Abstract
In this study, we explored the effect of NO-induced changes in [Ca2+]i homeostasis in rat ventricular cardiomyocytes through variation in extracellular Ca2+ and application of SNAP (S-nitroso-N-acetyl-d,l-penicillamine) as an NO-donor. The Fura-2 signal dynamics and phalloidin intensity profile of z-disks in rat cardiomyocytes served as endpoints to describe the mechanisms involved in the control of the intracellular Ca2+ levels, depending on the kinetics of distribution of the SNAP-produced NO. The results demonstrated that SNAP caused small phalloidin intensity profile changes between the z-lines in the presence of [Ca2+]o. However, in the absence of [Ca2+]o, SNAP in a concentration of 300 μmol/L induced a significant decrease in the distance between z-lines. This SNAP-induced decrease was reflected as a decrease in the phalloidin fluorescence intensity in the middle of the sarcomere, due to the preferential imaging of greater fluorescence in the bulk of the thin filaments. Based on the Ca2+ fluorescence intensity profile, we could suggest that intracellular Ca2+ is mainly affected by the mechanisms of Ca2+ outflow, rather than the mechanisms of Ca2+ inflow in the presence of NO. Actually, through variation in the electrochemical gradient of Ca2+, we induced mechanisms of faster/slower cytosolic Ca2+ emptying. The obtained data showed that the delay in the cytosolic Ca2+ emptying in the presence of [Ca2+]o is due to the increased electrochemical gradient of Ca2+.
Introduction
L-type Ca2+ channels (LTCCs) are the major mediator of Ca2+ influx in cardiomyocytes, regulating both excitation–contraction coupling and the activation of several signaling cascades. The Ca2+ current (ICa) is itself regulated by several pathways, including β-adrenergic, Ca2+/calmodulin-dependent protein kinase and calcineurin [Citation1]. Calcineurin is linked to the z-disk via calsarcin but also directly binds to and dephosphorylates the LTCCs [Citation2, Citation3]. Published data suggest that described patterns are clearly dependent on intracellular Ca2+ ([Ca2+]i) homeostasis, and their proper functioning is dependent on [Ca2+]i regulatory mechanisms as well [Citation4].
Nitric oxide (NO) markedly influences the [Ca2+]i homeostasis [Citation5, Citation6] by affecting the influx of Ca2+ through the plasma membrane and its release from the intracellular stores. Many reports have discussed the inhibitory effects of NO on the heart’s ICa. Sodium nitroprusside (SNP, 100 μmol/L) inhibits ICa in neonatal rat cardiac ventricular myocytes [Citation7]. NO-synthase inhibitors, L-NG-monomethyl arginine citrate (L-NMMA, 1 mmol/L) and L-NG-Nitroarginine (L-NNA, 1 mmol/L) induce rapidly pronounced stimulation of ICa in isolated guinea-pig ventricular myocytes [Citation8, Citation9]. Other authors have shown that rat ventricular myocytes [Citation10] and rabbit sinoatrial cells pre-stimulated with β-adrenergic agonist [Citation11–13] show inhibited ICa in the presence of different NO-donors. There are also reports of biphasic effects of NO on ICa in cardiomyocytes [Citation14]. Kirstein et al. [Citation15] showed that the NO-donor 3-morpholino-sydnonymine (SIN-1) has a pronounced stimulatory effect on ICa in isolated human atrial myocytes, starting from 1 pmol/L concentration. Moreover, it attains a maximal stimulatory effect (doubling of current amplitude) at 1 nmol/L, while increasing its concentration to 100 mmol/L abruptly reduces its stimulatory effect on ICa.
Several authors report dual effects of NO on ICa. Campbell et al. [Citation16] showed that in isolated ferret ventricular myocytes, SIN-1 (1 mmol/L) has a stimulatory effect on ICa in ∼40% of cells, an inhibitory effect in 40% of cells, and a biphasic effect in 20% of cells. Furthermore, Whaler and Dollinger (1995) used SIN-1 (10 μmol/L) in isolated guinea-pig ventricular myocytes to create a inhibitory effect, while in myocytes pre-stimulated with β-adrenergic agonist, the SIN-1 created a stimulatory effect on LTCCs [Citation17].
There are conflicting data about the influence of NO on [Ca2+]i homeostasis. The few studies performed with NO donors indicated different dose-dependent effects. In order to determine the NO-induced changes in intracellular Ca2+ homeostasis in rat ventricular cardiomyocytes, we employed a novel approach using fluorescent dyes. Through variation in extracellular Ca2+ ([Ca2+]o), and application of the NO-donor, S-nitroso-N-acetyl-d,l-penicillamine (SNAP), based on the Fura-2 signal dynamics and phalloidin intensity profile between z-disks in rat ventricular cardiomyocytes, we explored the mechanisms involved in the control of [Ca2+]i, depending on the kinetics of distribution of the SNAP-produced NO.
Materials and methods
Animals
All animal experiments were carried out in accordance with the Guide for Care and Use of Laboratory Animals published by the US National Institute of Health (8th edition, 2011). The experimental protocol was approved by the Bioethics Committee of the Moscow State University. Male outbred white rats weighing 200–250 g (n = 28) were held in the animal house for 4 weeks under 12:12-h light:dark period in standard T4 cages prior to the experiment. The animals were fed ad libitum.
Isolated cardiomyocyte preparation
The previously described cell isolation procedure was used [Citation17] (with slight modifications). Also, the rat hearts were isolated as described previously [Citation17]. The hearts were attached to the Langendorff apparatus for retrograde perfusion with a Ca2+-free solution containing: 120 mmol/L NaCl, 5.4 mmol/L KCl, 5 mmol/L MgSO4·7H2O, 5 mmol/L Na-pyruvate, 20 mmol/L glucose, 20 mmol/L taurine and 10 mmol/L Hepes at pH 7.4, adjusted with NaOH [Citation18–21]. After an initial perfusion period of 5 min with a Ca2+-free solution, the hearts were perfused for 18–20 min with the same solution and supplemented with type II collagenase (0.5 mg/mL), type XIV protease (0.08 mg/mL) and 20 μmol/L CaCl2. The perfusate was continuously bubbled with carbogen, and the temperature was equilibrated at 37 °C. Finally, the ventricles were separated, chopped and gently triturated to release the cells into a standard Kraftbrühe medium [Citation17]. The suspension of the cells obtained from each of the hearts was stored in a normal Kraftbrühe medium.
Phalloidin staining and confocal microscopy
The cells were fixed in 4% PFA (paraformaldehyde, 4%) for 25 min at room temperature. After washing (1 × 10 min with 120 mmol/L NaH3PO4, 1 × 10 min with LS-Buffer (phosphate buffer containing 150 mmol/L NaCl), 2 × 10 min with HS-Buffer (phosphate buffer containing 500 mmol/L NaCl), the cells were incubated with Alexa Fluor 488 phalloidin, 5 U/mL in fetal bovine serum (FBS; 16.7% fetal calf serum, 450 mmol/L NaCl and 20 mmol/L phosphate buffer) for 1.5 h at room temperature. After washing (1 × 10 min with HS-Buffer, 1 × 10 min with 120 mmol/L NaH3PO4, 1 × 10 min with 5 mmol/L NaH3PO4), the cells were incubated with nuclear stain. Nuclear staining was done with DAPI (4′,6-diamidino-2-phenylindole, Molecular Probes, Eugene, Oregon, USA), 10 μg/mL.
Images were collected using an inverted confocal laser scanning microscope, LSM-700 (Zeiss, Germany). The fluorescence of Alexa Fluor Phalloidin was excited at 488 nm and monitored using an LP505 emission filter; DAPI fluorescence was excited at 405 nm and registered through a 420–480 emission filter.
The images were analyzed using the Fiji/ImageJ software. The laser power and the detector settings were kept constant to maintain consistency in the data collection system.
Calcium imaging
The cardiomyocytes were loaded with the acetoxy-methyl ester form of Fura-2 (Fura-2 AM; 1 μmol/L) for 20–30 min at room temperature. After loading, the cells were incubated in a dye-free solution for 30 min to allow conversion of the colour to its Ca2+-sensitive form. The cells were plated on glass-bottom dishes and mounted on the stage of an inverted Olympus IX81 microscope with a 20× objective. Only well-attached cells (as assessed by whether a brief test pulse of shear fluid would move or blow the cell away) were used. Fura-2 fluorescence was excited at 340 nm and focused on the cells via a 20× objective (LUC Plan FL N 20x/0.45 Ph1, Olympus, Tokyo, Japan). The 510 nm emitted fluorescence was collected by a high-speed cooled CCD camera (Olympus, Tokyo, Japan) and recorded with cellM&cellR software. A quantitative analysis of fluorescent images was performed using Fiji/ImageJ software.
Chemicals
NaCl, KCl, MgSO4·7H2O, NaH3PO4, NaOH, CaCl2, Na-pyruvate, glucose, taurine, Hepes and SNAP were purchased from Sigma-Aldrich (St. Louis, MO). Collagenase type II and protease type XIV were purchased from Worthington, Lakewood, NJ. Fluorescent dyes, Fura-2 AM, the Alexa Fluor 488 Palloidine and DAPI were purchased from Molecular Probes (Eugene, OR). PFA, LS-Buffer, HS-Buffer and FSBB were purchased from Sigma-Aldrich (St. Louis, MO). Carbogen (95% O2 and 5% CO2) was obtained from Technical Gases-Moscow (Russian Federation).
Statistical analysis
One-way analysis of variance (ANOVA), followed by the Newman–Keuls multiple comparison tests, was used to analyze the tested groups. The statistical significance was used for testing two-tailed probabilities. The statistical significance was set at p < .05. All the analyses were performed with Graph Pad Prism 4.0 (San Diego, CA).
Results and discussion
This study highlights the modulatory effects of NO on [Ca2+]i homeostasis and discusses functional implications from the interaction of NO with [Ca2+]i. We have established a new approach based on changes in the phalloidin intensity profile between a cardiomyocyte’s z-disks depending on the changes in [Ca2+]i. The relative intensities at the z-band in the sarcomeres taken from the cardiomyocytes in the presence of [Ca2+]o (1.8 mmol/L) or SNAP (300 μmol/L) are shown in and . SNAP caused insignificant (p > .05) phalloidin intensity profile changes between z-lines in the presence of 1.8 mmol/L [Ca2+]o (). However, in the absence of [Ca2+]o, SNAP in a concentration of 300 μmol/L, induced a significant reduction in the distance between the z-lines (p < .05) (). We believe that such changes are a reflection of NO-induced changes in intracellular Ca2+. It was demonstrated that exogenous NO triggers classic ischemic preconditioning by preventing intracellular Ca2+ overload in cardiomyocytes [Citation22]. In fact, we believe that NO released by SNAP inhibits LTTCs and stimulates mechanisms of extracellular Ca2+ release. It appears that SNAP-induced Ca2+ release is reflected as a decrease in phalloidin fluorescence intensity in the middle of the sarcomere, due to preferential imaging of greater fluorescence in the bulk of thin filaments (). However, in the presence of high [Ca2+]o (1.8 mmol/L), the effect of NO was not pronounced, probably due to the high concentration gradient of Ca2+. This high [Ca2+]o is the reason for replenished intracellular Ca2+ stores [Citation23], and stable intracellular Ca2+ homeostasis (). More evidence that the entire process is Ca2+-dependent comes from the comparison between the date in . As can be seen, 300 μmol/L SNAP did not show the same effect in the presence or absence of [Ca2+]o. This indicates some involvement of NO in the regulation of intracellular Ca2+ homeostasis through Ca2+ regulatory players [Citation24].
Figure 1. Effect of SNAP (300 μmol/L), on zz-distance in the absence (A) or presence (B) of 1.8 mmol/L [Ca2+]o; n = 19; *p < .05.
![Figure 1. Effect of SNAP (300 μmol/L), on zz-distance in the absence (A) or presence (B) of 1.8 mmol/L [Ca2+]o; n = 19; *p < .05.](/cms/asset/f823c519-d9ef-4111-a871-1139819a8db4/tbeq_a_1488621_f0001_b.jpg)
Figure 2. Relative fluorescence intensity distribution (A) across the two typical sarcomeres shown in B and C, (n = 18). Fluorescence patterns of phalloidin incorporation into actin filaments of a representative rat ventricular cardiomyocyte in the absence (B) or presence (C) of 300 μmol/L SNAP? *p < .05.
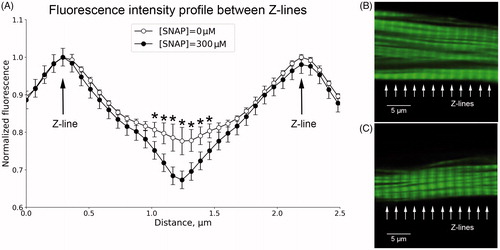
The Fura-2 fluorescence intensity of stained cardiomyocytes is presented in . The fluorescence intensity was recorded from the manually outlined region of interest along the cell membrane. The horizontal character of the curve demonstrates a stable level of [Ca2+]i during the experiment. In order to evaluate the effect of SNAP on [Ca2+]i assayed by means of Fura-2 fluorescence, the cardiomyocytes were incubated with SNAP (300 μmol/L) in the presence of 1.8 mmol/L [Ca2+]o (). In the absence of SNAP, [Ca2+]o did not cause any change in the Fura-2 dynamics. In contrast, in the absence of [Ca2+]o, the effect of SNAP on the normalized fluorescence drop was more pronounced in comparison to its effect in the presence of [Ca2+]o (, empty vs. black circles). Interestingly, [Ca2+]o in the presence of SNAP induced [Ca2+]i overload between 10 and 25 min of incubation, as expressed through increased fluorescence intensity (, black circles). clearly demonstrates that the fluorescence intensity, in the absence of [Ca2+]o, dropped at some point after 15 min of incubation (open circles). In the presence of [Ca2+]o, this process began at some point of time after the 27th min. This difference of 12–13 min was probably due to the fact that, in the absence of [Ca2+]o, the high NO level fosters the mechanisms of cytosolic Ca2+ emptying. In other words, in the presence of high [Ca2+]o, first as a result of the high concentration gradient for Ca2+, the low NO level at the very beginning (t1/2 for SNAP decomposition is ∼37 h) initiates mechanisms that lead to an increase in cytosolic Ca2+ concentration. As intracellular Ca2+ grows, intracellular stores are replenished, and at some point, Ca2+ overload is achieved. Meanwhile, the partial pressure of NO increases and induces inhibition of LTCCs, and at the same time, fosters the restoration of intracellular Ca2+ homeostasis by induction of mechanisms for extracellular Ca2+ transport [Citation25]. Actually, in the absence of [Ca2+]o, the increased outflow of Ca2+ along the gradient and the Na+/Ca2+ exchanger can change the mode and stoichiometry of the Ca2+ transfer, which was not the purpose of this study. Taking into account the NO-driven parallel dynamics in the Ca2+ outflow in the presence or absence of [Ca2+]o, we assume that [Ca2+]i is mainly affected by the mechanisms of Ca2+ outflow rather than the mechanisms of Ca2+ inflow in the presence of NO. This is in line with previous reports that submicromolar concentration changes in the cytoplasmic Ca2+ concentration increase the peak amplitude of the outward Na+/Ca2+ exchange current, even in the condition of reduced electrochemical gradient of Ca2+ [Citation26, Citation27]. This reduced electrochemical gradient of Ca2+ in our case (empty circles in ), was the reason for the faster cytosolic Ca2+ emptying. Therefore, it seems that the time delay in the presence of [Ca2+]o was due to the increased electrochemical gradient of Ca2+.
Figure 3. Fura-signal dynamics. Normalized fluorescence: in conditions of 300 μmol/L SNAP (^), n = 21; in conditions of 1.8 mmol/L [Ca2+]o (•), n = 20; in conditions of 1.8 mmol/L [Ca2+]o and 300 μmol/L (SNAP), n = 21 (•); *p < .05. Note: Grey circles (•) show the baseline level of fluorescence recorded for 40 min without SNAP application (control conditions).
![Figure 3. Fura-signal dynamics. Normalized fluorescence: in conditions of 300 μmol/L SNAP (^), n = 21; in conditions of 1.8 mmol/L [Ca2+]o (•), n = 20; in conditions of 1.8 mmol/L [Ca2+]o and 300 μmol/L (SNAP), n = 21 (•); *p < .05. Note: Grey circles (•) show the baseline level of fluorescence recorded for 40 min without SNAP application (control conditions).](/cms/asset/c30358b6-f15b-4bdc-a3ff-2ec6a889c4c9/tbeq_a_1488621_f0003_b.jpg)
In all experiments from this setting, we obtained high standard errors when [Ca2+]i decreasing began, which may be an indicator of a partially dys-synchronous Ca2+ release. A similarly dys-synchronous Ca2+ release has been previously demonstrated in atrial cells [Citation28], in model systems with high background Ca2+ influxes [Citation29], in dedifferentiated myocytes [Citation25, Citation30] and in detubulated myocytes subjected to osmotic shock [Citation28]. Consistent with the important role of high Ca2+ influxes [Citation29] and based on the obtained signal dynamics in the presence of [Ca2+]o, our findings help us to conclude that despite its ability to influence the kinetics of [Ca2+]i decreasing, [Ca2+]o increases the NO driven dys-synchronicity of Ca2+ release from cardiomyocytes.
Limitations
One of the basic limitations of this study is that we did not clarify whether calmodulin is involved in the examined mechanisms. Also, additional experiments with Ca2+-troponin C should be performed to determine any additional signaling pathways affected by SNAP and/or [Ca2+]o.
Conclusions
This report is among the firsts that, based on the Fura-2 signal dynamics and phalloidin intensity profile between z-lines, examined [Ca2+]i changes in the presence of 300 μmol/L SNAP and/or 1.8 mmol/L [Ca2+]o. The results, addressed a possible role of the Na+/Ca2+ exchanger in the regulation of [Ca2+]i homeostasis during high NO production. This finding does not minimize the role of SR Ca2+ uptake, but could help fill the gap in the literature regarding the role of the Na+/Ca2+ exchanger, suggesting that in conditions of higher NO production, this transporter can play an important role in the regulation of the [Ca2+]i homeostasis in cardiomyocytes. Additional investigations should be done in order to be able to give full clarification of the role of the Na+/Ca2+ exchanger in conditions of increased NO production in cardiomyocytes.
Disclosure statement
No potential conflict of interests was reported by the author(s).
Additional information
Funding
References
- Benitah JP, Alvarez JL, Gomez AM. L-type Ca(2+) current in ventricular cardiomyocytes. J Mol Cell Cardiol. 2010;48:26–36.
- Tandan S, Wang Y, Wang TT, et al. Physical and functional interaction between calcineurin and the cardiac L-type Ca2+ channel. Circ Res. 2009;105:51–60.
- Heineke J, Auger-Messier M, Correll RN, et al. CIB1 is a regulator of pathological cardiac hypertrophy. Nat Med. 2010;16:872–879.
- Frank D, Frey N. Cardiac z-disc signaling network. J Biol Chem. 2011;286:9897–9904.
- Shim AL, Mitrokhin VM, Kazanski V, et al. Discrete stretch eliminates electrophysiological dose-dependent effects of nitric oxide donor SNAP in rat atrium. Bull Exp Biol Med. 2017;163:705–709.
- Shim AL, Mitrokhin VM, Gorbacheva LR, et al. Kinetics of mechanical stretch-induced nitric oxide production in rat ventricular cardiac myocytes. Bull Exp Biol Med. 2017;163:583–585.
- Kazanski EV, Kamkin GA, Makarenko YE, et al. Role of nitric oxide in the regulation of mechanosensitive ionic channels in cardiomyocytes: Contribution of NO-synthases. Bull Exp Biol Med. 2010;150:263–267.
- Gallo MP, Ghigo D, Bosia A, et al. Modulation of guinea-pig cardiac L-type calcium current by nitric oxidesynthase inhibitors. J Physiol. 1998;506:639–651.
- Gallo MP, Malan D, Bedendi I, et al. Regulation of cardiac calcium current by NO and cGMP-modulating agents. Pflugers Arch. 2001;441:621–628.
- Abi-Gerges N, Fischmeister R, Méry PF. G protein-mediated inhibitory effect of a nitric oxide donor on the L-type Ca2+ current in rat ventricular myocytes. J Physiol. 2001;531:117–130.
- Han S, Schiefer A, Isenberg G. Ca2+ load of guinea-pig ventricular myocytes determines efficacy of brief Ca2+ currents as trigger for Ca2+ release. J Physiol. 1994;480:411–421.
- Han ZY, Chen M, Wen P, et al. 8%-9% and 12%-13% hypoxic gas induced free radicals generation in rat's left and right myocardium. Sheng Li Xue Bao. 1995;47:453–462.
- Han J, Kim E, Ho WK, et al. Effects of volatile anesthetic isoflurane on ATP-sensitive K+ channels in rabbit ventricular myocytes. Biochem Biophys Res Commun. 1996;229(3):852–856.
- Kazanski V, Mitrokhin VM, Mladenov MI, et al. Cytokine effects on mechano-induced electrical activity in atrial myocardium. Immunol Invest. 2017;46:22–37.
- Kirstein M, Rivet-Bastide M, Hatem S, et al. Nitric oxide regulates the calcium current in isolated human atrial myocytes. J Clin Invest. 1995;95:794–802.
- Campbell DL, Stamler JS, Strauss HC. Redox modulation of L-type calcium channels in ferret ventricular myocytes. Dual mechanism regulation by nitric oxide and S-nitrosothiols. J Gen Physiol. 1996;108:277–293.
- Wahler GM, Dollinger SJ. Nitric oxide donor SIN-1 inhibits mammalian cardiac calcium current through cGMP-dependent protein kinase. Am J Physiol. 1995;268:45–54.
- Aksyonov A, Mitrokhin VM, Mladenov MI. Effects of interleukin-2 on bioelectric activity of rat atrial myocardium under normal conditions and during gradual stretching. Immunol Lett. 2015;167:23–28.
- Mitrokhin VM, Mladenov MI, Kamkin AG. Effects of interleukin-6 on the bio-electric activity of rat atrial tissue under normal conditions and during gradual stretching. Immunobiology. 2015;220:1107–1112.
- Ovchinnikov RS, Mitrokhin VM, Mladenov MI. Effects of interleukin-17A on the bio-electric activity of rat atrial myocardium under normal conditions and during gradual stretching. Cytokine. 2015;76:561–565.
- Mitrokhin V, Kazanski V, Kalsin V, et al. Interleukin-6 induced activation of a non-selective outward cation conductance in human cardiac fibroblasts. Cytokine. 2017;97:117–122.
- Rickover O, Zinman T, Kaplan D, et al. Exogenous nitric oxide triggers classic ischemic preconditioning by preventing intracellular Ca2+ overload in cardiomyocytes. Cell Calcium. 2008;43:324–333.
- Franzini-Armstrong C, Protasi F, Ramesh V. Shape, size, and distribution of Ca(2+) release units and couplons in skeletal and cardiac muscles. Biophys J. 1999;77:1528–1539.
- Kass SR, Sanguinetti CM. Inactivation of calcium channel current in the Calf cardiac purkinje fiber; evidence for voltage and calcium-mediated mechanisms. J Gen Physiol. 1984;84:705–726.
- Lipp P, Huser J, Pott L, et al. Spatially non-uniform Ca2+ signals induced by the reduction of transverse tubules in citrate-loaded guinea-pig ventricular myocytes in culture J Physiol (Lond). 1996;497:589–597.
- Matsuoka S, Nicoll DA, Hryshko LV, et al. Regulation of the cardiac Na(+)-Ca2+ exchanger by Ca2+. Mutational analysis of the Ca(2+)-binding domain. J Gen Physiol. 1995;105:403–420.
- Fujioka Y, Hiroe K, Matsuoka S. Regulation kinetics of Na+-Ca2+ exchange current in guinea-pig ventricular myocytes. J Physiol (Lond). 2000;529:611–623.
- Brette F, Salle L, Orchard CH. Differential modulation of L-type Ca2+ current by SR Ca2+ release at the T-tubules and surface membrane of rat ventricular myocytes. Circ Res. 2004;95:1–7.
- Diaz ME, O’Neill SC, Eisner DA. Sarcoplasmic reticulum calcium content fluctuation is the key to cardiac alternans. Circ Res. 2004;94:650–656.
- Louch WE, Bito V, Heinzel FR, et al. Reduced synchrony of Ca2+ release with loss of T-tubules a comparison to Ca2+ release in human failing cardiomyocytes. Cardiovasc Res. 2004;62:63–73.