ABSTRACT
Periodontal tissue engineering is a possible strategy for regeneration of human periodontal ligaments (PDLs) around dental implants. The aim of this study was to investigate the feasibility of three-dimensional polyglycolic acid (PGA) fibre mesh as a scaffold for human PDL cells in periodontal tissue engineering with a nude mouse model. Human PDL cells at a density of 2 × 107/mL were seeded onto porous PGA scaffolds. After seven days of incubation in vitro, the PGA–cell constructs and cell-free scaffolds were subcutaneously implanted on the back of BALB/c-nu mice bilaterally. The mice were sacrificed in batches at 2, 4, 6 and 8 weeks after implantation, and the harvests were examined histologically. In our study, PGA scaffolds promoted mRNA expression of collagen type I, collagen type III and fibronectin in PDL cells. Masson's trichrome staining showed that after two weeks, the implants were well vascularised in vivo. Fluorescence microscopy indicated that the newly formed tissues were derived from the GFP-labelled human PDL cells. Our study suggested that the delivery of PDL cells via a non-woven PGA mesh might serve as a viable approach to promote periodontal tissue regeneration.
Introduction
To date, the superior solution for tooth loss caused by periodontitis or trauma is dental implant. Owing to the absence of periodontal ligament (PDL) tissue – specialised connective tissue that connects the cementum and alveolar bone to maintain tooth in situ and preserve tissue homeostasis [Citation1,Citation2] – some complications including ankylosis and root resorption may occur [Citation3]. In the last two decades, a number of regenerative procedures have been proposed, including guided tissue regeneration, bone grafting and enamel matrix derivatives; however, complete regeneration has hardly been observed [Citation4,Citation5].
Tissue engineering, which has developed into an interdisciplinary field, provides a new paradigm for tissue regeneration [Citation6,Citation7]. A recent study reported that the seeding of PDL cells or dental pulp cells on nano-hyroxyapatite-coated silk scaffolds could promote periodontal regeneration in dogs [Citation8]. Liu et al. [Citation9] demonstrated that the autologous PDL stem cell-mediated treatment resulted in the recovery of the heights of alveolar bone on a minipig model.
Accordingly, the present study was focused on the application of tissue engineering to PDL regeneration. The key to regenerate new PDL tissue is to provide the repair site with sufficient progenitor cells in a suitable delivery vehicle. Seo et al. [Citation10] concluded that PDL contains heterogeneous cell populations that are not terminally differentiated and have the capacity to differentiate into cells of different phenotypes, including osteoblasts, cementoblasts, chondrocytes, adipocytes and neurons. Consequently, PDL cells have been a good candidate in regenerating periodontal tissue. The biodegradable polymer family has been regarded as a type of accessible scaffold. Among them, polyglycolic acid (PGA) scaffold materials are optimal with respect to their easy processing and adjustable degradation rates. They have been widely used in business and clinical applications such as degradable sutures, which have been approved by the US Food and Drug Administration [Citation11]. In addition, a recent study has demonstrated that PGA did not cause any significant harmful side effects such as remarkable inflammation during six weeks of healing in experimental dogs [Citation12].
In light of these findings, we implemented this study with the hypothesis that it is possible to regenerate PDL tissue around dental implants by culturing and propagating PDL cells in vitro, and then delivering them with three-dimensional PGA scaffolds in vivo.
Materials and methods
Isolation and culture of human periodontal ligament cells
PDL cells were isolated and cultured as previously described [Citation13]. Clinically healthy canine or premolar teeth were extracted from orthodontic patients aged 9–14 years. Informed consent was obtained from all patients under a protocol approved by the Ethics Committee, School of Stomatology, Wuhan University. After rinsing the teeth in phosphate buffered saline (PBS), the apical two-thirds of the PDL was scraped off the root surface with a scalpel and teased into small pieces (approximately 1–2 mm3) in tissue culture plates. After a 30-min enzymatic digestion (0.05% trypsin and 0.15% collagenase; Sigma, St. Louis, MO) and centrifugation, single cells in suspension were obtained and then cultured in Dulbecco's modified Eagle's medium (DMEM, GIBCO/Invitrogen, Grand Island, NY) containing 10% fetal bovine serum (FBS, HyClone Laboratories, Logan, UT), 100 U/mL penicillin/streptomycin. The cultures were incubated at 37 °C in an incubator with 5% CO2. The cells were passaged after the monolayer outgrowth reached confluence. The fourth passage of PDL cells was used for this study. Cell cultures were observed under phase-contrast microscopy (Nikon Eclipse TS100) with a CCD camera (Spot Diagnostic Instruments Inc.).
Preparation of PGA scaffold for cell culture
The non-woven PGA fibre mesh was 2 × 2 cm2 with a thickness of 1 mm. The fibre diameter was approximately 12 µm with a specific gravity of 46.31 mg/cm3 and a porosity of 97% (Synthecon Inc., USA). The mesh was cut into 0.5 × 0.5 cm2 pieces and sterilised in 75% alcohol for 30 min. After washing in PBS three times, it was dried in air and stored until use.
Culture of cell-scaffold constructs
PGA scaffolds were put into 6-well culture plates and incubated overnight in DMEM supplemented with 10% FBS, 100 U/mL penicillin/streptomycin at 37 °C.The medium in each block was then removed, and PDL cells were slowly added to each PGA mesh at a density of 2 × 107/mL in 60 µL of medium. After 4 h, 5 mL of culture medium was added to each well. The controls were PGA meshes without cells. Phase-contrast microscopy was used to observe the status of cell adhesion and extension on PGA scaffolds.
Scanning electron microscopy (SEM) examination
After 7 days of culture, the PGA–cell constructs were taken out and fixed in freshly prepared 2.5% (w/v) glutaraldehyde in PBS at pH 7.2 and 4 °C for 24 h. They were then rinsed and dehydrated in gradient concentrations of ethanol and permuted by isoamyl acetate. After critical point drying, the samples were sputter-coated with gold and evaluated by SEM (Hitachi X-650).
Reverse transcription–polymerase chain reaction (RT-PCR)
Total RNA was isolated from cultured primary PDL cells (Group 1), unseeded fourth passage cells (Group 2) and PDL cells within PGA scaffolds (Group 3) using TriReagent (Molecular Research Center, Cincinnati, OH). RNA (3 μg) was used for cDNA synthesis. The RT-PCR reaction was carried out with a DNA thermal cycler (Perkin Elmer Cetus) for 40 cycles (60 s at 94 °C, 60 s at 57 °C, and 60 s at 72 °C). The primers used in this study are listed in . The amplified PCR products were electrophoretically separated in 1% agarose gels and were stained with ethidium bromide. The gene encoding glyceraldehyde 3-phosphate dehydrogenase (GAPDH) was used as a reference.
Table 1. Primer sequences for RT-PCR.
Green fluorescent protein gene transfection and cell-scaffold construct transplantation
PDL cells were cultured at a density of 1 × 106 cells per 100-mm culture dish in DMEM supplemented with 10% FBS. After overnight attachment, the cells were exposed to 4 mL of retroviral expression vector PLNCX2-GFP in the presence of polybrene (8 µg/mL) for 24 h. The medium was then replaced by 10 mL of DMEM supplemented with 10% FBS to remove the potentially toxic polybrene. After 48 h of transfection, cells were exposed for 14 days to G418 (0.6 mg/mL) in DMEM containing 10% FBS. PDL cells that expressed green fluorescent protein (GFP) (0.2 mL of a suspension containing 2 × 105 cells) were then seeded onto the PGA mesh. Implantation study was performed after 7 days of incubation of PGA–cell constructs. Twenty-four six-week-old nude BAL/C-nu mice (Center of Experimental Animals, Hubei, China) served as recipients of implantation. The Animal Care and Experiment Committee of Wuhan University (Hubei, China) approved the experimental protocol. After general anaesthesia, two sagittal incisions were made on the dorsa of BAL/C-nu mice. The PGA meshes containing PDL cells were inserted into the surgical pocket on the right side and the controls were inserted into the left side. The incisions were carefully closed. Mice were housed in the pathogen-free Experimental Animal Center of Wuhan University until sacrificed by cervical dislocation after 2, 4, 6 and 8 weeks. New tissue was harvested and evaluated by snap-freezing in isopentane-ice slush and observed with a fluorescence microscope.
Masson's trichrome staining
After fixing in 10% neutral-buffered formalin, the harvests were embedded in paraffin-wax. Tissue sections of exactly 6 µm in thickness were cut and stained with Masson's trichrome stain. Blood vessels were identified by the inclusion of erythrocytes within the blood vessel lumen under a light microscope. The numbers of blood vessels present in PGA-cells constructs and cell-free scaffolds explanted from the middle portion of the ventral aspect at each time point were counted in a blind manner in six different randomly selected fields within the meshes.
Statistical analysis
All results were expressed as mean values with standard deviation (±SD). The level of statistical significance was assessed by one-way analysis of variance with post-hoc Dunnett's test and paired t-test. Statistical significance was accepted at P < 0.01.
Results and discussion
Cell adhesion and extension on PGA in vitro
As reported in our previous study [Citation14], six to seven days after the beginning of culture, PDL cells covered approximately 60%–70% of the culture dish ((A)). Three days after seeding, PDL cells began to attach on the PGA fibres and extend processes along the full length of the PGA fibres ((B)). On day 7, PDL cells had proliferated to contact with each other and secreted extracellular matrix (ECM), filling the gaps along and between the PGA fibres ((C)).
Figure 1. Cell adhesion and extension on PGA in vitro and mRNA expression of collagen type I, collagen type III and FN. (A–C) Phase-contrast photographs of PDL cells cultured in DMEM supplemented with 10% FBS for 6 days (A) or cultured on PGA polymer fibres in DMEM supplemented with 10% FBS for three days (B) and seven days (C). (D–F) SEM of unseeded PGA scaffolds (D) and PGA fibres seeded with PDL cells for 7 days (E, F). (G) mRNA expression of collagen type I, collagen type III and fibronectin (FN) in primary PDL cells (Group 1), unseeded fourth passage cells (Group 2) and PDL cells within PGA scaffolds (Group 3).
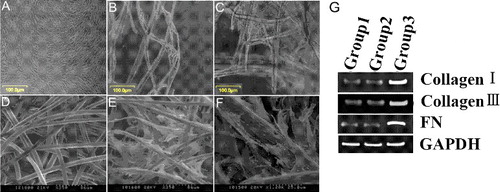
SEM revealed that PGA scaffolds were three-dimensional networks with highly porous fibre bundles ((D)). Human PDL cells were well attached to the surface of the carrier and exhibited a spindle shape or polygonal shape after 7 days of growth. The pseudopodia of cells extended in the same direction and ECM fibres filled in the space between the fibres ((E,F)). After 7 days of incubation, the material became swollen and gelatinous.
PGA scaffolds promote mRNA expression of collagen type I, collagen type III and fibronectin in PDL cells
RT-PCR was used to evaluate the influence of the scaffolds on the expression of collagen type I, collagen type III and fibronectin (FN). As shown in (G), PDL cells within PGA scaffolds (Group 3) had higher mRNA expression levels of collagen type I, collagen type III and FN during the culture periods than did primary PDL cells (Group 1) and unseeded fourth passage cells (Group 2).
It is well known that a cell in a living organism has a three-dimensional architecture. The metabolism and gene expression profile of cells is altered greatly during growth in two-dimensional culture. Some researchers have reported that the utilization of three-dimensional biomaterial scaffolds instead of ‘flat biology’ shows physiological performances that mimic functions seen in human tissues [Citation15,Citation16]. In our study, owing to fundamental disadvantages associated with applying a two-dimensional substrate, we selected a three-dimensionally reconstructed system, which plays a role similar to that of the ECM in natural tissue [Citation7]. The results indicated a high expression of ECM components on PGA fibre networks.
GFP labelling of implanted human PDL cells
When infected with PLNCX2-GFP vector and selected in G418, PDL cells were labelled with GFP, showing that they expressed GFP in culture dishes ((A)). This GFP expression remained observable after seeding onto PGA fibres for 7 days ((B)). Furthermore, 8 weeks after implantation of GFP-labelled PGA-cell scaffolds, GFP expression was still detected by fluorescence microscopy in engineered PDL-like tissue ((C)), indicating that engineered PDL-like tissue was formed by implanted PDL cells.
Figure 2. GFP labelling of implanted human PDL cells and Gross observation of new-born tissues. (A) GFP-labelled cells expressed GFP in culture dishes. (B) After seeding onto PGA fibres for 7 days, GFP expression remained observable. (C) After 8 weeks, GFP expression was detected by fluorescence microscopy in engineered PDL-like tissue. (D) and (E) Gross morphology of the new-born tissue 8 weeks after implantation of the specimen mouse.
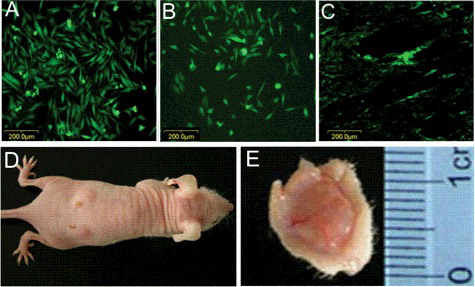
In the mid-1990 s, GFP successfully served as a versatile genetically encoded marker for tracking proteins. Although many other methods have come a long way from initial attempts, the GFP tracking strategy remains relevant because of its convenience, usefulness and effectiveness [Citation17]. The GFP fluorescence showed that the PDL cells in the newly formed tissue were derived only from the PDL cells that adhered to the PGA construct rather than from the autologous cells of the mice.
Gross observation of new-born tissues
(D, E) show that PGA–cell constructs in the experimental group exhibited whitish opacity. No significant difference could be seen macroscopically between the PDL-like tissue and the normal PDL tissue 8 weeks after implantation.
Histological findings in vivo
Histological examination firstly showed the degradation of the PGA mesh, which began to collapse at 2 weeks. Two weeks after implantation, collagen formation was noted in the PGA-cell constructs as indicated by Masson's trichrome staining. The control implants did not contain similar structures.
After 3 weeks, both PGA meshes and PGA–cell constructs were infiltrated by new tissue. Under light microscope, the PGA fibres were cellularised mainly by fibroblasts, with little macrophage-like cells scattered throughout the tissue. Masson's trichrome staining revealed the deposition of collagen in and around the implants ((A)).
Figure 3. Deposition of collagen (A) and formation of new blood vessels (B) in the PGA–cell constructs over a period of 1–4 weeks.
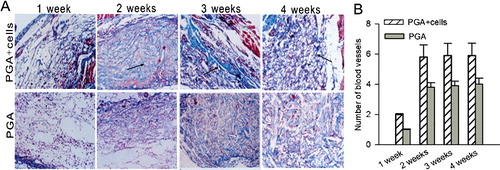
The presence of fibrovascular tissue indicated that the construct had a well-developed blood supply. At each time point, the number of blood vessels counted per field of view in the hybrid group was more than that in the controls. A significant increase in new blood vessels could be seen at 2 weeks (p < 0.01) in the cell–polymer constructs. No significant difference was observed at the other time points ((B)).
A multitude of researches have declared that vascularization is the capstone in tissue engineering application and that cells must be located within 150–200 μm of the nearest capillary to gain access to oxygen and nutrients and, additionally, to remove carbon dioxide and other metabolic products [Citation18,Citation19]. However, obtaining vascularised tissues is an obstacle for cultures engineered in vitro. In this study, we investigated whether engineered tissue could integrate with the host body in terms of vascular supply. To our excitement, we observed abundant new blood vessel ingrowths after 2 weeks in the thin piece of new tissue, which was enough to formulate functional vascular networks. The significant increase in blood vessels in the cell–polymer constructs compared to that in the control suggested that seeding cells might influence the ingrowth of vascular vessels from the surrounding tissues.
Conclusions
The present study suggests that non-woven PGA scaffolds provide effective structural support to promote the secretion of extracellular matrix in PDL cells. The cell-PGA constructs implanted in nude mice formed an engineered PDL-like tissue with a well-developed blood supply. Thus, PGA scaffolds combined with PDL cells offer a strategy for complex periodontal tissue regeneration.
Acknowledgement
This work was financially supported by the National Natural Science Foundation of China under grant number 8140030664.
Disclosure statement
No potential conflict of interest relevant to this work was reported.
Additional information
Funding
References
- de Jong T, Bakker AD, Everts V, et al. The intricate anatomy of the periodontal ligament and its development: Lessons for periodontal regeneration. J Periodont Res. 2017;52:965–974.
- Oshima M, Tsuji T. Whole tooth regeneration as a future dental treatment. Adv Exp Med Biol. 2015;881:255–269.
- Albrektsson T, Chrcanovic B, Ostman PO, et al. Initial and long-term crestal bone responses to modern dental implants. Periodontol 2000. 2017;73:41–50.
- Chen FM, Jin Y. Periodontal tissue engineering and regeneration: current approaches and expanding opportunities. Tissue Eng Part B Rev. 2010;16:219–255.
- Troiano G, Laino L, Zhurakivska K, et al. Adittion of enamel matrix derivatives to bone substitutes for the treatment of intrabony defects: A systematic review,meta-analysis and trial sequential analysis. J Clin Periodontol. 2017;44:729–738.
- Bjørge IM, Kim SY, Mano JF, et al. Extracellular vesicles, exosomes and shedding vesicles in regenerative medicine - a new paradigm for tissue repair. Biomater Sci. 2018;6:60–78.
- Jang YJ, Jung IH, Park JC, et al. Effect of seeding using an avidin-biotin binding system on the attachment of periodontal ligament fibroblasts to nanohydroxyapatite scaffolds: three-dimensional culture. J Periodontal Implant Sci. 2011;41:73–78.
- Yang C, Lee JS, Jung UW, et al. Periodontal regeneration with nano-hyroxyapatite-coated silk scaffolds in dogs. J Periodontal Implant Sci. 2013;43:315–322.
- Liu Y, Zheng Y, Ding G, et al. Periodontal ligament stem cell-mediated treatment for periodontitis in miniature swine. Stem Cells. 2008;26:1065–1073.
- Seo B-M, Miura M, Gronthos S, et al. Investigation of multipotent postnatal stem cells from human periodontal ligament. Lancet. 2004;364:149–155.
- Alinasab B, Haraldsson PO. Rapid resorbable sutures are a favourable alternative to non-resorbable sutures in closing transcolumellar incision in rhinoplasty. Aesth Plast Surg. 2016;40:449–452.
- Iwata T, Yamato M, Tsuchioka H, et al. Periodontal regeneration with multi-layered periodontal ligament-derived cell sheets in a canine model. Biomaterials. 2009;30:2716–2723.
- Wu Y, Wang Y, Ji Y, et al. C4orf7 modulates osteogenesis and adipogenesis of human periodontal ligament cells. Am J Transl Res. 2017;9:5708–5718.
- Wang Y, Xia H, Zhao Y, et al. Three-dimensional culture of human periodontal ligament cells on highly porous polyglycolic acid scaffolds in vitro. Conf Proc IEEE Eng Med Biol Soc. 2005;5:4908–4911.
- McKee C, Chaudhry GR. Advances and challenges in stem cell culture. Colloids Surf B Biointerfaces. 2017;159:62–77.
- Duval K, Grover H, Han LH, et al. Modeling Physiological events in 2D vs.3D cell culture. Physiology (Bethesda). 2017;32:266–277.
- Hoffman RM. Strategies for in vivo imaging using fluorescent proteins. J Cell Biochem. 2017;118:2571–2580.
- Chan EC, Kuo SM, Kong AM, et al. Three dimensional collagen scaffold promotes intrinsic vascularisation for tissue engineering applications. PloS One. 2016 [cited 2017 Jun 10];11:e0149799. DOI: 10.1371/journal.pone.0149799.
- Serbo JV, Gerecht S. Vascular tissue engineering: biodegradable scaffold platforms to promote angiogenesis. Stem Cell Res Ther. 2013 [cited 2018 Jan 29];4:8. DOI: 10.1186/scrt156.