ABSTRACT
Antimicrobial peptides (AMPs) with their broad and selective antimicrobial activity and lowered risk of resistance development in microbial populations are promining as a new alternative candidate to conventional antibiotics. Plant-based expression systems can be employed as cost-effective and high scale-up capacity production hosts for recombinant expression of AMPs. LFchimera is a chimerical peptide containing LFcin and LFampin antimicrobial peptides of bovine lactoferrin, and it has stronger bactericidal activity. Here, the LFchimera sequence was codon-optimized for tobacco and fused with endoplasmic reticulum retention signals along with a CaMV 35S promoter and then transferred by agrobacterium-mediated transformation. The integration and expression of the transgene in tobacco plants were confirmed by polymerase chain reaction (PCR) and RT-PCR, respectively. Recombinant production of the peptide was confirmed by sodium dodecyl sulphate–polyacrylamide gel electrophoresis (SDS-PAGE), and peptide functional activity was confirmed by the antibacterial evaluation of total protein extracts against various clinical and phytopathogenic bacteria. Finally, LFchimera peptide was partially purified using an affinity column, and the antibacterial activity was shown. Taken together, these results confirmed that tobacco can be utilized for the recombinant production of antimicrobial peptides such as LFchimera.
Introduction
Antimicrobial peptides (AMPs) are considered essential components of the innate immune system, and they typically have broad-spectrum activity against both gram-positive and gram-negative bacteria and fungi; their activity also extends to enveloped viruses, parasites and cancerous cells [Citation1–4]. The continuous increase of resistance among pathogenic microorganisms to conventional antibiotics has encouraged the development of new antimicrobial agents for the treatment of pathogenic diseases [Citation5]. Various AMPs with broad and selective antimicrobial activity and less risk of resistance development in microbial populations are promising, new, alternative candidates for antibiotics [Citation6–11].
To date, several natural AMPs have been identified and isolated from various organisms. However, because of the very low AMP concentrations present in most organisms, the direct isolation of AMPs from natural sources to obtain a reasonable amount of active peptide is a time-consuming and high-cost strategy [Citation12]. The chemical synthesis of peptides allows the production of both natural and synthetic AMPs. Nevertheless, large-scale peptide synthesis is expensive [Citation3]. Moreover, this method is faced with limitations in terms of complex AMPs that show complex post-translational modifications.
Recombinant production in bacteria and yeasts expression systems provide an opportunity for industrial-scale production of AMPs [Citation13]. Nevertheless, there are also some limitations. Factors that influence the expression level of the target peptide include inhibition of host growth by AMPs natural activities, AMP instability against bacterial and yeast proteases, and the inability to perform appropriate post-translational modifications (such as disulphide bonds) and produce recombinant proteins in the form of inactive inclusion bodies [Citation14,Citation15]. Furthermore, due to the requirement for fermentation, the large-scale production of AMPs in bacteria and yeast systems is limited, and the expensive downstream process significantly increases the production costs [Citation16,Citation17].
Heterologous expression of AMPs in various plant-based expression platforms includes plant cell suspensions and hairy root culture; field-grown transgenic plants provide various suitable alternative platforms for the production of AMPs [Citation18], and the development of new approaches in crop protection [Citation19]. These systems have emerged as promising hosts for AMP production because of their advantages over the currently applied expression systems based on bacteria or yeast [Citation20,Citation21]. Plants could successfully perform appropriate glycosylation, folding and disulphide bond formation of recombinant AMPs that are often essential for their biological activity [Citation18]. Plant-based systems offer more advantages towards product yield, quality, homogeneity and safety compared to other systems [Citation22–25].
Constitutive expression of AMPs in transgenic crops provides stable expression of AMPs over many generations and offers easy, fast, scale-up and low-cost production [Citation26,Citation27]. Different leafy crops, e.g. tobacco, lettuce, alfalfa and clover, have been used for the stable expression of proteins in their leaves [Citation23]. Tobacco is one of the best-studied leaf-based platforms for recombinant protein expression. This expression system is highly amenable to genetic manipulation, and there are well-established transformation and regeneration protocols. Tobacco produces high biomass and, unlike other leafy crops, is not a major food/feed crop [Citation28,Citation29].
Lactoferricin and lactoferrampin are two antimicrobial peptides situated positively in the N-terminus of bovine lactoferrin and released from lactoferrin by proteolytic activity. They show broad antibacterial, antifungal, antiviral and antitumor activities [Citation30,Citation31].
The combination of lactoferricin (amino acids 17–30) and lactoferrampin (amino acids 265–284) at their C-termini produces a chimeric peptide, LFchimera, that shows antimicrobial activity in lower required concentrations and shorter incubation times in comparison with the constituent peptides [Citation32–35]. We also previously indicate the antibacterial activity of LFchimera synthetic peptide against important phytopathogenic bacteria [Citation36].
LF-peptides like LFchimera have unique properties such as strong selective antimicrobial activity against clinical and phytopathogenic bacteria, and thus lack toxicity to the cells of higher organisms. These peptides have thermal stability; furthermore, they pose less risk of development of resistance in microbial populations. These characteristics give them the potential of being a promising class of antimicrobial compounds for clinical and agricultural applications [Citation30,Citation31,Citation36–38].
Recently, we successfully expressed and accumulated LFchimera peptide by recombinant production in an in vitro plant culture system and tobacco hairy roots [Citation39]. In this study, our efforts have focused on the use of tobacco whole plants as an expression host for large-scale and cost-effective production of LFchimera and to overcome the limitations of in vitro expression systems. The results showed that LFchimera was successfully expressed in tobacco and leaf extracts and had antibacterial activity against various clinical and phytopathogenic bacteria. Furthermore, the peptide was purified using an affinity column and showed antibacterial activity in a dose-dependent manner.
Materials and methods
Strains and vectors
E. coli strain DH5α was used as the cloning host for vector construction, amplification, and storage. A. tumefaciens strain C58C1 was used for plant transformation, and pBI121 binary vector was used for genetic cloning and plant transformation. Clinical bacteria Escherichia coli ATCC 8739 (gram-negative) and Staphylococcus aureus ATCC 6538 (gram-positive) were obtained from the Iranian Research Organization for Science and Technology (IROST). The phytopathogenic bacteria Ralstonia solanacearum and Erwinia amylovira were obtained from the Bacteriology Laboratory, Department of Plant Protection, Faculty of Agriculture, Shiraz University, Iran. The bacteria were cultured on liquid Luria–Bertani (LB) medium (10 g/L tryptone, 5 g/L yeast extract and 10 g/L NaCl) at a temperature appropriate for the specific bacteria and maintained at 4 °C.
Codon optimization of LFchimera, construction of expression vector and transformation of A. tumefaciens
The oligonucleotide fragment containing the Lfchimera coding region was designed according to Bolscher et al. [Citation33]. Codon usage was optimized for expression in Nicotiana tabacum based on the frequency available from the codon usage database (http://www.kazusa.or.jp/codon/). A BamHI restriction site followed by the Kozak sequence were added to the 5′ end of the codon-optimized sequence. A 6 × His tag and the endoplasmic reticulum (ER) retention signal KDEL followed by the ‘TGA’ termination codon was added at the C-terminus in-frame with the LFchimera CDS ((A)). The entire sequence was artificially synthesized and cloned into expression vector pBI121by Genrey (China) and named pBI-Lfchimera-HIS. In this vector, which contained ampicillin- and kanamycin-resistance genes, the expression of the LFchimera was under the control of the CaMV 35S promoter and the nopaline synthase (NOS) terminator ((B)).
Figure 1. Construction of pBI–LFchimera-HIS expression vector for LFchimera expression in tobacco. (A) oligonucleotide sequence of native and plant optimized LFchimera. The 6 × His tag and the ER-retention signal KDEL were fused at the C-terminus in-frame with the LFchimera CDS. (B) Plasmid pBI121 contained the nptII (kanamycin-resistance) gene under the control of the nopaline synthase (NOS) gene promoter and a codon-optimized LFchimera under the control of the CaMV 35S promoter and with a NOS terminator. RB, T-DNA right border; LB, T-DNA left border.
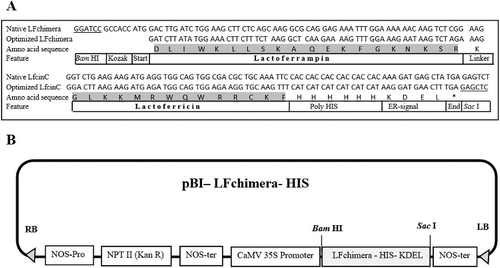
The constructed vector was transformed into DH5α for propagation and then transformed into Agrobacterium tumefaciens strain C58C1 using the electroporation method for use in plant transformation. The nucleotide sequence of the Lfchimera construct was confirmed by restriction profiling and DNA sequencing. An A. tumefaciens culture harbouring the binary vector was grown overnight at 28 °C on a rotary shaker at 170 rpm to mid-log phase (OD600 = 1) in 10 mL liquid LB medium supplemented with 100 mg/L kanamycin and rifampicin. The bacterial cells were collected by centrifugation at 1500 rpm (SIGMA 1–14 microfuge) for 10 min and resuspended to an OD600 of 0.5–1 in MS medium without sugar before plant inoculation.
Transformation of tobacco plants
Transformation of tobacco was done using the leaf disc method. Mature leaves from Nicotiana tabacum cv. Xanthi (one-month-old) were grown aseptically on MS medium [Citation40] containing 30 g/L sucrose (pH 5.8) in 16 h light photoperiod at 24 °C. Then they were cut into 1 cm2, transferred onto a suspension of A. tumefaciens, and incubated for 5 min with gentle shaking. Leaf discs that were co-cultivated with non-transformed A. tumefaciens C58C1 were used as the negative control. The bacteria were poured off, and the explants were dried on sterile filter paper and placed on MS solid medium (containing 0.1 mg/L NAA and 2 mg/L BAP, 30 g/L sucrose, pH 5.8) for 2 days under low light intensity. After two days of inoculation with bacteria, the explants were placed onto selection medium 1 containing 0.1 mg/L NAA, 2 mg/L BAP, 400 mg/L cefotaxime, and 50 mg/L kanamycin, until shoot formation. The regenerated shoots were transferred to selection medium 2 containing 0.1 mg/L NAA, 2 mg/L BAP, 400 mg/L cefotaxime and 100 mg/L kanamycin. Shoots that survived were then placed on a rooting medium containing 2 mg/L IBA, 400 mg/L cefotaxime and 100 mg/L kanamycin. After 10 days, regenerated roots and small seedlings were transferred to sterile soil for growth in the greenhouse.
DNA extraction and PCR reaction to screening putative transgenic plant lines
Integration of the transgene was confirmed by PCR analysis using genomic DNA extracted from 18 putative transgenic plants (T1 to T18) and 1 non-transgenic plant (NT). Genomic DNA was isolated from leaf tissue using the cetyltrimethylammonium bromide (CTAB) method described by Doyle and Doyle [Citation41] and subjected to polymerase chain reaction (PCR) amplification to show the presence of LFchimera with specific primers (forward, 5′-CGCACAATCCCACTATCCTT-3′; reverse, 5′-CACCTTCTCCACTGCCATCT -3′). The primers were designed using Vector NTI 11 Software and were synthesized by MWG Biotech (Germany). All PCR products were then separated by running on a 1% agarose gel and finally visualized using the ethidium bromide staining approach.
Reverse-transcriptase PCR (RT-PCR) analysis
Total RNA was isolated from leaves of transgenic (T3, T8, T11, T14) and non-transgenic (NT) plants (100 mg) using a DENA ZIST reagent kit (Dena Zist, Tehran, Iran) according to the manufacturer's instructions. Then, the quantity and concentration of the RNA were measured using a Nano-Drop ND 1000 Spectrophotometer (Wilmington, USA). The quality and quantity of the extracted RNA were evaluated by visual observation of the 28S and 18S rRNA bands on a 1% agarose gel. Then, confirmed RNAs were treated using DNaseI (Fermentase, Lithonia), and cDNAs were synthesized using a first-strand cDNA synthesis kit (Fermentas, Germany) according to the manufacturer's instructions. The cDNA samples were subjected to PCR amplification of LFchimera and tobacco NtEF1a (GenBank accession number: D63396) with specific primers (forward, 5′-TGCTGCTGTAACAAGATGGATGC-3′; reverse, 5′-GAGATGGGGACAAAGGGGATT -3′) as internal references to detect the quality of the RNA. The RT-PCR mixture was ultimately separated on a 1% agarose gel and photographed.
Total protein preparation
The total proteins were extracted using a phosphate buffer (50 mmol/L, pH 7). Three grams of fresh tobacco leaves were ground under liquid nitrogen, and the powder was suspended in 1:1 phosphate buffer (w/v) and vortexed vigorously for 1 min. Extracts were centrifuged at ∼12,000 rpm for 20 min at 4 °C, and supernatants were filtered using 0.45 μm membranes. The total protein concentration was measured using the method of Bradford [Citation42] with bovine serum albumin (BSA) as the standard and was used for sodium dodecyl sulphate-polyacrylamide gel electrophoresis (SDS-PAGE) analysis and antibacterial activity assays.
SDS-PAGE
About 50 μg of total soluble proteins from transgenic (T3) and non-transgenic (NT) plants was separated using 16.5% tricine–SDS–PAGE, according to the method described by Schagger and Jagow [Citation43]. Following electrophoresis, staining was done using Coomassie brilliant blue.
Antibacterial activities of transgenic tobacco plant extracts
The antimicrobial activity of protein extracts isolated from the leaves of transgenic and non-transgenic (NT) plants was determined by liquid growth inhibition assay in a 96-well microtiter plate. About 10 µg of crude plant extract, 180 µL of LB medium (1% tryptone, 0.5% yeast extract and 0.5% NaCl), and about 20 µL of suspension containing 108 CFU/mL of E. coli, S. aureus, E. amylovira and R. solanacearum was added to every well for each assay. The cells were incubated at a temperature appropriate for the specific bacteria for 18 h. All tests were performed in triplicate, and the mean OD of the bacteria growth inhibition was calculated. Antimicrobial activity was estimated as the percentage of growth inhibition of bacteria.
Peptide purification and antimicrobial activity evaluation
The recombinant peptide was purified using a Ni-NTA column (2.6_10 cm; GE Healthcare Co. Ltd., USA). First, the Ni-NTA column was equilibrated by loading 6 bed volumes of binding buffer (50 mmol/L NaH2PO4, 300 mmol/L NaCl, 10 mmol/L imidazole, pH 8.0); then, 5 mL of a transgenic plant extract containing 6 × His-tagged LFchimera was loaded onto the column. The column was washed with 10 bed volumes of wash buffer (50 mmol/L NaH2PO4, 300 mmol/L NaCl, 20 mmol/L imidazole, pH 8.0), and the protein was eluted using 5 bed volumes of elution buffer (50 mmol/L NaH2PO4, 300 mmol/L NaCl, 0–300 mmol/L imidazole, pH 8.0) with the FPLC System (GE Healthcare) at room temperature. All collected imidazole-eluted fractions were concentrated by freeze-drying and salted out, then eluted with sterile H2O. Finally, purified peptide concentration was measured using Bradford's method and subjected to SDS-PAGE analysis as described above; the antibacterial activity was also evaluated.
The antimicrobial activity of the purified LFchimera peptide was tested against E. coli using the disc-diffusion method [Citation44]. In brief, 25 μL of a bacterial suspension containing 108 CFU/mL was inoculated into 20 mL of pre-warmed (45 °C) nutrient agar (NA); after being vortexed, the mixture was poured into a 90-mm Petri dish and allowed to gel. Finally, 20 μL of purified LFchimera sample (at concentrations of 1 and 1.5 μg/μL) were loaded onto 6-mm sterile paper discs. The loaded discs were dried and placed on the NA plates at 4 °C for 2 h; the plates were then incubated at 37 °C for 18 h. Water was used as a negative control. The antimicrobial activity was evaluated by measuring the diameter of the inhibition zone around the discs (r zone − r disc).
Statistical analysis
Data sets were subjected to analysis by one-way analysis of variance (ANOVA) and Duncan's multiple range test using SPSS software (SPSS 20). In all cases, a value of p < 0.05 was considered significant. The data are expressed as mean values with standard deviations (±SD).
Results and discussion
Selection of transgenic tobacco plants (PCR analysis)
Transgenic tobacco plants carrying a pBI-LFchimera-HIS construct were regenerated on medium containing 100 mg/L kanamycin, and 18 independent kanamycin-resistant transgenic lines were selected for further analysis. Integration of the LFchimera gene into the tobacco genome was confirmed by PCR amplification using primer pairs specific for LFchimera. A 197-bp product was amplified from the positive control and all of the transgenic lines, but not detected in the negative control or the NT plant ((A)).
Figure 2. Screening transgenic plants. (A) PCR amplification of a 197-bp fragment of LFchimera using DNA derived from N. tobacco plants; Lane M, Ruler 1-kb DNA Ladder Mix (Fermentas, Germany); Lanes 1–5, transgenic plant lines; lane P, positive control (A. tumefaciens culture); Lane C, PCR negative control (water); and Lane NT, non-transgenic plant line. (B) RT-PCR analysis of LFchimera in NT and transgenic plants. The NtEF1a gene was used as a control.
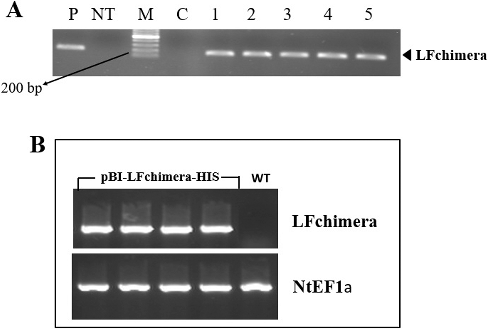
Expression of LFchimera in transgenic tobacco plants (RT-PCR analysis)
After investigating the LFchimera integration in the transgenic plants’ genomes, an RT-PCR was applied to detect transcription of LFchimera mRNA in LFchimera-containing transgenic plants using the NtEF1a gene as an internal reference. A 197-bp expected product was also amplified by RT-PCR from RNA isolated from leaves of PCR-positive plants, but not from NT plants ((B)).
Recombinant production of LFchimera peptide (SDS-PAGE analysis)
The detection of LFchimera peptide in the total soluble protein isolated from a positively integrated and expressed LFchimera tobacco line (T3) was used to monitor the production of recombinant peptide. The presence of peptide in the transgenic lines was clearly distinguished from that of the control tobacco line. As shown in , a prominent peptide band occurred at approximately 6 kDa which matched the predicted Mr for LFchimera.
Figure 3. Expression and partial purification of recombinant LFchimera from plant total soluble protein. Tricine SDS-PAGE (16.5%) showing recombinant peptide and purification profile of LFchimera. Lane M, molecular weight marker (Fermentas); Lane 1, total protein from the NT plant; Lane 2, total protein from the T3 transgenic plant; and Lane 3, purified LFchimera using the affinity column.
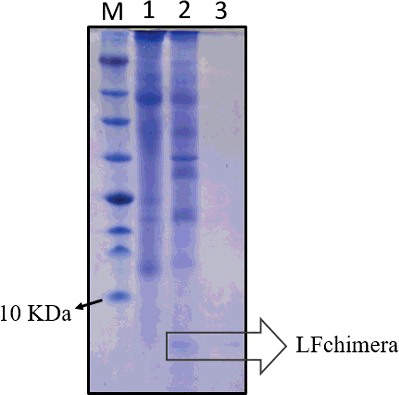
Evaluation of antimicrobial activity
The antimicrobial activity of protein extracts isolated from the leaves of transgenic and non-transgenic (NT) plants was determined against selected clinical and phytopathogen indicator bacteria using liquid growth inhibition assay in a 96-well microtiter plate. Total protein extracts () showed an inhibition range from 18% to 45% on the growth of bacteria (E. coli, S.aureus, E.amylovira and R. solanacearum). Protein extracts from NT tobacco plants did not display any antimicrobial activity. This is the first investigation of antimicrobial activity of recombinant LFchimera against phytopathogenic bacteria. The antimicrobial activity of LF and LFcinB against phytopathogenic bacteria has been investigated in several other studies [Citation45–47].
Peptide purification and antimicrobial activity evaluation
The purified recombinant LFchimera was analyzed by Tricine-SDS–PAGE. The size of the recombinant LFchimera was consistent with the molecular weight of about 6.0 kDa calculated from the deduced amino acid sequence ().
For evaluation, the activity of purified LFchimera peptide and the antibacterial activity of different concentrations of LFchimera were compared to the control (). The results showed a dose-dependent activity of recombinant LFchimera, as indicated for the synthetic peptide active against phytopathogenic bacteria in our previous work [Citation36]. These results suggest that recombinant LFchimera could be employed as a candidate antibiotic peptide or for establishment of transgenic plants with enhanced resistance against bacterial disease.
Figure 5. Antibacterial activity of purified peptide against E. coli, assayed using the disc-diffusion method.
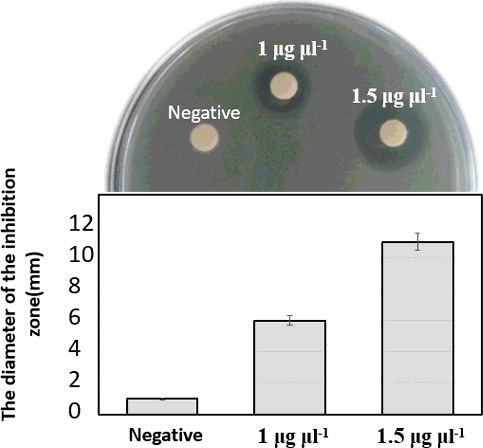
In this study, different strategies were applied to determine the most efficient expression and accumulation of recombinant LFchimera peptide in a plant-based expression system. The choice of a suitable production host and a transformation approach is the first important step in the efficient production of recombinant protein [Citation48]. Transgenic whole plants which could be grown in the field have been used in the inexpensive and scalable production of the majority of plant-derived therapeutic proteins and will be more economical when compared to bacterial expression systems [Citation22,Citation49]. Different plants, including tobacco, potato, tomato, rice, corn, wheat and alfalfa have been used to express a vast variety of recombinant proteins in their leaves, seeds or tubers [Citation23]. Tobacco is the main production host and usually is the most preferred host for the production of pharmaceutical recombinant proteins [Citation29]. Several biopharmaceuticals for human use, such as vaccines, antibodies, cytokines and growth factors, have been successfully produced in field-grown tobacco and are in in vitro, animal or human trials, or are approved for sale [Citation29,Citation50]. Recent studies show that tobacco can be used as a suitable expression system for cost-effective production of diagnostic proteins and interleukins and for stable production of recombinant proteins, such as antibodies, across all the plants generations [Citation49,Citation51,Citation52]. The expression of different recombinant proteins in dicot plants, such as tobacco, is generally achieved through the transformation of the transgene using biological methods and utilizing Agrobacterium bacteria [Citation48]. This method provides simple and stable transformation and integration of the gene of interest in plants’ nuclear genomes [Citation49,Citation53] and is best suited for rapid and scalable transient gene expression in plants [Citation54].
The production of recombinant proteins in each expression system is influenced by several factors at the transformation, transcription, translation, and post-translational steps. Evaluation and optimization of these factors in plant hosts have been improving expression and final yields of recombinant proteins [Citation55]. In this regard, the expression constructs should be optimized to ensure transcript expression, stability and translational efficiency ().
Promoters are important elements of the expression constructs. The strong constitutive promoters are those that are most frequently utilized and give higher expression compared to others, like strong tissue-specific promoters [Citation55]. The constitutive cauliflower mosaic virus 35S (CaMV35S) promoters are most frequently utilized to enhance the expression of recombinant proteins in dicotyledons such as tobacco [Citation48,Citation56,Citation57]. Polyadenylation sites downstream of the stop codon are critical for RNA processing; they also influence the stability of the transcript and the level of protein expression [Citation58]. Widely used polyadenylation sites include those of the CaMV 35S transcript and the Agrobacterium NOS transcript [Citation57,Citation59].
It is indicated that living organisms exhibit different preferred codon usage, such that it might be important to optimize the nucleotide sequence of the transgene without changing the amino acid sequence to suit the respective host. This increases the translational efficiency and subsequently improves the recombinant protein expression and the overall costs of protein production in the considered host [Citation60,Citation61]. Codon optimization is employed to increase the production of human and animal recombinant proteins in the tobacco expression system [Citation49,Citation57]. A similar codon optimization effect was also observed in bacteria and yeast expression systems [Citation62].
In this regard and because of the mammalian origin of LFchimera, genetic codes for 20 out of the 34 amino acids of the LFchimera peptide sequence were codon-optimized for expression in tobacco whole plant, and the translational start-site of the heterologous peptide was modified to match the Kozak consensus for efficient initiation of translation in plants () [Citation61,Citation63]. The results from RT-PCR showed that the LFchimera was properly transcribed, and the transcripts were stable in the plants. The translation of LFchimera transcripts into peptide was confirmed using SDS-PAGE which showed an approximately 6-kDa of protein under native conditions ().
After translation, choosing the correct subcellular targeting can significantly influence the stability and accumulation of recombinant proteins. Recombinant proteins can be targeted to the cytosol, apoplast, chloroplast or vacuole or retained in the ER [Citation48,Citation55]. Among subcellular targets, ER possesses a favourable environment for the stable accumulation of recombinant proteins due to its low protease activity and the presence of molecular chaperones [Citation64,Citation65]. Targeting the protein to the ER can be successfully employed through the addition of the ER retention signal, KDEL (Lys-Asp-Glu-Leu), to the C-terminus of the considered protein, and it can lead to a several-fold increase in accumulation levels compared to targeting to the apoplast or cytosol in tobacco and other plants [Citation66,Citation67]. Therefore, the KDEL tetrapeptide ER-retention signal was fused to the C-terminus of LFchimera ((A)) to facilitate the retention of the peptide within the ER lumen and increase its accumulation in plant cells.
AMPs as antimicrobial agents are frequently used to increase disease resistance in a variety of transgenic plants. The secretion of foreign AMPs to apoplast might enhance the peptide–pathogen interaction [Citation68,Citation69], but the respective peptide might be exposed to plant endogenous proteases and be degraded and/or negatively affect the fitness and fertility of transgenic plants [Citation70]. Conversely, several studies have indicated that targeting to the apoplastic space is not always necessary to obtain disease resistance [Citation71]. Hence, choosing the accurate final destination of AMPs in a plant cell is important and can prevent the cytotoxicity of the AMPs in the host plant. ER's targeting of AMPs can be utilized to promote the accumulation of recombinant AMPs without significantly influencing their biological activity [Citation72]. In another study, the recombinant MAP30 antimicrobial protein was effectively expressed and targeted to ER in Nicotiana tabacum hairy roots; it exhibited significant antibacterial activity [Citation73], and ER-signal was used for successful expression of a plant defencin protein in transgenic tobacco seeds [Citation53]. However, because AMPs have natural destructive effects on microorganisms and are relatively sensitive to proteolytic degradation, they are often produced by fusion to a partner in heterologous hosts and/or by modifying different parameters during fermentation to obtain successful recombinant production [Citation74–76].
The present results indicated that LFchimera was properly expressed and functional peptide accumulated in plant leaves ( and ). Moreover, the produced peptide had no obvious negative impact on the growth or development of transgenic plants (data not shown). Purification is an important step in the efficient production of recombinant protein. PolyHis is the first commercially available affinity tag; it is designed from naturally occurring epitopes and used primarily for purification [Citation77]. Histidine (His) tags are widely used for affinity purification of recombinant proteins in a nickel column [Citation49,Citation78]. Furthermore, the results from the present study confirm that fusion with His tag does not affect the antibacterial activity of recombinant AMPs [Citation79].
Conclusions
The results of this study demonstrated that the transgene was successfully integrated into the tobacco genome and expressed. Functional recombinant peptide was accumulated in leaves without obvious negative impact on the growth or development of transgenic plants. Total protein extracts showed an inhibitory effect on the growth of clinical and phytopathogen indicator bacteria and the purified peptide was effective against bacteria in a dose-dependent manner. The whole plant expression system could be a suitable production system for LFchimera. We envisage that this strategy would be applicable to other AMPs in an efficient manner and that it will minimize the limitations of the production that exist in other expression systems.
Acknowledgments
This work was supported by the Ministry of science, research and technology of Iran under grant number 32/732 in Ilam university. also, the authors would like to thank the Institute of Biotechnology of Shiraz university for supporting some parts of research.
Disclosure statement
The authors declare that they have no conflict of interest.
Additional information
Funding
References
- Silva ON, Mulder KC, Barbosa AE, et al. Exploring the pharmacological potential of promiscuous host-defense peptides: from natural screenings to biotechnological applications. Front Microbiol . 2011; 2:232:1–14.
- Thevissen K, Kristensen HH, Thomma BP, et al. Therapeutic potential of antifungal plant and insect defensins. Drug Discov Today. 2007;12:966–971.
- Li C, Blencke HM, Paulsen V, et al. Powerful workhorses for antimicrobial peptide expression and characterization. Bioeng Bugs. 2010;1:217–220.
- Hoskin DW, Ramamoorthy A. Studies on anticancer activities of antimicrobial peptides. Biochim Biophys Acta. 2008;1778:357–375.
- Verraes C, Van Boxstael S, Van Meervenne E, et al. Antimicrobial resistance in the food chain: a review. Int J Environ Res Public Health. 2013;7:2643–2669.
- Zasloff M. Antimicrobial peptides of multicellular organisms. Nature. 2002;415:389–395.
- Laverty G, Gorman SP, Gilmore BF. The potential of antimicrobial peptides as biocides. Int J Mol Sci. 2011;12:6566–6596.
- Mookherjee N, Hancock RE. Cationic host defense peptides: innate immune regulatory peptides as a novel approach for treating infections. Cell Mol Life Sci. 2007;64:922–933.
- Da Rocha Pitta MG, da Rocha Pitta MG, Galdino SL. Development of novel therapeutic drugs in humans from plant antimicrobial peptides. Curr Protein Pept Sci. 2010;11:236–247.
- Hancock RE. Cationic peptides: effectors in innate immunity and novel antimicrobials. Lancet Infect Dis. 2001;1:156–164.
- Peters BM, Shirtliff ME, Jabra-Rizk MA. Antimicrobial peptides: primeval molecules or future drugs? PLoS Pathog. 2010 [cited 2017 Aug 20];6:e1001067. DOI: 10.1371/journal.ppat.1001067.
- Li C, Haug T, Styrvold OB, et al. Strongylocins, novel antimicrobial peptides from the green sea urchin, Strongylocentrotus droebachiensis. Dev Comp Immunol. 2008;32:1430–1440.
- Parachin NS, Mulder KC, Viana AAB, et al. Expression systems for heterologous production of antimicrobial peptides. Peptides. 2012;38:446–456.
- Li Y. Recombinant production of antimicrobial peptides in Escherichia coli: a review. Protein Expr Purif. 2011;80:260–267.
- Wang XJ, Wang XM, Teng D, et al. Recombinant production of the antimicrobial peptide NZ17074 in Pichia pastoris using SUMO3 as a fusion partner. Lett Appl Microbiol. 2014;59:71–78.
- Calık P, Ata O, Gunes H, et al. Recombinant protein production in Pichia pastoris under glyceraldehyde-3-phosphate dehydrogenase promoter: From carbon source metabolism to bioreactor operation parameters. Biochem Eng J. 2015;95:20–36.
- Rosano GL, Ceccarelli EA. Recombinant protein expression in Escherichia coli: advances and challenges. Front Microbiol. 2014 [cited 2017 Aug 20];5:172. DOI: 10.3389/fmicb.2014.00172.
- Holaskova E, Galuszka P, Frebort I, et al. Antimicrobial peptide production and plant-based expression systems for medical and agricultural biotechnology. Biotechnol Adv. 2015;33:1005–1023.
- Montesinos E. Antimicrobial peptides and plant disease control. FEMS Microbiol Lett. 2007;270:1–11.
- Huang J, Wu L, Yalda D, et al. Expression of functional recombinant human lysozyme in transgenic rice cell culture. Transgenic Res. 2002;11:229–239.
- Basaran P, Rodriguez-Cerezo E. Plant molecular farming: opportunities and challenges. Crit Rev Biotechnol. 2008;28:153–172.
- Xu J, Dolan MC, Medrano G, et al. Green factory: Plants as bioproduction platforms for recombinant proteins. Biotechnol Adv. 2012;30:1171–1184.
- Fischer R, Stoger E, Schillberg S, et al. Plant-based production of biopharmaceuticals. Curr Opin Plant Biol. 2004;7:152–158.
- Peterson RKD, Charles AJ. On risk and plant-based biopharmaceuticals. Trends Biotechnol. 2004;22:64–66.
- Magnusdottir A, Vidarsson H, Björnsson JM, et al. Barley grains for the production of endotoxin-free growth factors. Trends Biotechnol. 2013;31:572–580.
- Cabanos C, Ekyo A, Amari Y, et al. High-level production of lactostatin, a hypocholesterolemic peptide, in transgenic rice using soybean A1aB1b as carrier. Transgenic Res. 2013;22:621–629.
- Morassutti C, De Amicis F, Skerlavaj B, et al. Production of a recombinant antimicrobial peptide in transgenic plants using a modified VMA intein expression system. FEBS Lett. 2002;519:141–146.
- Davies HM. Commercialization of whole-plant systems for biomanufacturing of protein products: evolution and prospects. Plant Biotechnol J. 2010;8:845–861.
- Tremblay R, Wang D, Jevnikar AM, et al. Tobacco, a highly efficient green bioreactor for production of therapeutic proteins. Biotechnol Adv. 2010;28:214–221.
- García-Montoya IA, Cendón TS, Arévalo-Gallegos S, et al. Lactoferrin a multiple bioactive protein: an overview. Biochim Biophys Acta. 2012;1820:226–236.
- Gifford JL, Hunter HN, Vogel HJ. Lactoferricin: a lactoferrin-derived peptide with antimicrobial, antiviral, antitumor and immunological properties. Cell Mol Life Sci. 2005;62:2588–2598.
- Bolscher JG, Adao R, Nazmi K, et al. Bactericidal activity of LFchimera is stronger and less sensitive to ionic strength than its constituent lactoferricin and lactoferrampin peptides. Biochimie. 2009;91:123–132.
- Leon-Sicairos N, Canizalez-Roman A, De La Garza M, et al. Bactericidal effect of lactoferrin and lactoferrin chimera against halophilic Vibrio parahaemolyticus. Biochimie. 2009;91:133–140.
- Bolscher J, Nazmi K, Van Marle J, et al. Chimerization of lactoferricin and lactoferrampin peptides strongly potentiates the killing activity against Candida albicans. Biochem Cell Biol. 2012;90:378–388.
- Leo´n-Sicairos N, Angulo-Zamudio UA, Vidal JE, et al. Bactericidal effect of bovine lactoferrin and synthetic peptide lactoferrin chimera in Streptococcus pneumonia and the decrease in luxS gene expression by lactoferrin. Biometals. 2014;27:969–980.
- Chahardoli M, Fazeli A, Ghbooli M. Antimicrobial activity of LFchimera synthetic peptide against plant pathogenic bacteria. Arch Phytopathology Plant Protect. 2017;50(19–20):1008–1018.
- Haney EF, Nazmi K, Bolscher J, et al. Structural and biophysical characterization of an antimicrobial peptide chimera comprised of lactoferricin and lactoferrampin. Biochim Biophys Acta. 2012;1818:762–775.
- Tang XH, Tang ZR, Wang SP, et al. Expression, purification, and antibacterial activity of bovine lactoferrampin–lactoferricin in Pichia pastoris. Appl Biochem Biotechnol. 2012;166:640–651.
- Chahardoli M, Fazeli A, Ghbooli M. Recombinant production of bovine Lactoferrin-derived antimicrobial peptide in tobacco hairy roots expression system. Plant Physiol Biochem. 2018;123:414–421.
- Murashige T, Skoog F. A revised medium for rapid growth and bioassay with tobacco tissue cultures. Physiol Plant. 1962;15:473–497.
- Doyle JJ, Doyle JL. A rapid DNA isolation procedure for small quantities of fresh leaf tissues. Phytochem Bull. 1987;19:11–150.
- Bradford MM. A rapid and sensitive method for quantitation of microgram quantities of protein utilising the principle of protein-dye binding. Anal Biochem. 1976;72:248–254.
- Schagger H, Jagow GV. Tricine-sodium dodecyl sulfate-polyacryl-amide gel electrophoresis for the separation of proteins in the range from 1 to 100 kDa. Anal Biochem. 1987;166:368–379.
- Vincent J, Vincent H. Filter paper disc modification of the Oxford cup penicillin determination. Proc Soc Exp Biolo Med. 1944;55:162–164.
- Mitra A, Zhang Z. Expression of a human lactoferrin cDNA in tobacco cells produces antibacterial protein. Plant Physiol. 1994;106:977–981.
- Zhang Z, Coyne D, Vidaver AK, et al. Expression of human lactoferrin cDNA confers resistance to Ralstonia solanacearum in transgenic tobacco plants. Phytopathology. 1998;88:730–734.
- Fukuta S, Kawamoto KI, Mizukami Y, et al. Transgenic tobacco plants expressing antimicrobial peptide bovine lactoferricin show enhanced resistance to phytopathogens. Plant Biotechnol. 2012;29:383–389.
- Egelkrout E, Rajan V, Howard JA. Overproduction of recombinant proteins in plants. Plant Sci. 2012;184:83–101.
- Sadeghi A, Mahdieh M, Salimi S. Production of Recombinant Human Interleukin-11 (IL-11) in transgenic tobacco (Nicotiana tabacum) plants. J Plant Biotechnol. 2016;43:432–437.
- Tschofen M, Knopp D, Hood E, et al. Plant molecular farming: much more than medicines. Annu Rev Anal Chem. 2016;9:271–294.
- Ganapathy M, Chakravarthi M, Jason Charles S, et al. Immunodiagnostic properties of Wucheraria bancrofti SXP-1, a potential filarial diagnostic candidate expressed in tobacco plant, Nicotiana tabacum. Appl Biochem Biotechnol. 2015;176:1889–1903.
- Ma J, Drossard J, Lewis D, et al. Regulatory approval and a first-in-human phase I clinical trial of a monoclonal antibody produced in transgenic tobacco plants. Plant Biotechnol J. 2015;13:1106–1120.
- Thao HT, Lan NTL, Tuong HM, et al. Expression analysis of recombinant Vigna radiata plant defensin 1 protein in transgenic tobacco plants. J App Biol Biotech. 2017;5:70–75.
- Edgue G, Twyman RM, Beiss V. Antibodies from plants for bionanomaterials. WIREs Nanomed Nanobiotechnol. 2017 [cited 2017 Aug 20];9:e1462. DOI: 10.1002/wnan.1462.
- Desai PN, Shrivastava N, Padh H. Production of heterologous proteins in plants: Strategies for optimal expression. Biotechnol Adv. 2010;28:427–435.
- Odell JT, Nagy F, Chua NH. Identification of DNA sequences required for activity of the cauliflower mosaic virus 35S promoter. Nature. 1985;313:810–812.
- Shahriari AG, Bagheri A, Bassami MR, et al. Expression of hemagglutinin–neuraminidase and fusion epitopes of newcastle disease virus in transgenic tobacco. Electron J Biotechnol. 2016;22:38–43.
- Lin HH, Huang LF, Su HC, et al. Effects of the multiple polyadenylation signal AAUAAA on mRNA 3′-end formation and gene expression. Planta. 2009;230(4):699–712.
- Ma JKC, Drake PMW, Christou P. The production of recombinant pharmaceutical proteins in plants. Nat Rev Genet. 2003;4:794–805.
- Gustafssion C, Govindarajan S, Minshull J. Codon bias and heterologous protein expression. Trends Biotechnol. 2004;22(7):346–353.
- Kang TJ, Han SC, Jang MO, et al. Enhanced expression of B-subunit of escherichia coli heat-labile enterotoxin in tobacco by optimization of coding sequence. Appl Biochem Biotechnol. 2004;117:175–187.
- Deng T, Ge H, He H, et al. The heterologous expression strategies of antimicrobial peptides in microbial systems. Protein Expr Purifi. 2017;140:52–59.
- Kawaguchi R, Bailey-Serres J. Regulation of translational initiation in plants. Curr Opin Plant Biol. 2002;5(5):460–465.
- Nuttall J, Vine N, Hadlington JL, et al. ER-resident chaperone interactions with recombinant antibodies in transgenic plants. Eur J Biochem. 2002;269(24):6042–6051.
- Conrad U, Fiedler U. Compartment-specific accumulation of recombinant immunoglobulins in plant cells: an essential tool for antibody production and immunomodulation of physiological function and pathogen activity. Plant Mol Biol. 1998;38(1–2):101–109.
- Wirth S, Calamante G, Mentaberry A, et al. Expression of active human epidermal growth factor (hEGF) in tobacco plants by integrative and non-integrative systems. Mol Breed. 2004;13(1):23–35.
- Fiedler U, Philips J, Artsaenko O, et al. Optimisation of scFv antibody production in transgenic plants. Immunotechnology. 1997;3:205–216.
- Düring K. Genetic engineering for resistance to bacteria in transgenic plants by introduction of foreign genes. Mol Breed. 1996;2:297–305.
- Jan PS, Huang HY, Chen HM. Expression of a synthesized gene encoding cationic peptide cecropin B in transgenic tomato plants protects against bacterial diseases. Appl Environ Microbiol. 2010;76:769–775.
- Coca M, Peñas G, Gómez J, et al. Enhanced resistance to the rice blast fungus Magnaporthe grisea conferred by expression of a cecropin A gene in transgenic rice. Planta. 2006;223:392–406.
- Zhou M, Hu Q, Li Z, et al. Expression of a novel antimicrobial peptide Penaeidin4-1 in creeping bentgrass (Agrostis stolonifera L.) enhances plant fungal disease resistance. PLoS One. 2011 [cited 2017 Aug 20];6:e24677. DOI: 10.1371/journal.pone.0024677.
- Badosa E, Moiset G, Montesinos L, et al. Derivatives of the antimicrobial peptide BP100 for expression in plant systems. PLoS One. 2013 [cited 2017 Aug 20];8:e85515. DOI: 10.1371/journal.pone.0085515.
- Moghadam A, Niazi A, Afsharifar A, et al. Expression of a recombinant anti-HIV and anti-tumor protein, MAP30, in nicotiana tobacum hairy roots: A pH-stable and thermophilic antimicrobial protein. PLoS ONE. 2016 [cited 2017 Aug 20];11(7):e0159653. DOI: 10.1371/journal.pone.0159653.
- Panteleev PV, Ovchinnikova TV. Improved strategy for recombinant production and purification of antimicrobial peptide tachyplesin I and its analogs with high cell selectivity. Biotechnol Appl Biochem. 2017;64:35–42.
- Elhag O, Zhou D, Song Q, et al. Screening, expression, purification and functional characterization of novel antimicrobial peptide genes from Hermetia illucens (L.). PLoS ONE. 2017 [cited 2017 Aug 20];12(1):e0169582. DOI: 10.1371/journal.pone.0169582.
- Sang M, Wei H, Zhang J, et al. Expression and characterization of the antimicrobial peptide ABP-dHC-cecropin A in the methylotrophic yeast Pichia pastoris. Protein Expr Purifi. 2017;140:44–51.
- Young CL, Britton ZT, Robinson AS. Recombinant protein expression and purification: a comprehensive review of affinity tags and microbial applications. Biotechnol J. 2012;7:620–634.
- Abdoli Nasab M, Jalali Javaran M, Cusido RM, et al. Purification of recombinant tissue plasminogen activator (rtPA) protein from transplastomic tobacco plants. Plant Physiol Bioch. 2016;108:139–144.
- Chen GH, Chen WM, Huang GT. Expression of recombinant antibacterial lactoferricin-related peptides from Pichia pastoris expression system. J Agric Food Chem. 2009;57:9509–9515.