adopted according to Allen's scoring system () [Citation23]. Histopathological analysis was carried out using semi-quantitative methods by two pathologists (blinded to the PBM group) under a light microscope (Olympus BX51®, Optical Co. Ltd, Tokyo, Japan). The scores for inflammation and fibrosis were determined by counting the associated cells and their ratio to the total cell count as – (0%), 1 (+) (5%–30%), 2 (++) (30%–60%), or 3 (+++) (> 60%) in a standardized area at various magnifications by FIVE image analysis software (Olympus®, Tokyo, Japan). Four histologic sections representing the center of the original surgical defect were selected for the histologic analysis and the histomorphometric analysis in order to increase the reliability of the data used in the statistical analysis.
Table 2. Fracture healing scoring system.
Statistical analysis
The statistical analysis was performed by the Statistical Package for Social Sciences (SPSS, version 17.0) program. Mann–Whitney U test was used to statistically compare the control and study groups. The mean differences in each group were analyzed by Wilcoxon's signed-rank test with a confidence interval of 95% (p < 0.05).
Results and discussion
According to Allen’ s scoring system, on the 8th postoperative day, the histological findings demonstrated that the new bone formation at the simvastatin-treated defect in Group I-A was significantly higher (p < 0.05) than that in Group II-A (). The new bone formation at the control defect in Group I-A was more pronounced than that in Group II-A, although the difference was not significant. The fibrosis values of the simvastatin-treated defects in Group I-A were significantly lower than those in Group II-A (p < 0.05). The inflammation values of the control defects in Group I-A were significantly lower (p < 0.05) than those in Group II-A ().
Figure 1. Group I-A with simvastatin. New bone formation around the simvastatin with active connective tissue and rich neovascularisation structure (H&E × 200).
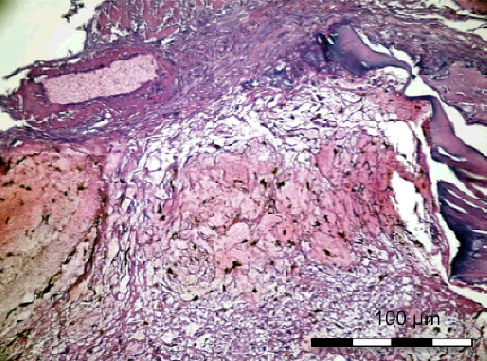
Figure 2. Group I-A without simvastatin. Osteoblasts, differentiated young mesenchymal stem cells and new secreted bone matrix (H&E × 400).
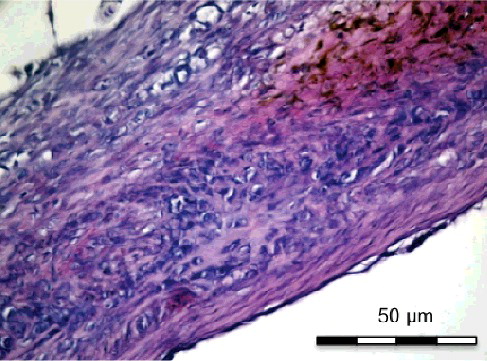
Figure 3. Group II-A with simvastatin. New bone formation with active connective tissue and simvastatin particles (H&E x 100).
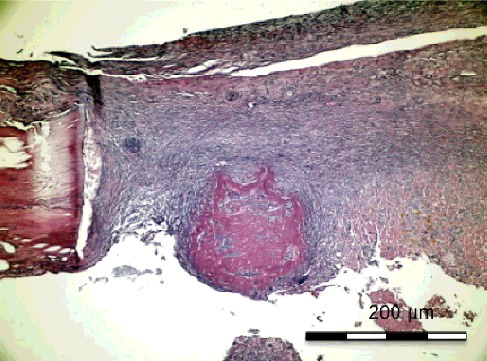
Figure 4. Group II-A without simvastatin. Young and old bleeding areas in the active connective tissue and osteoblastic differentiated young mesenchymal stem cells (H&E × 100).
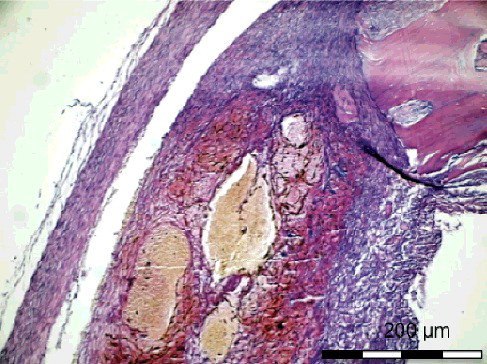
Table 3. Comparison of bone healing, inflammation, necrosis and fibrosis values on day 8.
On the 15th postoperative day, the histological sections () showed that the new bone formation at the site of the simvastatin-treated defect in Group I-B was significantly higher than that in Group II-B (p < 0.05). The new bone formation at the control defect in Group I-B was higher than that in Group II-B, although the difference was not significant. The inflammation scores were significantly lower in Group I-B than in Group II-B for both the simvastatin-treated and the control defects (p < 0.05). The necrosis score of the control defects was significantly lower (p < 0.05) in Group I-B than in Group II-B ().
Figure 5. Group I-B with simvastatin. Ice band like reticular bone formation between defect margins (H&E × 100).
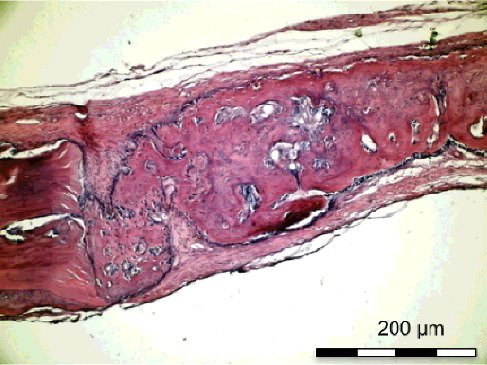
Figure 6. Group I-B without simvastatin. Bridge-like new bone formation covering the defect (H&E × 100)
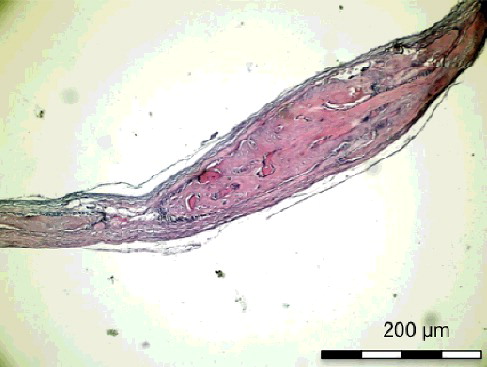
Figure 7. Group II-B with simvastatin. New bone trabecules filling the half of the defect around the simvastatin particles (H&E × 100).
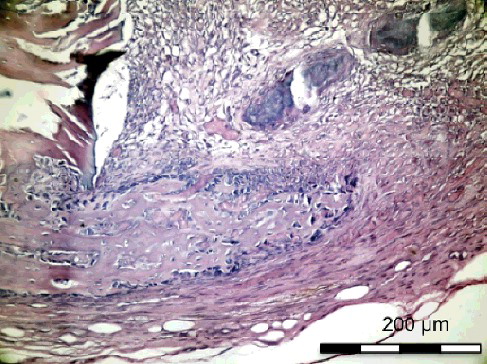
Table 4. Comparison of bone healing, inflammation, necrosis and fibrosis values on day 15.
The treatment of bone defects using biomaterials and tissue engineering methods has been extensively studied in dental practice. Recent studies demonstrated that local application of statins accelerates bone growth [Citation24,Citation25]. LED PBM has been shown to stimulate proliferation and differentiation of osteoblast precursor cells and consequently new bone formation [Citation26]. The present study was designed to determine the influence of PBM on bone healing in the rat calvarial defects filled with or without simvastatin solution impregnated gelatin sponge. To our knowledge, this is the first histological investigation on the use of the association of PBM and locally applied simvastatin.
Statins, HMG-CoA reductase inhibitors, can have pleiotropic effects on bone formation by up-regulating BMP-2 mRNA expression [Citation27]. Several studies have been performed to investigate the effects of systemic use of statins on bone healing but there are limited studies on local application. Simvastatin treatment reportedly has beneficial effects on bone mineral density (BMD) and bone turn-over and reduces the fracture risk in hypercholesterolemic postmenopausal women [Citation28,Citation29]. Most human studies are retrospective and although they found favourable results, statins cannot accumulate in bone sufficiently because of their high hepatic targeting property [Citation30]. This causes minimal effects after systemic administration of simvastatins. Less than 5% of an oral dose will reach the systemic circulation. High doses of systemic application can raise the risk of liver failure [Citation31]. This has prompted investigators to aim at local application of statins. In order to maximize their efficacy in skeletal tissues, the route of administration and dose formulation of statins could be further optimized. An alternative would be local administration of statins into defect sites, directly or by slow-release carrier systems [Citation32]. Retrospective animal studies have been carried out to objectively investigate the effect of statins on bone formation. Oxlund and Andreassen [Citation33] orally administered 40 mg/kg simvastatin solution daily by a gastric tube for 3 months on ovariectomized female rats. They concluded that simvastatin induces a moderate increase in cortical bone formation at the periosteal bone surface. Ayukawa et al. [Citation34] reported similar results around titanium implants in tibiae: the 10 mg/kg orally simvastatin administered group showed significantly greater values of bone density and bone contact ratio around the implants than the control group.
The stimulation of local bone formation is an important factor in the repair of isolated bone defects. To optimize the osteoinductive effect of existing statins, local administration is needed. Furthermore, local application helps preventing systemic side effects of drugs. Gutierrez et al. [Citation35] determined that topical application of statin was 50 times more active on bone formation in rats than oral administration.
Ezirganlı et al. [Citation22] created 8 mm CSDs on rat calvarium and locally applied 0.5–1–1.5 mg concentrated simvastatin solutions by gelatin sponge in experimental groups. They demonstrated that both the volume and the area of regenerated bone were higher in the experimental groups than in the control groups. The 0.5 mg simvastatin-treated group showed minimum inflammation rates compared to the others. The authors concluded that the local simvastatin application enhances the healing of the bone defects in the rat model CSDs [Citation22]. Nyan et al. [Citation36], Tylin et al. [Citation37] and Stein et al. [Citation25] reported similar results, in which local application of lower concentrations of simvastatin produced increased new bone formation with less tissue inflammation. Although the anti-inflammatory effects of statins have been reported [Citation25,Citation37], high-dose simvastatin induces inflammation around the site of local application. It has been shown that local simvastatin application at 2.2 mg causes inflammation and scabbing of the overlying skin on murine calvaria [Citation37]. Stein et al. [Citation25] concluded that reducing the simvastatin dose from 2.2 to 0.5 mg can decrease the signs of clinical inflammation. Similarly, Ezirganlı et al. [Citation22] reported less inflammation with local application of a single dose of 0.5 mg simvastatin on the defect area compared to 1 and 1.5 mg and control groups and claimed that 0.5 mg simvastatin is the optimal dose for a single local application. In our study, we chose a single dose 0.5 mg simvastatin and demonstrated that new bone formation was statistically higher at day 15 compared to that in the control areas. Additionally, the anti-inflammatory effect was significantly higher than in the other groups both on the 8th and the 15th day.
Recent studies have investigated the effect of the local application of simvastatin associated with different carriers such as methylcellulose gel [Citation25,Citation37], inorganic bovine bone graft, hydroxiapatite combined with calcium sulphate [Citation38], polylactic acid/polyglycolic acid copolymer [Citation39], calcium sulphate [Citation36,Citation40], lyophilized sponge [Citation41], collagen sponge [Citation42] and gelatin sponge [Citation22,Citation43]. However, there is no consensus about an ideal carrier of the drug for bone defects. In our study, we preferred to use collagen sponge because of its long shelf life, simple manipulation, cost effectiveness and slow release properties. In the experimental group, significantly higher new bone formation and lower inflammation values were observed than in control sites at day 15. These results demonstrated the high activity of the carrier in the defect area.
In the present study, the rate of bone regeneration was significantly higher in the simvastatin combined PBM group both at day 8 and day 15. Furthermore, enhanced bone healing was observed in the PBM group when compared with the simvastatin-treated and control groups, but no significant differences were detected. These findings may be indicative of an increased activity of irradiated osteoblasts in bone tissue. This can be explained by the high affinity of fibroblasts and osteoblasts to light, in the early stage of bone healing [Citation44].
LED devices produce non-coherent light and have fewer side-effects compared to LLL devices [Citation44]. LED therapy provides positive effects in several procedures. LED arrays do not generate heat during the therapy, therefore thermal injury is not expected, unlike with LLLs [Citation42]. Furthermore, LED therapy can be arranged by flat arrays allowing treatment of larger surface with lower cost compared to LLL therapy [Citation17,Citation18].
Studies on the effects of PBM therapy on bone regeneration have indicated that its effect on bone healing is related with the total dose of irradiation, duration and the irradiation mode. Different PBM protocols have been reported concerning time intervals and durations. Soares et al. [Citation3], Pinheiro et al. [Citation2] and Aciole et al. [Citation45] applied PBM at 48-h intervals during 2 weeks. On the other hand, Uysal et al. [Citation19] applied PBM at 24-h intervals for 10 consecutive days and Casalechi et al. [Citation46] preferred to apply PBM every 24-h for 7-, 14- and 21-day periods according to their study groups. These authors suggested that PBM significantly increases bone regeneration. In the present study, PBM was applied at 24-h intervals for 20 min per session for 7 and 14 consecutive days similarly to the protocol used by Deniz et al. [Citation44]. In conjunction with the previous studies, high bone regeneration values were obtained in the present study.
The biological effects of the PBM procedure remain to be elucidated. Visible light has been demonstrated to stimulate the action of cytochrome C oxidase in vivo and in vitro, a major photo acceptor molecule, causing increased production and stimulation of mitochondrial oxidative metabolism. Cytochrome C oxidase allows better functioning of cells which produces adenosine triphosphate (ATP). ATP accelerates mitose, improves bone repair mediates the production of fibroblasts and increases the peripheral blood circulation, improving the anti-inflammatory action in the early phase of tissue healing [Citation47]. In the present study, we focused on the effect of PBM on the bone regeneration rate during the early period of healing. Fracture healing occurs in four consecutive histologic phases: hematoma formation phase, early inflammatory phase (2–4 weeks), repair phase (within 1–2 months) and late remodelling phase [Citation48]. In the present study, we evaluated the effect of PBM on the early inflammatory phase, in which osteoblastic activity is just beginning with the formation of bone matrix. These osteoblasts were low in number and were not counted [Citation44]. A seven-point scoring method (modified Allen's Scoring System) was used to assess bone healing. We suggest that an additional latent period of time is needed to obtain more predictable results.
To obtain optimal biological results, it is necessary to identify the optimal light and experimental parameters. The wavelength delivered by the OsseoPulse® LED device in the present study was 625 nm with an output power of 20 mW/cm2. The energy density used in this study was 5 J/cm2, which was supported by several previous reports suggesting that 1–5 J/cm2 is effective to induce positive effects on both bone and soft tissues [Citation20,Citation49]. Our results showed increased formation of bone matrix stimulated by PBM in the early phase of the healing period.
The results from this study should be further evaluated with additional data from in vivo human trials to validate this hypothesis. Along this suggestion, the mechanical properties of the PBM treated and simvastatin grafted bone defect sites should be extended to contribute to a better understanding of the long-term clinical performance of this treatment modality.
Conclusions
The obtained results suggested that 0.5 mg simvastatin solution and LED light PBM with a wavelength of 625 nm might have a positive effect on the early phase of wound healing of bone defects individually. Combined application of simvastatin and LED light PBM therapy resulted in significantly higher new bone formation values than those observed by either individual treatment or in the control areas. The use of LED light PBM therapy with locally applied simvastatin solution could be suggested as a way of accelerating the bone tissue healing process in order to reduce the healing time.
Acknowledgements
The author woulld like to thanks Prof. Dr. Ertugrul Kilic for his generous support.
Disclosure statement
No potential conflict of interest was reported by the authors.
References
- Soares LG, Marques AM, Aciole JM, et al. Do laser/LED phototherapies influence the outcome of the repair of surgical bone defects grafted with biphasic synthetic microgranular HA + β-tricalcium phosphate? A Raman spectroscopy study. Lasers Med Sci. 2014;29(5):1575–1584.
- Pinheiro AL, Gerbi ME. Photoengineering of bone repair processes. Photomed Laser Surg. 2006;24:169–178.
- Soares LG, Marques AM, Barbosa AF, et al. Raman study of the repair of surgical bone defects grafted with biphasic synthetic microgranular HA C b-calcium triphosphate and irradiated or not with λ780 nm laser. Lasers Med Sci. 2014;29:1539–1550.
- Baba S, Inoue T, Hashimoto Y, et al. Effectiveness of scaffolds with pre-seeded mesenchymal stem cells in bone regeneration–assessment of osteogenic ability of scaffolds implanted under the periosteum of the cranial bone of rats. Dent Mater J. 2010;29(6):673–681.
- Arima Y, Uemura N, Hashimoto Y, et al. Evaluation of bone regeneration by porous alpha-tricalcium phosphate/atelocollagen sponge composite in rat calvarial defects. Orthodontic Waves. 2013;72:23–29.
- Pişkin E, Işoğlu IA, Bцlgen N, et al. In vivo performance of simvastatin-loaded electrospun spiral-wound polycaprolactone scaffolds inreconstruction of cranial bone defects in the rat model. J Biomed Mater Res A. 2009;90(4):1137–1151.
- Yin H, Li J, Yu X, et al. Effects of simvastatin on osseointegration in a canine total hip arthroplasty model: an experimental study. J Arthroplasty. 2011;26(8):1534–1539.
- Wong RW, Rabie AB. Early healing pattern of statin-induced osteogenesis. Br J Oral Maxillofac Surg. 2005;43:46–50.
- Ozeç I, Kiliç E, Gümüş C, et al. Effect of local simvastatin application on mandibular defects. J Craniofac Surg. 2007;18(3):546–550.
- Garrett IR, Gutierrez G, Mundy GR. Statins and bone formation. Curr Pharm Des. 2001;7(8):715–736.
- Chissas D, Stamatopoulos G, Verettas D, et al. Can low doses of simvastatin enhance fracture healing? An experimental study in rabbits. Injury. 2010;41(7):687–692.
- Uzzan B, Cohen R, Nicolas P, et al. Effects of statins on bone mineral density: a meta-analysis of clinical studies. Bone. 2007;40(6):1581–1587.
- Mundy G, Garrett R, Harris S, et al. Stimulation of bone formation in vitro and in rodents by statins. Science. 1999;286(5446):1946–1949.
- Brawn PR, Kwong-Hing A, Boeriu S, et al. Accelerated implant stability after LED photobiomodulation. J Dent Res. 2008;87:2021–2024.
- Aaboe M, Pinholt EM, Hjorting-Hansen E. Healing of experimentally created defects: a review. Br J Oral Maxillofac Surg. 1995;33(5):312–318.
- Sohn HM, Ko Y, Park M, et al. Comparison of the alendronate and irradiation with a light-emitting diode (LED) on murine osteoclastogenesis. Laser Med Sci. 2017;32(1):189–200.
- Desmet KD, Paz DA, Corry JJ, et al. Clinical and experimental applications of NIR-LED photo-biomodulation. Photomed Laser Surg. 2006;24:121–128.
- Whelan HT, Smits RL, Buchman EV, et al. Effect of NASA light-emitting diode irradiation on wound healing. J Clin Laser Med Surg. 2001;19:305–314.
- Uysal T, Ekizer A, Akcay H, et al. Resonance frequency analysis of orthodontic miniscrews subjected to light-emitting diode photobiomodulation therapy. Eur J Orthod. 2012;34(1):44–51.
- Brawn PR, Kwong-Hing A. Histologic comparison of light emitting diode phototherapy-treated hydroxyapatite - grafted extraction sockets: a same-mouth case study. Implant Dent. 2007;16(2):204–211.
- Fávaro–Pípi E, Ribeiro DA, Ribeiro JU, et al. Low-level laser therapy induces differential expression of osteogenic genes during bone repair in rats. Photomed Laser Surg. 2011;29(5):311–317.
- Ezirganlı Sç, Polat S, Barısç E, et al. Comparative investigation of the effects of different materials used with a titanium barrier on new bone formation. Clin Oral Implants Res. 2013;24(3):312–319.
- Allen HL, Wase A, Bear WT. Indomethacin and asprin: effect of nonsteroidal anti-inflammatory agents on the rate of fracture repair in rat. Acta Orthop. 1980;51:595–600.
- Wong RW, Rabie AB. Statin collagen grafts used to repair defects in the parietal bone of rabbits. Br J Oral Maxillofac Surg. 2003;41(4):244–248.
- Stein D, Lee Y, Schmid MJ, et al. Local simvastatin effects on mandibular bone growth and inflammation. J Periodontol. 2005;76(11):1861–1870.
- Ozawa Y, Shimizu N, Kariya G, et al. Low-energy laser irradiation stimulates bone nodule formation at early stages of cell culture in rat calvarial cells. Bone. 1998;22(4):347–354.
- Sukul M, Min YK, Lee SY, et al. Osteogenic potential of simvastatin loaded gelatin-nanofibrillar cellulose-β tricalcium phosphate hydrogel scaffold in critical-sized rat calvarial defect. EurPolym J. 2015;73:308–323.
- Montagnani A, Gonnelli S, Cepollaro C, et al. Effect of simvastatin treatment on bone mineral density and bone turnover in hypercholesterolemic postmenopausal women: a 1-year longitudinal study. Bone. 2003;32(4):427–433.
- Chan KA, Andrade SE, Boles M, et al. Inhibitors of hydroxymethylglutaryl-coenzyme A rductase and risk of fractures among older women. Lancet. 2000;355:2185–2188.
- Rogers. MJ. Statins: lower lipids and better bones? Nat Med. 2000;6:21–23.
- Guyton JR. Benefit versus risk in statin treatment. Am J Cardiol. 2006;97:95–97.
- Monjo M, Rubert M, Wohlfahrt JC, et al. In vivo performance of absorbable collagen sponges with rosuvastatin in critical-size cortical bone defects. Acta Biomater. 2010;6(4):1405–1412.
- Oxlund H, Andreassen TT. Simvastatin treatment partially prevents ovariectomy induced bone loss while increasing cortical boneformation. Bone. 2004;34(4):609–618.
- Ayukawa Y, Okamura A, Koyano K. Simvastatin promotes osteogenesis around titanium implants. Clin Oral Implant Res. 2004;15(3):346–350.
- Gutierrez GE, Lalka D, Garrett IR, et al. Transdermal application of lovastatin to rats causes profound increases in bone formation and plasma concentrations. Osteoporos Int. 2006;17(7):1033–1042.
- Nyan M, Sato D, Oda M, et al. Bone formation with the combination of simvastatin and calcium sulfate in critical-sized rat calvarial defect. J Pharmacol Sci. 2007;104(4):384–386.
- Thylin MR, McConnell JC, Schmid MJ, et al. Effects of simvastatin gels on murine calvarial bone. J Periodontol. 2002;73(10):1141–1148.
- Papadimitriou K, Karkavelas G, Vouros I, et al. Effects of local application of simvastatin on bone regeneration in femoral bone defects in rabbit. J Craniomaxillofac Surg. 2015;43(2):232–237.
- Wu Z, Liu C, Zang G, et al. The effect of simvastatin on remodelling of the alveolar bone following tooth extraction. Int J Oral Maxillofac Surg. 2008;37(2):170–176.
- Nyan M, Sato D, Kihara H, et al. Effects of the combination with alpha-tricalcium phosphate and simvastatin on bone regeneration. Clin Oral Implants Res. 2009;20:280–287.
- Ibrahim HK, Fahmy RH. Localized rosuvastatin via implantable bioerodible sponge and its potential role in augmenting bone healing and regeneration. 2016;23(9):3181–3192.
- Santana WM, Sousa DN, Ferreira VM, et al. Simvastatin and biphasic calcium phosphate affects bone formation in critical-sized rat calvarial defects. Acta Cir Bras. 2016;31(5):300–307.
- Özeç İ, Kılıç E, Gümüsç C, et al. Lokal olarak üç farklı dozda simvastatin uygulamasının kemik defekti iyilesçmesi üzerine etkisinin değerlendirilmesi [ Evaluatıon of effects of three different doses of local simvastatin applicatıon on healing of bone defects]. Cumhuriyet Üniv Disç Hekim Fak Der. 2007;2(10):82–86. Turkish.
- Deniz E, Arslan A, Diker N, et al. Evaluation of light-emitting diode photobiomodulation on bone healing of rat calvarial defects. Biotechnol Biotechnol Equip. 2015;29(4):758–765.
- Aciole JM, de Castro IC, Soares LG, et al. Assessment of the LED phototherapy on femoral bone defects of ovariectomized rats: a Raman spectral study. Lasers Med Sci. 2014;29:1269–1277.
- Casalechi HL, Nicolau RA, Casalechi VL, et al. The effects of low-level light emitting diode on the repair process of Achilles tendon therapy in rats. Lasers Med Sci. 2009;24:659–665.
- Pauly S, Luttosch F, Morawski M, et al. Simvastatin locally applied from a biodegradable coating of osteosynthetic implants improves fracture healing comparable to BMP-2 application. Bone. 2009;45(3):505–511.
- Refai H, Radwan D, Hassanien N. Radiodensitometric assessment of the effect of pulsed electromagnetic field stimulation versus low ıntensity laser ırradiation on mandibular fracture repair: a preliminary clinical trial. J Maxillofac Oral Surg. 2014;13(4):451–457.
- Márquez Martínez ME, Pinheiro AL, Ramalho LM. Effect of IR laser photobiomodulation on the repair of bone defects grafted with organic bovine bone. Lasers Med Sci. 2008;23(3):313–317.