ABSTRACT
Ketogulonicigenium vulgare and Bacillus play an important role in the two-step fermentation of vitamin C. Resolving the interaction between the two strains, not only can reduce the production cost and improve the productivity gains of vitamin C, but also can give significant clues for the study of complex microbial consortia. We analyzed some physiological properties, antioxidant-related enzyme activities and gene expression patterns of Bacillus endophyticus ST-1 and Ketogulonicigenium vulgare 25B-1 in vitamin C mono- and co-culture fermentation systems before and after B. endophyticus ST-1 sporulation. B. endophyticus ST-1 accelerated the growth and caused excessive accumulation of reactive oxygen species in K. vulgare 25B-1, resulting in oxidative stress. Oxidative stress signals could be transmitted to B. endophyticus ST-1, resulting in the upregulation of sodA, katE and gp genes, which are related to oxidation resistance and regulation of the oxidation–reduction state. The release of spores and the lysis of B. endophyticus ST-1 cells provided a favourable oxidation–reduction state for K. vulgare 25B-1 growth. The expression of sodB, grxC, gor and zwf genes in K. vulgare 25B-1 was gradually upregulated, leading to increased antioxidant capacity. B. endophyticus ST-1 regulates the oxidation–reduction state in the vitamin C fermentation system, thereby enhancing the antioxidant capacities of K. vulgare 25B-1.
Introduction
The ‘two-step fermentation’ process is the main method for vitamin C (Vc) industrial production in China, as initially reported by Yin et al. [Citation1]. During the first step, L-sorbose is formed from D-sorbitol by Gluconobacter oxydans, and the second step is completed by co-culture fermentation with two bacteria, a companion strain and an acid-producing strain, for conversion of L-sorbose into 2-keto-L-gulonic acid (2-KLG), the precursor of Vc. The acid-producing strain is Ketogulonicigenium vulgare, and the companion strain is typically from the Bacillus genus, e.g. Bacillus megaterium, B. cereus, B. subtilis or B. pumilus. In a mono-culture, K. vulgare growth is very slow, accompanied by low rate of 2-KLG production. In contrast, the companion strain can greatly accelerate K. vulgare growth and enhance 2-KLG yields in co-culture fermentation [Citation2–4].
Recent studies of interactions between these two strains in the Vc co-culture fermentation system have revealed that the Bacillus spp. companion strain can provide necessary nutrients for the growth of K. vulgare. Additionally, rapid proliferation of K. vulgare occurs after the sporulation of Bacillus spp. [Citation5–8]. A metabolic analysis of K. vulgare identified deficiencies in cysteine synthesis [Citation9]. During Vc fermentation co-culture, cysteine levels were significantly different before and after the sporulation of the companion Bacillus strain [Citation10]. Moreover, the addition of reduced glutathione (GSH) to K. vulgare mono-culture fermentation could significantly enhance cell growth and 2-KLG production [Citation11]. Cysteine, glutathione, mycothiol and coenzyme A are commonly present in bacterial cytoplasm as low-molecular-weight biothiols and redox buffering components, functioning to maintain the balance of cellular oxidation–reduction states [Citation12–14]. Additionally, Ma et al. [Citation15] found that the expression levels of certain proteins, such as superoxide dismutase (SOD), glutathione S-transferase, NAD(P)H:quinone oxidoreductase, and glucose-6-phosphate dehydrogenase, were elevated in co-cultures of K. vulgare with B. megaterium compared to those in K. vulgare mono-cultures. Accordingly, K. vulgare growth and 2-KLG production may be related to the oxidation–reduction state in the surrounding medium.
Herein, we evaluated several physiological properties of mono-cultures of K. vulgare and B. endophyticus ST-1 and those of the co-culture Vc fermentation system composed of K. vulgare 25B-1 and B. endophyticus ST-1. Additionally, we analyzed antioxidant–related enzyme activities and oxidative stress-related gene expression levels in co- and mono-culture fermentation systems before and after B. endophyticus ST-1 sporulation to compare the antioxidant capacities and oxidation–reduction states in different fermentation systems.
Materials and methods
Bacterial strains
The bacterial strains used in this study for Vc fermentation, B. endophyticus ST-1 and K. vulgare 25B-1, were provided by the Northeast Pharmaceutical General Factory of the Vitamin C Company (Shenyang, China). The strains were stored at −80 °C or 4 °C for long- or short-term storage, respectively.
Culture medium and culture conditions
Seed medium used to cultivate K. vulgare 25B-1 and co-culture seeds consisted of 20 g/L sorbose, 1 g/L urea, 2 g/L glucose, 1 g/L CaCO3 and 5 g/L corn steep liquor. Companion strain medium used to cultivate B. endophyticus ST-1 seed consisted of 5 g/L corn steep liquor, 2 g/L glucose and 1 g/L urea. Fermentation medium used for fermentation of different systems consisted of 80 g/L sorbose, 12 g/L urea, 10 g/L corn steep liquor, 1 g/L KH2PO4, 0.2 g/L MgSO4 and 5 g/L CaCO3. The pH values of all culture media were adjusted to 6.7 using NaOH (40% w/v), and media were sterilized at 121 °C for 30 min. For all preparations, sorbose and urea were sterilized separately before mixing with other sterile media components.
Seed preparation and fermentation conditions
For B. endophyticus ST-1 mono-cultures, we selected a single colony from a plate (4 °C for short-term storage) and inoculated it into a 250-mL conical flask filled with 20 mL companion strain medium. The flask was incubated at 30 °C for 12 h with shaking at 200 rpm. Then a single-ring of B. endophyticus ST-1 liquid culture was inoculated into 250-mL conical flasks that contained 20 mL fermentation medium.
For K. vulgare 25B-1 mono-cultures, approximately 200 colonies of K. vulgare 25B-1 on a plate were pooled in 1 mL sterile water. The bacterial suspension was inoculated into 250-mL conical flasks filled with 20 mL seed medium and incubated at 30 °C for 24 h with shaking at 200 rpm to obtain seed cultures. Then, 2 mL seed culture (10% of the total fermentation medium volume) was inoculated into 250-mL conical flasks that contained 20 mL fermentation medium.
For co-cultures of B. endophyticus ST-1 and K. vulgare 25B-1, nearly 200 colonies of K. vulgare 25B-1 on a plate were selected and suspended in 1 mL sterile water (labelled as bacterial liquid culture A). A single-ring from a single colony on a B. endophyticus ST-1 plate was selected and suspended in 1 mL sterile water (labelled as bacterial liquid culture B), and a single-ring of bacterial liquid culture B was selected and combined with bacterial liquid culture A to form a mixed-bacteria suspension (labelled as bacterial liquid culture C). Suspensions were cultured at 30 °C with shaking at 200 rpm for 24 h. Finally, 2 mL bacterial liquid culture C (10% of the total fermentation medium volume) was inoculated into 250-mL conical flasks that contained 20 mL fermentation medium.
Assay methods
Assays for cell density and 2-KLG yields
Cell concentrations of K. vulgare 25B-1 and B. endophyticus ST-1 from different fermentation systems were determined by hemocytometer count. In the fermentation broth, 2-KLG was measured as previously described [Citation1,Citation16].
Determination of oxidation–reduction potential (ORP)
Two millilitres of fermentation broth were added to a 10-mL test-tube, and incubated in a water bath at 30 °C. An OHAUS ST2100/3C Pro pH/ORP meter (Company Infor) was used to monitor ORP, according to the manufacturer's instructions.
Determination of reactive oxygen species (ROS) levels
In mono-cultures of B. endophyticus ST-1 or K. vulgare 25B-1, 3 mL bacterial culture broth was collected at different time points and centrifuged at 13,000 × g for 15 min. Supernatants were discarded, and pellets were washed with phosphate-buffered saline (PBS) three times and then resuspended to a volume of 3 mL (referred to as B.e and K.v).
To separate B. endophyticus ST-1 and K. vulgare 25B-1 from co-cultures, 3 mL bacterial culture broth was collected at different time points and centrifuged at 1500 × g for 5 min, the pellets were of B. endophyticus ST-1. Supernatants were transferred into new centrifuge tubes and centrifuged at 13,000 × g for 15 min, the remaining pellets were of K. vulgare 25B-1. These two pellets were washed with PBS three times and then resuspended to a volume of 3 mL (referred to as Co-K.v and Co-B.e). The differential centrifuge assay can almost separate these two strains. After observation under the microscope, the experimental error can be neglected.
Total intracellular ROS levels in different fermentation systems were determined using a Reactive Oxygen Species Assay Kit with DCFH-DA as probe (chemical fluorescence method; Nanjing Jiancheng Bioengineering Institute, Nanjing, China).
Preparation of intracelluar and extracelluar fluid
Preparation of intracellular fluid
From each of the different fermentation systems, 120 mL fermentation broth was collected and concentrated to 12-mL bacterial suspensions of B.e, K.v, Co-B.e or Co-K.v, using the centrifuge method.
To prepare intracellar fluid of B.e and Co-B.e, lysozyme (final concentration of 20 mg/mL) was added to the two bacterial suspensions, which were placed in a water bath at 37 °C for 60 min. Bacterial culture broths were then placed into 2-mL centrifuge tubes and centrifuged at 13,000 × g for 20 min. Supernatants were filtered with a 0.22-μm filter, and filtrates were considered to be intracellular fluid of B.e and Co-B.e.
To prepare intracellar fluid of K.v and Co-K.v, 4-mL bacterial suspensions were collected and added to 10-mL centrifuge tubes, which were then placed in an ultrasonic disruption instrument surrounded by ice (350 W, operating 5 s with 4-s intervals, 10 min). Samples were centrifuged at 13,000 × g for 20 min, and supernatants were filtered with a 0.22-μm filter. Filtrates were considered to be intracellar fluid of K.v and Co-K.v.
Preparation of extracellular fluid
10 mL extracellular fluid from B.e, K.v, and B.e + K.v were harvested from each fermentation system, then filtered with a 0.22-μm filter and filtrates were considered to be extracelluar fluid of B.e, K.v, and B.e + K.v.
Preparations of intracellular and extracellular fluid were performed as described above, and assessments of total SOD (T-SOD) activity, catalase (CAT) activity, and total antioxidant capacity (T-AOC) were determined using a T-SOD assay kit (hydroxylamine method), a CAT assay kit (visible light) and a T-AOC assay kit (all three kits were from Nanjing Jiancheng Bioengineering Institute, Nanjing, China) following the provider's instructions.
Analysis of differential gene expression
At different time points, 1 mL of bacterial suspension was collected from each of the different fermentation systems and rapidly added to 2 mL RNAProtect Bacterial Reagent (two volumes), followed by oscillation for 2 min. Bacterial suspensions were incubated with oscillation in the presence of lysozyme (final concentration: 20 mg/mL) and proteinase K at 25 °C. Total RNA purification was performed using an RNeasy Mini Kit (Qiagen, Valencia, CA, USA), and cDNA was synthesized using a FastQuant RT Kit (with gDNase, KR106-02; Tiangen, Beijing, China). Quantitative polymerase chain reaction (qPCR) was performed using SYBR Premix Ex Taq II (Tli RNaseH Plus, RR820A; Takara, Dalian, China) according to the manufacturer's instructions, with 16S rDNA as a reference gene [Citation17]. Primers used for qPCR are listed in .
Table 1. qPCR primers used in this study.
Statistical analysis
All experiments were repeated independently three times. Data are expressed as mean values with standard derivations (±SD). Data were analyzed using t-test to assess significance (P< 0.05) using GraphPad Prism 5 software.
Results and discussion
Physiological properties of mono- and co-cultures in Vc fermentation systems
Growth curves for each strain in different fermentation systems
The cell count of B. endophyticus ST-1 and K. vulgare 25B-1 at different time points during the fermentation process are shown in . For K. vulgare 25B-1, the cell density was higher in co-cultures than in mono-cultures at each time point, indicating that the companion strain B. endophyticus ST-1 stimulated the growth of K. vulgare 25B-1 in the Vc co-cultures fermentation system. For B. endophyticus ST-1, the cell growth was accelerated by K. vulgare 25B-1 from 0 to 28 h in co-cultures, and the sporulation of B. endophyticus ST-1 was stimulated after 24 h. Additionally, the growth of B. endophyticus ST-1 was inhibited after 32 h (i.e. after sporulation).
Variations in ORPs and 2-KLG yields in different fermentation systems
The pH value, dissolved oxygen and substances in a fermentation system can greatly alter the microenvironment and have significant effects on the growth and metabolism of micro-organisms [Citation18,Citation19]. In fermentation systems, the oxidation–reduction potential (ORP) can reflect these indices comprehensively. In earlier studies, Knaysi and Dutky [Citation20] found that B. megaterium had notable effects on the ORP of the surroundings during the growth. Indeed, ORP tended to decrease during the growth of B. megaterium [Citation20]. In the present study, we measured the ORPs for different fermentation systems. In mono-cultures of K. vulgare 25B-1, ORP fluctuated slightly around −20 mV, whereas in mono-cultures of B. endophyticus ST-1, ORP changed within a large range of −205– 56 mV. In co-cultures of B. endophyticus ST-1 and K. vulgare 25B-1, ORP fluctuated within the range of −236–61 mV. ORP in the co-culture fluctuated in a pattern more similar to that of B. endophyticus ST-1 mono-culture (a). Thus, it is plausible to suggest that the fluctuations in ORP in the co-cultures system were mainly caused by B. endophyticus ST-1 and the ORP tended to decrease during the fermentation.
Figure 2. Variations in ORP (a) and 2-KLG (b) production in mono- and co-cultures fermentation systems.
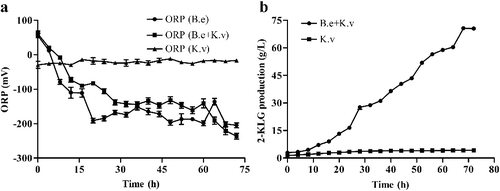
Based on measurements of 2-KLG yields, we found that 2-KLG in co-cultures gradually increased and reached 70 ± 0.4 g/L at 72 h, which was 17-fold higher than in K. vulgare 25B-1 mono-culture (b). Therefore, 2-KLG produced by K. vulgare 25B-1 increased significantly in the presence of the companion strain B. endophyticus ST-1, confirming earlier findings that the Bacillus companion strain promoted the growth and 2-KLG production by the acid producing strain in the co-culture Vc fermentation system [Citation21–24]. Moreover, the analysis of the Pearson correlation coefficient between 2-KLG production and ORP by SPSS 22.0 represented that the correlation of these two variables is significant at the 0.001 level, as the Pearson correlation is −0.875. Accordingly, further studies are needed to determine the specific oxidation-reduction states of each strain in the fermentation process and to elucidate the roles of ORP in 2-KLG production in co-cultures fermentation system.
Responses to oxidative stress in a co-culture fermentation system
Differences in intracellular ROS levels in different fermentation systems
ROS, as natural by-products of aerobic metabolism, are primarily derived from flavins of dehydrogenases and autoxidation of non-respiratory flavoproteins [Citation25–28]. They can play important roles in both cellular signalling and affect the intracellular oxidation–reduction states [Citation29]. Under normal circumstances, cells can eliminate internal ROS through scavenging enzymes, such as SOD (encoded by sod) and CAT (encoded by kat). However, when the amount of ROS is in excess, oxidative stress occurs. We selected four representative time points in the fermentation process: 0 h (beginning of culture), 18 h (before sporulation of B. endophyticus ST-1), 24 h (after a large quantity of spores had been produced by B. endophyticus ST-1) and 40 h (after cell lysis and release of B. endophyticus ST-1 spores). The intracellular ROS levels of B. endophyticus ST-1 and K. vulgare 25B-1 were measured at each selected time point. The ROS levels in K. vulgare 25B-1 did not significantly change at any time point in the mono-culture system. In contrast, in the co-cultures system, the ROS levels for both strains peaked at 18 h, and the difference was significant (P < 0.01); thereafter, the ROS levels gradually decreased (). Previous sequencing results of K. vulgare WSH-001 indicated that there were deficiencies in certain metabolic pathways. In the co-cultures fermentation system, the companion strain can accelerate the growth and 2-KLG production of K. vulgare [Citation5,Citation21], which could result in excessive ROS generation. During the later stages of fermentation (i.e. after sporulation), as ROS gradually accumulated inside K. vulgare cells, the companion strain could help to eliminate ROS. Additionally, in our study, ROS inside B. endophyticus ST-1 cells also increased at 18 h (i.e. before sporulation), potentially due to the oxidative stress caused by the microenvironment of the co-cultures fermentation system.
Differences in T-SOD, CAT activity and T-AOC in different fermentation systems
In agreement with the study described above, we found that intracellular ROS increased in both strains during the co-culture growth phases. Therefore, we subsequently evaluated the intra- and extra-cellular T-SOD, CAT activity and T-AOC in K. vulgare 25B-1 and B. endophyticus ST-1 in different fermentation systems at different time points (). No significant difference in the intra-cellular T-SOD, CAT activity and T-AOC were observed in the K. vulgare 25B-1 mono-culture fermentation system. In contrast, in the B. endophyticus ST-1 mono-culture system, the intra- and extra-cellular T-SOD, CAT activity and T-AOC reached a maximum at 24 h (i.e. after sporulation). In co-cultures, the intracellular T-SOD, CAT activity and T-AOC for B. endophyticus ST-1 reached a maximum at 18 h (i.e. before sporulation), whereas the extracellular T-SOD, CAT activity and T-AOC in the supernatant were significantly increased, reaching a maximum at 40 h (P < 0.01).
Figure 4. Variations in T-SOD (a, b), CAT activity (c, d), and T-AOC (e, f) in different fermentation systems: in K. vulgare 25B-1 (a, c, e) and B. endophyticus ST-1 (b, d, f).
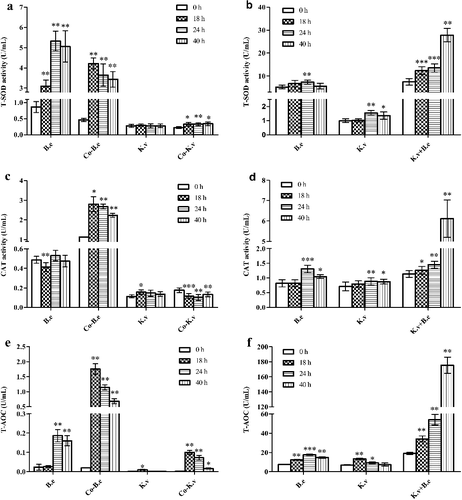
T-AOC can reflect the antioxidant capacities of both enzymes and non-enzyme molecules. These data further suggested that the companion strain B. endophyticus ST-1 could greatly increase the T-AOC of the fermentation system and improve the oxidation–reduction states, which could be explained by the intracellular release of active substances from B. endophyticus ST-1 cells.
Expression levels of oxidative stress-related genes in B. endophyticus ST-1 and K. vulgare 25B-1 in different fermentation systems
Changes in enzyme activities and reduction of oxidative stress in the Vc co-cultures fermentation systems were observed based on the co-operative activities of the two strains, K. vulgare 25B-1 and B. endophyticus ST-1. Therefore, we next addressed whether the reduced oxidative stress was due to the companion strain or K. vulgare 25B-1 by evaluating the expression of oxidative stress-related genes in the two strains from different fermentation systems (). For B. endophyticus ST-1, in both mono- and co-cultures, the expression of the genes sodA, katE, gp and zwf showed similar patterns. The expression levels of these genes (except zwf) in mono-culture were significantly increased during the fermentation and reached maxima at 40 h (P< 0.01); those in co-cultures were also significantly increased over time, reaching maxima at 18 h (P< 0.001). Thus, in the co-culture fermentation system, B. endophyticus ST-1 was exposed to oxidative stress during the early growth period, leading to the upregulation of sodA and katE for direct scavenging of ROS. Glutathione peroxidase (GP) can catalyze the transformation of the reduced form of glutathione (GSH) into its oxidized form glutathione disufide (GSSG), and glutathione reductase (GR) can catalyze the transformation of the oxidized form of glutathione of GSSG into GSH using NADPH as a reducing agent. The main source of reducing capacity in microbial cells is associated with multiple oxidation-reduction enzymes [Citation30], and NADPH is primarily generated by glucose-6-phosphate dehydrogenase (G6PD), encoded by zwf, in the pentose phosphate pathway (PPP) [Citation31]. The upregulation of gp and zwf also indicated improvement of the oxidation–reduction state in mono- and co-cultures fermentation systems. In most Bacillus species, the regulatory factors PerR and OhrR can respond to oxidative stress and control the expression of genes such as katE, ahpCF, mrgA and sodA. Moreover, PerR can also regulate the expression of spx, which responds to thiol stress as a repressor. The spx regulon consists of genes whose products function in thiol homeostasis, cysteine biosynthesis, detoxification and NADPH production, thereby guaranteeing that the cells would maintain a relatively stable oxidation–reduction state [Citation32–34].
Figure 5. Expression levels of the oxidative stress-related genes sodA (a), katE (b), gp (c) and zwf (d) from B. endophyticus ST-1 in mono- and co-cultures.
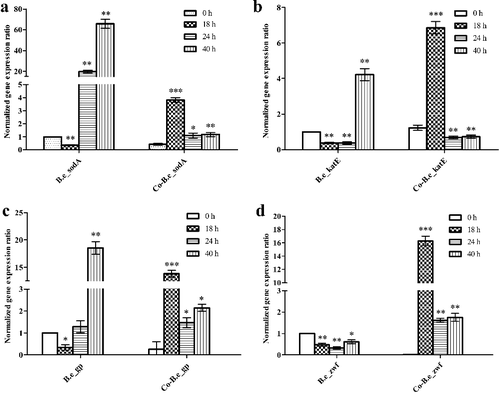
In most bacteria, the modulation of the oxidation–reduction state is closely related to the Grx system, which includes NADPH, GSH, GR and glutaredoxin (Grx) and is responsible for disulfide reduction [Citation35]. Our data showed that for K. vulgare 25B-1 (), in mono-cultures, the expression levels of sodB, katA, gor and grxC gradually decreased. In co-cultures, the expression of sodB, grxC and zwf significantly increased; additionally, the katA and gor expression levels were not significantly altered. These data indicated that the companion strain B. endophyticus ST-1 could enhance the antioxidant capacities and improve the oxidation–reduction state of K. vulgare 25B-1.
Conclusions
In summary, our data suggested that the mechanism through which B. endophyticus ST-1 regulated the oxidation–reduction state of a fermentation system were as follows. First, when K. vulgare 25B-1 is cultured with B. endophyticus ST-1, the growth and 2-KLG production of K. vulgare 25B-1 are enhanced, consistent with changes in metabolic capacities. These changes result in intracellular ROS accumulation, which causes oxidative stress in K. vulgare 25B-1. This signal is then transmitted to B. endophyticus ST-1 via signaling molecules. In response, B. endophyticus ST-1 regulates oxidative stress-related genes and generates active substances to modulate the intracellular oxidation–reduction state. Moreover, K. vulgare 25B-1 can also stimulate the sporulation of B. endophyticus ST-1, resulting in cell lysis and the release of intracellular substances into the fermentation system. This release provides K. vulgare 25B-1 with nutrients, establishes a hospitable oxidation–reduction state and improves the antioxidant capacity of K. vulgare 25B-1. Analysis of the molecular mechanisms of the interactions between K. vulgare and Bacillus strains, not only can establish a theoretical and methodological foundation for further use of traditional mutagenesis techniques, genetic engineering strategies or metabolic engineering methods to enhance vitamin C production, but also can give significant clues for the study of other complex microbial consortia.
Disclosure statement
The authors report no conflicts of interest.
Additional information
Funding
References
- Yin G, He J, Ren S, et al. Production of vitamin C precursor—2-keto-L-gulonic acid from L-sorbose by a novel bacterial component system of SCB329-SCB933 I. The biological characteristics of a novel bacterial component system of SCB329-SCB933. Indust Microbiol. 1997;27(1):1–7.
- Takagi Y, Sugisawa T, Hoshino T. Continuous 2-keto-L-gulonic acid fermentation from L-sorbose by Ketogulonigenium vulgare DSM 4025. Appl Microbiol Biotechnol. 2009;82(6):1049–1056.
- Lyu S, Zhao S, Yang Y, et al. Research progress on Vc precursor of 2-KGA production through mixed fermentation from L-sorbose. Biotechnol Bull. 2011;5(1):50–54.
- Han X, Zhang W, Zhang T. Progress in vitamin C fermentation. Lett Biotechnol. 2009;20:433–435.
- Lyu S, Zhou L, Feng S, et al. The role of Bacillus megaterium in two-step vitamin C fermentation. J Microbiol. 2001;21(3):3–4.
- Zhang J, Liu J, Shi Z, et al. Manipulation of B. megaterium growth for efficient 2-KLG production by K. vulgare. Process Biochem. 2010;45(4):602–606.
- Wang J, Feng W, Wang L, et al. Promotion effect of Bacillus megaterium on Ketogulonigenium vulgare in two-step fermentation of Vitamin C. Chin J Bioprocess Eng. 2009;7:24–28.
- Jiao Y, Zhang W, Xie L, et al. Effect of Bacillus cereus on Gluconobacter Oxydans in vitamin C fermentation process. Microbiol. 2002;29:35–38.
- Huang Z, Zou W, Liu J, et al. Glutathione enhances 2-keto-L-gulonic acid production based on Ketogulonicigenium vulgare model iWZ663. J Biotechnol. 2013;164(4):454–460.
- Zhou J, Ma Q, Yi H, et al. Metabolome profiling reveals metabolic cooperation between Bacillus megaterium and Ketogulonicigenium vulgare during induced swarm motility. Appl Environ Microbiol. 2011;77(19):7023–7030.
- Ma Q, Zhang W, Zhang L, et al. Proteomic analysis of Ketogulonicigenium vulgare under glutathione reveals high demand for thiamin transport and antioxidant protection. PLoS One. 2012 [cited 2017 Aug 19];7:e32156. DOI: 10.1371/journal.pone.0032156.
- Zuber P. Management of oxidative stress in Bacillus. Ann Rev Microbiol. 2009;63:575–597.
- Nakano S, Küster-Schöck E, Grossman AD, et al. Spx-dependent global transcriptional control is induced by thiol-specific oxidative stress in Bacillus subtilis. Proc Natl Acad Sci U S A. 2003;100(23):13603–13608.
- Reyes AM, Pedre B, De Armas MI, et al. Chemistry and redox biology of mycothiol. Antioxid Redox Signal. 2018;28(6):487–504.
- Ma Q, Zhou J, Zhang W, et al. Integrated proteomic and metabolomic analysis of an artificial microbial community for two-step production of vitamin C. PLoS One. 2011 [cited 2017 Aug 19];6:e26108. DOI: 10.1371/journal.pone.0026108.
- Xu A, Yao J, Yu L, et al. Mutation of Gluconobacter oxydans and Bacillus megaterium in a two-step process of L-ascorbic acid manufacture by ion beam. J Appl Microbiol. 2004;96(6):1317–1323.
- Fu S, Zhang W, Guo A, et al. Identification of promoters of two dehydrogenase genes in Ketogulonicigenium vulgare DSM 4025 and their strength comparison in K. vulgare and Escherichia coli. Appl Microbiol Biotechnol. 2007;75(5):1127–1132.
- Riondet C, Cachon R, Waché Y, et al. Extracellular oxidoreduction potential modifies carbon and electron flow in Escherichia coli. J Bacteriol. 2000;182(3):620–626.
- Levar CE, Hoffman CL, Dunshee AJ, et al. Redox potential as a master variable controlling pathways of metal reduction by Geobacter sulfurreducens. ISME J. 2017;11(3):741–752.
- Knaysi G, Dutky SR. The growth of Bacillus megaterium relation to the oxidation-reduction potential and the oxygen content of the medium. J Bacteriol. 1934;27(2):109–119.
- Liu L, Chen K, Zhang J, et al. Gelatin enhances 2-keto-l-gulonic acid production based on Ketogulonigenium vulgare genome annotation. J Biotechnol. 2011;156(3):182–187.
- Lyu S, Wang J, Yu L, et al. Study on the effect of Bacillus megaterium in two-stage fermentation of Vc. J the Grad Sch C A S. 2004;21:84–89.
- Yang W, Liu C, Xu H. l-sorbose is not only a substrate for 2-keto-l-gulonic acid production in the artificial microbial ecosystem of two strains mixed fermentation. J Ind Microbiol Biotechnol. 2015;42(6):897–904.
- Jia N, Ding MZ, Gao F, et al. Comparative genomics analysis of the companion mechanisms of Bacillus thuringiensis Bc601 and Bacillus endophyticus Hbe603 in bacterial consortium. Sci Rep. 2016 [cited 2017 Aug 19];6:28794. DOI:10.1038/srep28794.
- Kussmaul L, Hirst J. The mechanism of superoxide production by NADH: ubiquinone oxidoreductase (complex I) from bovine heart mitochondria. Proc Natl Acad Sci U S A. 2006;103(20):7607–7612.
- Korshunov S, Imlay JA. Two sources of endogenous hydrogen peroxide in Escherichia coli. Mol Microbiol. 2010;75(6):1389–1401.
- Khademian M, Imlay JA. Escherichia coli cytochrome c peroxidase is a respiratory oxidase that enables the use of hydrogen peroxide as a terminal electron acceptor. Proc Nat Acad Sci. 2017;114(33):E6922–E6931.
- Seaver LC, Imlay JA. Are respiratory enzymes the primary sources of intracellular hydrogen peroxide? J Biol Chem. 2004;279(47):48742–48750.
- Devasagayam TPA, Tilak JC, Boloor KK, et al. Free radicals and antioxidants in human health: current status and future prospects. J Assoc Physicians India. 2004;52:794–804.
- Mittler R. Oxidative stress, antioxidants and stress tolerance. Trends Plant Sci. 2002;7:405–410.
- Janero DR. Malondialdehyde and thiobarbituric acid-reactivity as diagnostic indices of lipid peroxidation and peroxidative tissue injury. Free Radic Biol Med. 1990;9(6):515–540.
- Choi SY, Reyes D, Leelakriangsak M, et al. The global regulator Spx functions in the control of organosulfur metabolism in Bacillus subtilis. J Bacteriol. 2006;188(16):5741–5751.
- Helmann JD. Specificity of metal sensing: iron and manganese homeostasis in Bacillus subtilis. J Biol Chem. 2014;289(41):28112–28120.
- Nakano S, Erwin KN, Ralle M, et al. Redox-sensitive transcriptional control by a thiol/disulphide switch in the global regulator, Spx. Mol Microbiol. 2005;55(2):498–510.
- Flohé L. The impact of thiol peroxidases on redox regulation. Free Radical Res. 2016;50(2):126–142.