ABSTRACT
Although the number of sequenced plant genomes has been rapidly increasing, many plant proteins in public databases are recognized as proteins with domains of unknown function (DUF). The domain of unknown function DUF829 family consists of plant proteins with an average length of around 220 residues. In this study, we attempted to uncover the biological functions of four DUF829 members (OsDUF829.1–OsDUF829.4) in rice Nipponbare. We classified these proteins into three groups (group I, II and III) using phylogenetic analysis. Real time polymerase chain reaction (PCR) showed that the expression patterns of the four OsDUF829 family members were different in 15 different rice tissues. Under salt and heat stress conditions, the expression level of OsDUF829.2 and OsDUF829.4 was significantly elevated. Overexpression of OsDUF829.2 and OsDUF829.4 in Escherichia coli significantly improved the resistance to salt stress. The obtained results provide important implications for further functional studies of the OsDUF829 family in rice.
KEYWORDS:
Introduction
Plants are susceptible to various abiotic stresses, including drought, salinity and extreme temperatures, during different growth and development periods. These abiotic stress conditions can exert strong negative effects on the productivity and quality of plants in agriculture [Citation1]. To counteract these negative effects, plants have developed a number of physiological and biochemical strategies to preclude detrimental impacts from specific stress [Citation2–7]. Salinity, which can lead to the disruption of homeostasis and ion distribution in plant cells, is generally considered as one of the important osmotic stress factors [Citation8–10]. The molecular responses of plants to salinity stress are controlled by abscisic acid (ABA) [Citation11,Citation12], reactive oxygen species (ROS) [Citation13–15], ion transport [Citation16,Citation17] and some transcription factors [Citation18].
It is urgent to determine the molecular mechanisms involved in the salt stress response of plants. At present, some genes have been used to enhance salt tolerance in rice, and certain effects have been achieved [Citation19–25], however, the number of such genes still remains rather small.
The domain of unknown function (DUF) is a protein domain with unknown function. The names of DUFs are given when protein families have no functional annotation in the Pfam database (http://pfam.xfam.org/family) [Citation26,Citation27]. In recent years, it has been reported that some DUF-containing proteins could enhance the tolerance to stresses in plants [Citation24,Citation28–31]. The DUF829 family consists of many plant proteins of unknown function and has an average length of around 220 residues. However, so far the OsDUF829 family has not been functionally characterized in rice.
In this study, we analyzed the protein sequence of the OsDUF829 family in rice, their spatio-temporal expression profile and their expression pattern under various stresses and ABA treatment. Furthermore, in order to better understand the function of OsDUF829.2 and OsDUF829.4, we overexpressed these genes in Escherichia coli.
Materials and methods
Database searches
The sequences of putative OsDUF829 family proteins in rice were obtained by searching the rice genome annotation project (RGAP version 7) database (http://rice.plantbiology.msu.edu/) with domain number PF05705. The corresponding protein sequences of these genes were then downloaded from RGAP and confirmed with the SMART database (http://smart.embl-heidelberg.de/smart/batch.pl) [Citation32,Citation33]. If there were several gene models at one locus, only the complete gene model was selected for further sequence analysis.
Sequence analysis
For each OsDUF829 gene, information about the chromosomal localization, amino acid (aa) length and full length cDNA accessions was obtained from RGAP and the Knowledge-Based Oryza Molecular Biological Encyclopedia (KOME) (http://cdna01.dna.affrc.go.jp/cDNA). The protein localizations of OsDUF829 members were predicted using online protein location prediction software TargetP 1.1 (http://www.cbs.dtu.dk/services/TargetP/) [Citation34]. MEME version 4.11.2 (http://meme.nbcr.net/meme/) was used to predict the motifs of OsDUF829 members [Citation35]. The alignment of the aa sequences of OsDUF829 family members was done using Clustal Omega [Citation36]. A phylogenetic tree of DUF829 members in rice and Arabidopsis was constructed using MEGA4 and Bootstrap testing was performed with 1,000 resamplings [Citation37].
Plant growth and various stress and ABA treatment
Rice (Oryza sativa L. subsp. japonica cv. Nipponbare) seeds were germinated for 3 days. The resulted seedlings were grown hydroponically in a 30-L vessel containing nutrient solution as described previously [Citation38]. At the emergence of the fourth leaf, the seedlings were subjected to various stresses and ABA treatment as described previously [Citation39,Citation40].
RNA extraction and real-time polymerase chain reaction (PCR)
Samples were collected form 15 tissues: leaf blade at the four-leaf stage, leaf blade from plants with four tillers, leaf blade at ripening stage, leaf sheath at four-leaf stage, leaf sheath from plants with four tillers, root at the four-leaf stage, root from plants with four tillers, stem from plants with four tillers, stem at the ripening stage, 1.2–1.5 mm anther, pistil from 10 to 14 cm inflorescence, embryo at 7 days and 28 days after flowering and endosperm at 7 days and 28 days after flowering. The samples were ground to fine powder in liquid nitrogen.
Total RNA was extracted with Trizol reagent (GIBCO, Burlington, ON, USA) according to the manufacturer's instructions. Next, the total RNA was treated with RNase-free DNase I (Invitrogen, Carlsbad, CA, USA) for 15 min to degrade any potential DNA contamination, and then used for first-strand cDNA synthesis. Real-time PCR was performed on an optical 96-well plate with a Bio-Rad CFX96 (Cambridge, MA, USA) real-time PCR system. Each reaction contained 5 µL of 2 × SYBR Green Master Mix Reagent (Roche, Indianapolis, IN, USA), 1 µL of cDNA samples and 200 nmol/L gene-specific primer in a final volume of 10 µL. The thermal cycle used was as follows: 95 °C for 10 min, followed by 39 cycles of 95 °C for 10 s, 60 °C for 30 s and 72 °C for 1 min. The rice Actin1 gene (LOC_Os03g50885) was used as an internal control with primers 5'-TGGCATCTCTCAGCACATTC-3' and 5'-TGCACAATGGATGGGCCAGA-3'. The primers used in the OsDUF829 gene family members’ expression analysis are listed in Table S1 in the Supplementary Appendix. Data analyses of the relative expression levels with the 2–△△Ct method were performed as described previously [Citation41].
Assay for salt stress tolerance of E. coli transformants
Primers used to construct the expression vector pET32a-OsDUF829.2 and pET32a-OsDUF829.4 are listed in Table S2 in the Supplementary Appendix. The amplified products were cloned into a pET32a vector at the BamHI-HindIII site to express the fusion protein that contains a Trx•Tag™ thioredoxin at the N-terminus [Citation42]. The transformed E. coli Rosetta cells (Sanborn, MN, USA) were grown in Luria–Bertani (LB) liquid medium containing 100 µg/mL of ampicillin at 37 °C overnight, then they were inoculated into fresh LB medium (1:100 dilution) supplemented with ampicillin (100 µg/mL) to be incubated until the exponential growth phase (optical density at 600 nm (OD600) of 0.5–0.6). Isopropylthio-β-D-galactoside (IPTG) was added into the cultures to induce the expression of the transformed gene. For the salt tolerance assay, the growth curve of E. coli transformants was plotted. The OD values of pET-32a, pET32a-OsDUF829.2 and pET32a-OsDUF829.4 cells were measured at 0, 2 h, 4 h, 6 h, 8 h, 10 h, 12 h, 14 h, 16 and 18 h under normal conditions and 200 mmol/L NaCl treatment.
Data analysis
The experiments were repeated three times. Data are mean values from three independent experiments, with standard error of the means. Statistical analysis was performed using the Student's t-test by EXCEL version 2003.
Results and discussion
Identification of OsDUF829 family members in rice
Four genes encoding OsDUF829 family proteins were identified () by searching the rice genome annotation project with domain number PF05705. The presence of these OsDUF829 members in rice genome was then confirmed with the SMART database (Figure S1 in the Supplementary Appendix). These genes were named OsDUF829.1 to OsDUF829.4 according to their positions on pseudomolecules. These genes are distributed on chromosome 2 (OsDUF829.1), 6 (OsDUF829.2 and OsDUF829.3) and 11 (OsDUF829.4).
Table 1. OsDUF829 genes and the properties of the putative proteins they encode.
As the rice genome includes 10 duplicated blocks, accounting for 45% of its total size [Citation43], it is possible that genome duplication may be the mechanism that underlies the distribution of OsDUF829 family members in different chromosomes, similar to that previously suggested for OsDUF866 and OsDUF946 members [Citation39,Citation40].
Sequence analysis of OsDUF829 family proteins
Information about OsDUF829 family members, including amino acid length, full length cDNA accessions and subcellular localization, is presented in . The length of OsDUF829 proteins varies from 381 (OsDUF829.1) to 406 (OsDUF829.2) amino acids. In order to identify sequences conserved among the four OsDUF829 members, the MEME motif search tool (http://meme.sdsc.edu/meme/) was applied and three distinct motifs were determined (A). Motif 1 and motif 2 were found in all four OsDUF829 members, whereas motif 3 was present only in OsDUF829.2 and OsDUF829.4 (B).
Figure 1. Conservative structural analysis of rice OsDUF829 family. (A) Moif 1, motif 2, and motif 3 were conserved motifs in rice OsDUF829 family obtained by MEME. (B) Distribution of conserved motifs in OsDUF829 proteins identified by MEME software.
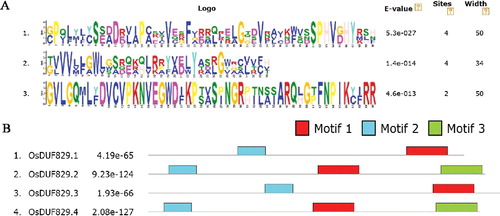
The multiple sequence alignment analysis of the OsDUF829 members was performed using Clustal Omega (Figure S2 in the Supplementary Appendix). The percent identity was 22.14% for OsDUF829.1 and OsDUF829.2, 42.43% for OsDUF829.1 and OsDUF829.3, 20.70% for OsDUF829.1 and OsDUF829.4, 20.44% for OsDUF829.2 and OsDUF829.3, 54.27% for OsDUF829.2 and OsDUF829.4 and 21.61 for OsDUF829.3 and OsDUF829.4. To examine the evolutionary relationships among the putative OsDUF829 family members in rice and Arabidopsis, a phylogenetic analysis was performed using MEGA4. Here four Arabidopsis DUF829 members, AT2G15695.1 (located in the nucleus), AT2G18245.1 (located in plastids), AT3G19970.1 (located in plastids) and AT5G44250.1 (located in peroxisomes), were included as reference sequences. The results indicated that the DUF829 family members can be classified into three major groups (I, II and III) (). Group I is composed of four members (OsDUF829.2, OsDUF829.4, AT2G15695.1 and AT5G44250.1), group II consists of two members (OsDUF829.1 and AT2G18245.1), and group III includes two members (OsDUF829.3 and AT3G19970.1). Interestingly, OsDUF829.2 and OsDUF829.4, which have the highest sequence similarity, also have many similar features. For example, they both belong to group I and they both were predicted to be located in the mitochondria.
Expression patterns of OsDUF829
The expression patterns of OsDUF829 family members in various rice tissues still remain poorly understood. In this study, the expression of the four OsDUF829 protein-encoding genes in 15 rice tissues was examined by real time PCR. Under normal conditions, the four members of OsDUF829 showed different expression profile (). For OsDUF829.1, the highest expression was in the pistil from 10 to 14 cm inflorescence, whereas the lowest expression was in the leaf sheath from plants with four tillers. For OsDUF829.2, the highest expression was in the leaf blade at the four-leaf stage, whereas the lowest expression was also in the endosperm at 28 days after flowering. For OsDUF829.3, the highest expression was in the leaf blade at the four-leaf stage, whereas the lowest expression was in the leaf sheath from plants with four tillers. For OsDUF829.4, the highest expression was in the leaf blade from plants with four tillers, whereas the lowest expression was in the leaf sheath from such plants. According to the different expression patterns of OsDUF829 family members (), we speculated that they may play distinct roles in different tissues in rice.
Figure 3. Real time PCR analysis of OsDUF829 genes in different tissues of Nipponbare rice. Fifteen representative tissues are as follows: Lb1, leaf blade at four-leaf stage; Lb2, leaf blade from plants with four tillers; Lb3, leaf blade at ripening stage; Ls1, leaf sheath at four-leaf stage; Ls2, leaf sheath from plants with four tillers; Rt1, root at four-leaf stages; Rt2, root from plants with four tillers; St1, stem from plants with four tillers; St2, stem at ripening stage; An, 1.2–1.5 mm anther; Pi, pistil from 10 to 14 cm inflorescence; Em1, embryo at 7 days after flowering; Em2, embryo at 28 days after flowering; En1, endosperm at 7 days after flowering; En2, endosperm at 28 days after flowering.
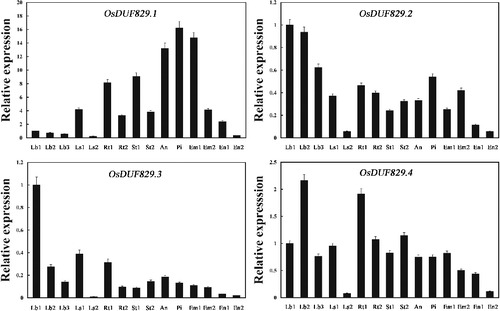
The expression of OsDUF829 family members under various stress conditions and ABA treatment may help elucidate their functions; however, little is known about it thus far. Therefore, we performed real-time PCR to examine the expression of OsDUF829 family genes under various stress conditions (). The expression of OsDUF829.1 was significantly (P < 0.05) upregulated under heat conditions and the expression of OsDUF829.3 was significantly (P < 0.05) upregulated under both drought and cold conditions. Under salt and heat conditions, the expression level of OsDUF829.2 and OsDUF829.4 was significantly (P < 0.05) elevated. The results indicated that OsDUF829 family members play different roles in response to various stress.
OsDUF829.2 and OsDUF829.4 improved salt resistance in transgenic E. coli
Since the expression level of OsDUF829.2 and OsDUF829.4 was significantly elevated under salt stress conditions, we hypothesized that they may be involved in the response and resistance to salt stress. To test this hypothesis, we overexpressed these genes in E. coli and examined the salt stress resistance based on their growth curves.
The OD values of pET-32a, pET32a-OsDUF829.2 and pET32a-OsDUF829.4 were measured at 0–18 h under normal conditions and 200 mmol/L NaCl treatments (). Under normal conditions, there was no evident difference in the growth rate between transgenic lines (E. coli transformed with pET32a-OsDUF829.2 and pET32a-OsDUF829.4) and the control (E. coli transformed with pET-32a). Under salt conditions, the growth rate in E. coli transformed with pET32a-OsDUF829.2 (A) and pET32a-OsDUF829.4 (B) was much higher than that of the control from 2 to 18 h. This result indicated that the overexpression of OsDUF829.2 and OsDUF829.4 in E. coli may significantly improve the resistance to salt.
Figure 5. Growth of E. coli recombinants overexpressing OsDUF829.2 (A) and OsDUF829.4 (B) under normal and salt conditions.
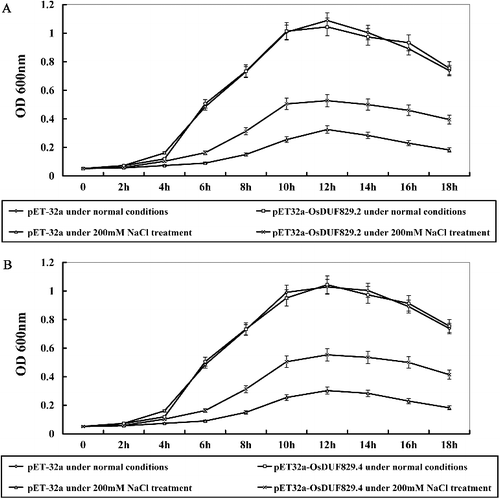
Production of reactive oxygen species (ROS) is a frequent event in organisms when they suffer from abiotic stresses, including salinity [Citation44,Citation45]. ROS production can cause oxidative damage on membrane lipids and perturbation of cell membrane function [Citation46]. It is therefore of high importance to maintain the balance between ROS generation and ROS scavenging in cells subjected to abiotic stresses [Citation47]. In this study, OsDUF829.2 and OsDUF829.4 could improve salt resistance in transgenic E. coli. At present, the specific mechanism by which salt tolerance is enhanced in E. coli recombinants is unclear. It is possible that the salt stress signal is strengthened in E. coli recombinants transformed with OsDUF829.2 and OsDUF829.4, which in turn promotes the expression of the downstream stress-related genes, including genes encoding ROS scavenging enzymes. As a result, the salt tolerance in transgenic E. coli was improved.
The functions of some DUF proteins have been elucidated recently. TBL3 (TRICHOME BIREFRINGENCE-like3), encoding a protein with a DUF231 domain, contributes to the synthesis and deposition of secondary wall cellulose [Citation48]. Some DUF724 gene family members in Arabidopsis may be involved in the polar growth of plant cells via transportation of RNAs [Citation49]. Several DUF784 genes are involved in Arabidopsis embryo sac development [Citation50] and some DUF1218 family members have been implicated in several aspects of cell wall biology [Citation51]. In our previous study, OsDUF866.1 improved the heat stress resistance [Citation39] and OsDUF946.4 improved the tolerance to salt and drought in transgenic E. coli [Citation40]. In this study, OsDUF829.2 and OsDUF829.4 improved the salt resistance in transgenic E. coli. DUF-containing proteins can play different roles in plants.
Conclusions
This study provides not only important sequence information regarding the OsDUF829 family members, but also showed the expression patterns of OsDUF829 family members in different tissues, and under various abnormal conditions including abiotic stress and ABA-treatment. Furthermore, this study suggested that OsDUF829.2 and OsDUF829.4 can improve the resistance to salt stresses in transgenic E. coli. These data can provide important reference for further studies of the functions of OsDUF829 family.
Supplemental_Data.pdf
Download PDF (359.8 KB)Disclosure statement
No potential conflict of interest was reported by the authors.
Additional information
Funding
References
- Boyer JS. Plant productivity and environment. Science. 1982;218:443–448.
- Ingram J, Bartels D. The molecular basis of dehydration tolerance in plants. Annu Rev Plant Physiol Plant Mol Biol. 1996;47:377–403.
- Pastori GM, Foyer CH. Common components, networks, and pathways of cross-tolerance to stress. The central role of “redox” and abscisic acid-mediated controls. Plant Physiol. 2002;129:460–468.
- Zhu JK. Salt and drought stress signal transduction in plants. Annu Rev Plant Biol. 2002;53:247–273.
- Wang W, Vinocur B, Altman A. Plant responses to drought, salinity and extreme temperatures: towards genetic engineering for stress tolerance. Planta. 2003 [cited 2017 Sep 15];218:1. [17 p] DOI: 10.1007/s00425-003-1105-5.
- Xiang Y, Tang N, Du H, et al. Characterization of OsbZIP23 as a key player of the basic leucine zipper transcription factor family for conferring abscisic acid sensitivity and salinity and drought tolerance in rice. Plant Physiol. 2008;148:1938–1952.
- Bohnert HJ, Gong Q, Li P, et al. Unraveling abiotic stress tolerance mechanisms–getting genomics going. Curr Opin Plant Biol. 2006;9:180–188.
- Zhu JK. Plant salt tolerance. Trends Plant Sci. 2001;6:66–71.
- Serrano R, Rodriguez-Navarro A. Ion homeostasis during salt stress in plants. Curr Opin Cell Biol. 2001;13:399–404.
- Zhu JK. Regulation of ion homeostasis under salt stress. Curr Opin Plant Biol. 2003;6:441–445.
- Fujii H, Zhu JK. Osmotic stress signaling via protein kinases. Cell Mol Life Sci. 2012;69:3165–3173.
- Park SY, Fung P, Nishimura N, et al. Abscisic acid inhibits type 2C protein phosphatases via the PYR/PYL family of START proteins. Science. 2009;324:1068–1071.
- Kwak JM, Mori IC, Pei ZM, et al. NADPH oxidase AtrbohD and AtrbohF genes function in ROS-dependent ABA signaling in Arabidopsis. EMBO J. 2003;22:2623–2633.
- Ma L, Zhang H, Sun L, et al. NADPH oxidase AtrbohD and AtrbohF function in ROS-dependent regulation of Na(+)/K(+)homeostasis in Arabidopsis under salt stress. J Exp Bot. 2012;63:305–317.
- Pei ZM, Murata Y, Benning G, et al. Calcium channels activated by hydrogen peroxide mediate abscisic acid signalling in guard cells. Nature. 2000;406:731–734.
- Sutter JU, Sieben C, Hartel A, et al. Abscisic acid triggers the endocytosis of the arabidopsis KAT1 K+ channel and its recycling to the plasma membrane. Curr Biol. 2007;17:1396–1402.
- Zhang X, Miao YC, An GY, et al. K+ channels inhibited by hydrogen peroxide mediate abscisic acid signaling in Vicia guard cells. Cell Res. 2001;11:195–202.
- Fujita Y, Fujita M, Shinozaki, K, et al. ABA-mediated transcriptional regulation in response to osmotic stress in plants. J Plant Res. 2011;124:509–525.
- Yu Y, Cui YC, Ren C, et al. Transgenic rice expressing a cassava (Manihot esculenta Crantz) plasma membrane gene MePMP3-2 exhibits enhanced tolerance to salt and drought stresses. Gen Mol Res. 2016 [cited 2017 Sep 15];15(1):1–16. DOI: 10.4238/gmr.15017336.
- Wang C, Yang Y, Wang H, et al. Ectopic expression of a cytochrome P450 monooxygenase gene PtCYP714A3 from Populus trichocarpa reduces shoot growth and improves tolerance to salt stress in transgenic rice. Plant Biotechnol J. 2016;14:1838–1851.
- Liu Y, Sun J, Wu Y. Arabidopsis ATAF1 enhances the tolerance to salt stress and ABA in transgenic rice. J Plant Res. 2016;129:955–962.
- Jing P, Zou J, Kong L, et al. OsCCD1, a novel small calcium-binding protein with one EF-hand motif, positively regulates osmotic and salt tolerance in rice. Plant Sci. 2016;247:104–114.
- Hong Y, Zhang H, Huang L, et al. Overexpression of a stress-responsive NAC transcription factor gene ONAC022 improves drought and salt tolerance in rice. Front Plant Sci. 2016 [cited 2017 Sep 15];7:4. DOI: 10.3389/fpls.2016.00004.
- Guo C, Luo C, Guo L, et al. OsSIDP366, a DUF1644 gene, positively regulates responses to drought and salt stresses in rice. J Integr Plant Biol. 2016;58:492–502.
- Dou M, Fan S, Yang S, et al. Overexpression of AmRosea1 gene confers drought and salt tolerance in rice. Int J Mol Sci. 2016 [cited 2017 Sep 15];18:2. DOI:10.3390/ijms18010002
- Bateman A, Coggill P, Finn RD. DUFs: families in search of function. Acta Crystallogr Sect F Struct Biol Cryst Commun. 2010;66:1148–1152.
- Finn RD, Coggill P, Eberhardt RY, et al. The Pfam protein families database: towards a more sustainable future. Nucleic Acids Res. 2016;44:279–285.
- He X, Hou X, Shen Y, et al. TaSRG, a wheat transcription factor, significantly affects salt tolerance in transgenic rice and Arabidopsis. FEBS Lett. 2011;585:1231–1237.
- Kim SJ, Ryu MY, Kim WT. Suppression of Arabidopsis RING-DUF1117 E3 ubiquitin ligases, AtRDUF1 and AtRDUF2, reduces tolerance to ABA-mediated drought stress. Biochem Biophys Res Commun. 2012;420:141–147.
- Luo C, Guo C, Wang W, et al. Overexpression of a new stress-repressive gene OsDSR2 encoding a protein with a DUF966 domain increases salt and simulated drought stress sensitivities and reduces ABA sensitivity in rice. Plant Cell Rep. 2014;33:323–336.
- Wang L, Shen R, Chen LT, et al. Characterization of a novel DUF1618 gene family in rice. J Integr Plant Biol. 2014;56:151–158.
- Schultz J, Milpetz F, Bork P, et al. SMART, a simple modular architecture research tool: identification of signaling domains. Proc Natl Acad Sci U S A. 1998;95:5857–5864.
- Letunic I, Doerks T, Bork P. SMART: recent updates, new developments and status in 2015. Nucleic Acids Res. 2014;43:257–260.
- Emanuelsson O, Brunak S, von Heijne G, et al. Locating proteins in the cell using TargetP, SignalP and related tools. Nat Protoc. 2007;2:953–971.
- Bailey TL, Boden M, Buske FA, et al. MEME SUITE: tools for motif discovery and searching. Nucleic Acids Res. 2009;37:202–208.
- Sievers F, Wilm A, Dineen D, et al. Fast, scalable generation of high-quality protein multiple sequence alignments using Clustal Omega. Mol Syst Biol. 2011 [cited 2017 Sep 15];7:539. DOI: 10.1038/msb.2011.75.
- Tamura K, Dudley J, Nei M, et al. MEGA4: Molecular Evolutionary Genetics Analysis (MEGA) software version 4.0. Mol Biol Evol. 2007;24:1596–1599.
- Li L, Liu C, Lian X. Gene expression profiles in rice roots under low phosphorus stress. Plant Mol Biol. 2010;72:423–432.
- Li L, Xie C, Ye T, et al. Molecular characterization, expression pattern, and function analysis of the rice OsDUF866 family. Biotechnol Biotechnol Equip. 2017;31:243–249.
- Li L, Ye T, Xu J, et al. Molecular characterization and function analysis of the rice OsDUF946 family. Biotechnol Biotechnol Equip. 2017;31:477–485.
- Livak KJ, Schmittgen TD. Analysis of relative gene expression data using real-time quantitative PCR and the 2(-Delta Delta C(T)) Method. Methods. 2001;25:402–408.
- LaVallie ER, DiBlasio EA, Kovacic S, et al. A thioredoxin gene fusion expression system that circumvents inclusion body formation in the E. coli cytoplasm. Biotechnology (NY). 1993;11:187–193.
- Wang X, Shi X, Hao B, et al. Duplication and DNA segmental loss in the rice genome: implications for diploidization. New Phytol. 2005;165:937–946.
- Miller G, Shulaev V, Mittler R. Reactive oxygen signaling and abiotic stress. Physiol Plant. 2008;133:481–489.
- Mittler R. Oxidative stress, antioxidants and stress tolerance. Trends Plant Sci. 2002;7:405–410.
- Gechev TS, Van Breusegem F, Stone JM, et al. Reactive oxygen species as signals that modulate plant stress responses and programmed cell death. Bioessays. 2006;28:1091–1101.
- Mittler R, Vanderauwera S, Gollery M, et al. Reactive oxygen gene network of plants. Trends Plant Sci. 2004;9:490–498.
- Bischoff V, Nita S, Neumetzler L, et al. TRICHOME BIREFRINGENCE and its homolog AT5G01360 encode plant-specific DUF231 proteins required for cellulose biosynthesis in Arabidopsis. Plant Physiol. 2010;153:590–602.
- Cao X, Yang KZ, Xia C, et al. Characterization of DUF724 gene family in Arabidopsis thaliana. Plant Mol Biol. 2010;72:61–73.
- Jones-Rhoades MW, Borevitz JO, Preuss D. Genome-wide expression profiling of the Arabidopsis female gametophyte identifies families of small, secreted proteins. PLoS Genet. 2007;3:1848–1861.
- Persson S, Wei H, Milne J, et al. Identification of genes required for cellulose synthesis by regression analysis of public microarray data sets. Proc Natl Acad Sci U S A. 2005;102:8633–8638.