Abstract
Use of thermotolerant strains offers the advantage of conducting production process at elevated temperatures, thereby improving the catalytic efficiency and reducing the energy consumption and the production costs. In the present study, thermotolerance gene groES from the thermophilic strain Bacillus licheniformis B186 was implanted into B. subtilis WB600 to improve its thermotolerance. The engineered strain WB600-pHY-groES displayed reduced doubling time as well as increased specific growth rates and cell viability compared with the control. Our results suggested that introducing heat shock proteins (HSPs) from thermophiles into B. subtilis could be an effective approach to enhance its thermotolerance, thus facilitating the development of multichaperone expression systems for constructing more thermotolerant commercial B. subtilis strains. To the best of our knowledge, this is the first report on improving the thermotolerance of B. subtilis by overexpression of HSPs.
Introduction
Bacillus subtilis, a rod-shaped, Gram-positive and spore-forming bacterium thriving in the soil, has been widely used in food [Citation1], feed [Citation2], pharmaceutical [Citation3], chemical [Citation4,Citation5] and biotechnological industries [Citation6]. For example, B. subtilis is used as a host for the production of commercial enzymes, pharmaceutical proteins, biofuels and fine chemicals due to its superior fermentation properties, generally regarded as safe (GRAS) status, high-level protein secretion capability (20–25 g/L), simplified downstream processes [Citation7], as well as other desirable features including the absence of significant codon bias and ease of genetic manipulation [Citation6].
During the fermentation processes, B. subtilis constantly faces a variety of environmental stresses, resulting in decreased production capacity. Therefore, improving its cellular robustness to various stresses is of great importance for efficient industrial production of biomolecules, especially enzymes, biochemicals and biofuels [Citation8]. Among the desirable traits of B. subtilis for industrial processes, thermotolerance is crucial for minimising the risk of microbial contamination, reducing cooling and product recovery costs [Citation9], and for shortening the fermentation period [Citation10]. To date, many strategies have been developed to obtain super strains exhibiting resistance to elevated temperatures, such as isolation and selection of strains from nature, mutagenesis, protoplast fusion as well as adaptive laboratory evolution. Besides, heat shock proteins (HSPs) are also considered essential components of microbial heat shock responses in that they protect cytoplasmic proteins from irrevocable misfolding, denaturation and aggregation caused by exogenous or biotic stresses. There are two major groups of HSPs, the 70-kDa DnaK family and 60-kDa GroE family [Citation11]. Chaperonins GroES and GroEL from the latter family are the extensively studied HSPs which play an important role in the thermotolerance of B. subtilis [Citation12]. Structural and biochemical studies have demonstrated that the co-chaperone GroES acts with its partner, GroEL, as a two-stroke ATP-regulated folding machine. In addition to preventing aggregation, the GroES-GroEL complex can also provide an enclosed environment for the correct folding of proteins under both normal growth and stressful conditions [Citation11,Citation13]. Therefore, overexpression of HSPs has been used as a promising technique to improve the thermotolerance of Saccharomyces cerevisiae [Citation8], Lactococcus lactis [Citation14–17], Bifidobacterium longum [Citation18], Escherichia coli [Citation19,Citation20] and Anabaena sp. [Citation21]. Although various aspects of the response of HSPs to thermal stress have been extensively studied in Bacillus species, their role in the improvement of thermotolerance is not well documented for B. subtilis.
Previously, the thermotolerance mechanism of the thermophilic strain B. licheniformis B186 was investigated by Dong et al. using tandem mass tag-based quantitative proteomics, and the groES gene encoding a 10 kDa chaperonin was implicated in its thermotolerance [Citation22]. In the present study, the groES gene from B. licheniformis B186 was cloned and heterologously overexpressed in B. subtilis WB600 to enhance its thermotolerance. The effects of groES overexpression on the cell growth and the viability of the engineered strain were then investigated. Our results showed that groES overexpression in B. subtilis WB600 significantly improved its thermotolerance, highlighting the utility of the groES gene for engineering efficient and robust industrial strains.
Materials and methods
Plasmids, primers, bacterial strains and gowth conditions
Plasmid pHY-WZX [Citation23] was used for gene cloning and expression. E. coli JM109 and B. subtilis WB600 stocked in our lab served as the hosts for gene cloning and expression, respectively. B. lichenformis B186 with an optimum growth temperature of 50 °C [Citation22] was purchased from CICIM-CU (Jiangnan University, China; http://cicim-cu.jiangnan.edu.cn). All the strains were cultured in Luria–Bertani (LB) medium (yeast extract 5 g/L, tryptone 10 g/L, NaCl 10 g/L). If necessary, 10 μg/mL kanamycin was added to the medium.
Chemicals and reagents
PyrobestTM DNA polymerase, T4 DNA ligase and restriction enzymes were purchased from TaKaRa Bio Inc. (Dalian, China). OMEGA Gel Extraction Kit and EZNATM Plasmid Mini Kit were supplied by OMEGA Bio-Tek (Norcross, GA, USA). EZ-10 Spin Column Genomic DNA Isolation Kit and ampicillin were the products of Bio Basic Inc. (Toronto, Canada) and Sangon Biotech Co., Ltd. (Shanghai, China), respectively. Yeast extract and tryptone were bought from OXOID (Basingstoke, England). All other chemicals used in this study were of analytical grade.
Cloning of groES gene from B. licheniformis B186
Genomic DNA of B. licheniformis B186 was isolated using EZ-10 Spin Column Genomic DNA Isolation Kit according to the instruction manual. The primers designed based on the published genome sequence of B. licheniformis ATCC 14580 (GenBank accession No. Q65MZ9.1) were as follows: groES1 (5′-TGCTCTAGATTGTTAAAGCCATTAGGTGATCGCG-3′) and groES2 (5′-GGGTTAGCCGATAACAGCTAAAATGTCGC-3′), where the restriction site of XbaI is underlined. The open reading frame (ORF) of the groES gene was then amplified by polymerase chain reaction (PCR) using genomic DNA from B. licheniformis B186 as a template and the primers groES1 and groES2. Amplification was conducted under the following programme: initial denaturation step at 95 °C for 5 min; 30 cycles of denaturation (96 °C for 30 s), annealing (58 °C for 30 s) and extension (72 °C for 1 min); and a final extension step at 72 °C for 10 min (Techne TC-512 thermocycler; Techne Co., Staffordshire, UK). The obtained PCR product was analysed by electrophoresis and purified by TaKaRa MiniBEST DNA Fragment Purification Kit Ver.4.0 (Dalian, China).
Construction of recombinant bacteria
The purified groES PCR fragment was cut by XbaI and ligated with XbaI- and SmaI-digested plasmid pHY-WZX, generating the recombinant plasmid pHY-groES. This recombinant plasmid was subsequently transformed into E. coli JM109 competent cells and positive clones were screened on LB plates supplemented with 10 μg/mL kanamycin. After the correctness of plasmid construction was confirmed by restriction analysis and DNA sequencing (Sangon Biotech Co., Ltd., Shanghai, China), the recombinant plasmid pHY-groES and the corresponding control plasmid pHY-WZX were transformed into B. subtilis WB600 competent cells. Recombinant B. subtilis WB600 strains harbouring plasmid pHY-groES (designated WB600-pHY-groES) or pHY-WZX (control, designated WB600-pHY-WZX) were confirmed by colony PCR and plasmid DNA extraction.
Determination of cell viability and cell concentration
Single colonies of B. subtilis WB600-pHY-WZX and WB600-pHY-groES were grown at 37 °C for 6 h in LB medium suplemented with kanamycin. To investigate the thermotolerane of B. subtilis WB600, the two cultures were then diluted to an optical density at 600 nm (OD600) of 1.0 and cultured at 37, 42, 50 and 55 °C for 1 h. After 10-fold serial dilutions, each of these cultures was spread on LB-kanamycin plates and then aerobically incubated at 37 °C for 12 h to observe the growth of the bacteria.
To monitor the growth of B. subtilis WB600 under long-term stress conditions, single colonies of B. subtilis WB600-pHY-groES and WB600-pHY-WZX were inoculated into 250 mL-Erlenmeyer flasks containing 25 mL LB media, and cultured at 37 °C and 200 r/min for 12 h. An aliquot of the resulting culture was inoculated in 250 mL shake flasks containing 50 mL LB medium, starting with an OD600 of 0.1 for further cultivation at 37, 42, 50 and 55 °C for 12 h, and the cell samples were taken at 2 h-intervals to measure OD600. Doubling time was computed by the online tool (http://www.doubling-time.com/compute.php), according to the duration time, and the initial and final cell concentrations. All data are mean values with standard deviation (±SD) from three independent experiments.
Quantitative real-time PCR
Total RNA from exponentially growing (OD600 of 0.8–1.0) B. subtilis strains was isolated with the RNAprep pure Plant Kit (Tiangen Biotech (Beijing) Co. Ltd., Beijing, China) and then used for cDNA synthesis according to the HiFiScript gDNA Removal cDNA Synthesis Kit (CWBIO Co. Ltd., Beijing, China). Primer pairs (groES-F: 5′-TCGTACTTCCTGACTCCGCAA-3′ and groES-R: 5′-TGATGCGGTCGCCTGTTT-3′) were designed and synthesised to amplify 123-bp regions of groES for quantitative reverse transcription PCR (qRT-PCR). Real-Time PCR was carried out in a 20 μL (total volume) mixture containing 9 μL of SYBR® Premix Ex Taq™ II (2.5×), 0.5 μL each of the forward and the reverse primer (10 μmol/L) and 1 μL of the cDNA sample. The detection was performed as follows: incubation at 95 °C for 10 s, 33 cycles at 9 5 °C for 5 s, 51 °C for 15 s (Step One System, ABI, USA). The transcriptional level was normalized by the ΔΔCT method [Citation24], with the house-keeping 16S ribosomal RNA as the reference gene for the calculations.
Statistical analysis
Statistical Package for Social Scientists (SPSS) version 19.0 (IBM, Armonk, New York, USA) was used to investigate statistical differences. Values of P < 0.05 were considered significant.
Results and discussion
Overexpression of groES in B. subtilis WB600
In nature, thermophiles show superior robustness under harsh conditions, possibly due to their well-adapted stress-response genes during long-term natural evolution [Citation25], such as molecular chaperones [Citation22]. Overexpression of molecular chaperones, such as HSPs, has been considered as one of the most promising approaches for improving microbial strain tolerance to a variety of stresses, including heat shock [Citation8,Citation15]. In this study, to enhance the thermotolerance of B. subtilis WB600, the groES gene from the thermophilic strain B. licheniformis B186 was introduced into this strain. As described above, an approximately 300-bp PCR product, consistent with the theoretical length of groES (285 bp), was agarose gel-purified and used to construct the expression plasmid pHY-groES, followed by confirmation via sequencing. The 285-bp ORF of groES is predicted to encode 94 amino acids with a theoretical molecular weight of 10.1 kDa. Plasmids pHY-WZX and pHY-groES were then respectively transformed into B. subtilis WB600, generating corresponding recombinant strains WB600-pHY-WZX and WB600-pHY-groES. In the exponential phase of B. subtilis WB600-pHY-groES, the transcriptional levels of groES were 7.4-fold higher than those in WB600-pHY-WZX as determined by qRT-PCR (), suggesting that groES was successfully overexpressed in B. subtilis WB600-pHY-groES.
Figure 1. Relative expression levels of groES in B. subtilis WB600-pHY-WZX and WB600-pHY-groES determined by qRT-PCR. Note: The relative transcription levels of groES were normalised to the transcription level of the 16S rRNA gene. The presented values are averages of three independent experiments. The error bars indicate standard deviations.
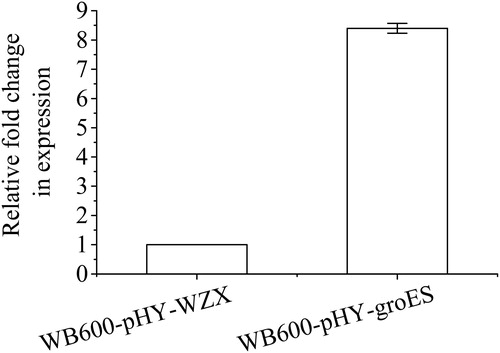
Thermotolerance of groES-overexpressing B. subtilis WB600-pHY-groES
The growth behaviour of the recombinant and control strains was investigated in LB liquid medium supplemented with kanamycin by exposure to 37 °C, 42 °C, 50 °C and 55 °C for 1 h, followed by incubation at 37 °C for 12 h. As shown in , the cell viability dramatically decreased with increasing temperature, and the recombinant strain B. subtilis WB600-pHY-groES showed relatively higher cell viability than the control cultured at the same temperature.
Figure 2. Growth phenotype of transformants containing plasmids pHY-groES or pHY-WZX after exposure to different temperatures. Note: Exposure to different temperatures for 1 h, 10-fold serial dilution and cultivation at 37 °C for 12 h.
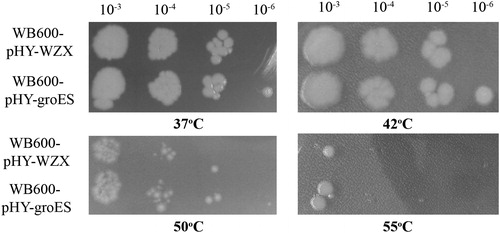
The experimental analysis of thermotolerance was extended to a study of long-term growth at the aforementioned four temperatures with monitoring at 2-h intervals. Samples were analysed for OD600 and doubling time and the results are summarised in and , respectively. Variations were observed in both groES-overproducing and control strains. When cultured at the same temperature, the specific growth rate of B. subtilis WB600-pHY-groES was higher than that of the control, and their final cell densities were similar except for cultivation at 37 °C. Furthermore, the fermentation periods for both strains decreased with increasing temperature, and the fermentation period of B. subtilis WB600-pHY-groES was shorter than or similar to that of the parent strain (). As shown in , the doubling time of the engineered strain was also shorter than that of the control strain. These results demonstrated that overexpression of groES had a positive effect on the thermotolerance of B. subtilis WB600.
Figure 3. Growth profiles of B. subtilis WB600-pHY-WZX and WB600-pHY-groES cultured at 37 °C (a), 42 °C (b), 50 °C (c), and 55 °C (d) for 12 h. Filled square and unfilled circle represent B. subtilis WB600-pHY-WZX and WB600-pHY-groES, respectively.
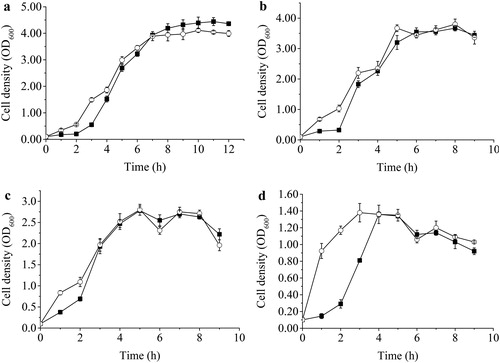
Table 1. Doubling time of B. subtilis WB600-pHY-WZX and WB600-pHY-groES cultured at different temperatures.
The misfolding of intracellular proteins is considered to be a main factor making microorganisms inactive under thermal stress, although other factors such as membrane fluidity also affect their thermotolerance [Citation26]. There have been many reports showing that overexpression of HSPs such as GroESL can enhance microbial thermotolerance, which may be due to the folding or refolding activity of the HSPs towards misfolded cellular proteins under thermal stress [Citation13]. Our study may also be one of the examples to show that enhanced microbial thermotolerance can be achieved by the overexpression of HSPs. Besides enhancing the viability of microorganisms under heat shock, GroESL overexpression can also improve diverse types of stress-tolerance of different microbes, including antibiotic resistance [Citation27], stresses of solvents, salinity, drying, etc. [Citation19,Citation21,Citation28,Citation29], thus expanding the applicability of industrial microorganisms, such as B. subtilis WB600.
However, although overproduction of the groES gene improved the thermotolerance of B. subtilis WB600, the final cell concentration decreased with increased temperature (). Further studies are therefore required to increase the final cell density of this thermotolerant strain. As groES genes from extremophiles are superior to those from mesophilic bacteria and they perform quite differently in different hosts [Citation19], the relation and compatibility between the host and extremophilic bacteria must be considered for selecting the proper groES to engineer microbial robustness. Besides, heat shock response usually involves a number of proteins, such as HrcA, DnaK, DnaJ, HtpG, FtsH and GroEL chaperone proteins, and they usually cooperate with each other [Citation12,Citation18,Citation22,Citation30]. Therefore, overexpression of an entire battery of these thermal stress-related proteins may give better cellular survival at elevated temperatures, since it would more closely mimic the heat adaptation response of B. subtilis.
Conclusions
In the present study, to construct a thermotolerant B. subtilis strains, the groES gene was cloned from B. licheniformis B186 and overexpressed in B. subtilis WB600. Our results suggested that overexpression of groES showed the potential to improve the capacity of B. subtilis to withstand thermal stress conditions, reinforcing the notion that overexpression of HSPs in B. subtilis plays an important role in improving the tolerance of cells to heat stress and thus the cell viability. Knowledge gained from this work will also facilitate the development of multichaperone overexpression systems to generate more robust commercial B. subtilis strains for the food industry.
Abbreviations | ||
HSPs | = | heat shock proteins |
GRAS | = | generally regarded as safe |
LB | = | Luria-Bertani |
ORF | = | open reading frame |
PCR | = | polymerase chain reaction |
OD600 | = | optical density at 600 nm |
qRT-PCR | = | quantitative reverse transcription PCR |
SPSS | = | Statistical Package for Social Scientists |
Disclosure statement
No potential conflict of interest was reported by the authors.
Additional information
Funding
References
- Farzaneh M, Shi ZQ, Ghassempour A, et al. Aflatoxin B1 degradation by Bacillus subtilis UTBSP1 isolated from pistachio nuts of Iran. Food Control 2012;23(1):100–106.
- Liu CH, Chiu CH, Wang SW, et al. Dietary administration of the probiotic, Bacillus subtilis E20, enhances the growth, innate immune responses, and disease resistance of the grouper, Epinephelus coioides. Fish Shellfish Immunol. 2012;33(4):699–706.
- Rosalesmendoza S, Angulo C Bacillus subtilis comes of age as a vaccine production host and delivery vehicle. Expert Rev Vaccines. 2015;14(8):1135–1148.
- Guan Z, Xue D, Abdallah II, et al. Metabolic engineering of Bacillus subtilis for terpenoid production. Appl Microbiol Biotechnol. 2015;99(22):9395–9406.
- Zhang XZ, Zhang YHP. One-step production of biocommodities from lignocellulosic biomass by recombinant cellulolytic Bacillus subtilis: opportunities and challenges. Eng Life Sci. 2010;10(5):398–406.
- Ozturk S, Calik P, Ozdamar TH. Fed-batch biomolecule production by Bacillus subtilis: a state of the art review. Trends Biotechnol. 2016;34(4):329–345.
- van Dijl JM, Hecker M. Bacillus subtilis: from soil bacterium to super-secreting cell factory. Microb Cell Fact. 2013;12:3.
- Liu Y, Zhang G, Sun H, et al. Enhanced pathway efficiency of Saccharomyces cerevisiae by introducing thermo-tolerant devices. Bioresour Technol. 2014;170:38–44.
- Lin L, Xu J. Dissecting and engineering metabolic and regulatory networks of thermophilic bacteria for biofuel production. Biotechnol Adv. 2013;31(6):827–837.
- Postmus J, Aardema R, de Koning LJ, et al. Isoenzyme expression changes in response to high temperature determine the metabolic regulation of increased glycolytic flux in yeast. FEMS Yeast Res. 2012;12(5):571–581.
- Georgopoulos C, Welch WJ. Role of the major heat shock proteins as molecular chaperones. Annu Rev Cell Biol. 1993;9:601–634.
- Schumann W. The Bacillus subtilis heat shock stimulon. Cell Stress Chaperones. 2003;8(3):207–217.
- Hayer-Hartl M, Bracher A, Hartl FU. The GroEL-GroES chaperonin machine: a nano-cage for protein folding. Trends Biochem Sci. 2016;41(1):62–76.
- Desmond C, Fitzgerald GF, Stanton C, et al. Improved stress tolerance of GroESL-overproducing Lactococcus lactis and probiotic Lactobacillus paracasei NFBC 338. Appl Environ Microbiol. 2004;70(10):5929–5936.
- Abdullah Al M, Sugimoto S, Higashi C, et al. Improvement of multiple-stress tolerance and lactic acid production in Lactococcus lactis NZ9000 under conditions of thermal stress by heterologous expression of Escherichia coli DnaK. Appl Environ Microbiol. 2010;76(13):4277–4285.
- Weidmann S, Maitre M, Laurent J, et al. Production of the small heat shock protein Lo18 from Oenococcus oeni in Lactococcus lactis improves its stress tolerance. Int J Food Microbiol. 2017;247:18–23.
- Tian H, Tan J, Zhang L, et al. Increase of stress resistance in Lactococcus lactis via a novel food-grade vector expressing a shsp gene from Streptococcus thermophilus. Braz J Microbiol. 2012;43(3):1157–1164.
- Khaskheli GB, Zuo F, Yu R, et al. Overexpression of small heat shock protein enhances heat- and salt-stress tolerance of Bifidobacterium longum NCC2705. Curr Microbiol. 2015;71(1):8–15.
- Luan G, Dong H, Zhang T, et al. Engineering cellular robustness of microbes by introducing the GroESL chaperonins from extremophilic bacteria. J Biotechnol. 2014;178:38–40.
- Ezemaduka AN, Yu J, Shi X, et al. A small heat shock protein enables Escherichia coli to grow at a lethal temperature of 50 °C conceivably by maintaining cell envelope integrity. J Bacteriol. 2014;196(11):2004–2011.
- Chaurasia AK, Apte SK. Overexpression of the groESL operon enhances the heat and salinity stress tolerance of the nitrogen-fixing cyanobacterium Anabaena sp. strain PCC7120. Appl Environ Microbiol. 2009;75(18):6008–6012.
- Dong Z, Chen Z, Wang H, et al. Tandem mass tag-based quantitative proteomics analyses reveal the response of Bacillus licheniformis to high growth temperatures. Ann Microbiol. 2017;67(7):501–510.
- Niu D, Wang ZX. Development of a pair of bifunctional expression vectors for Escherichia coli and Bacillus licheniformis. J Ind Microbiol Biotechnol. 2007;34(5):357–362.
- Schmittgen TD, Livak KJ. Analyzing real-time PCR data by the comparative C(T) method. Nat Protoc. 2008;3(6):1101–1108.
- Egorova K, Antranikian G. Industrial relevance of thermophilic Archaea. Curr Opin Microbiol. 2005;8(6):649–655.
- Kim SY, Ayyadurai N, Heo MA, et al. Improving the productivity of recombinant protein in Escherichia coli under thermal stress by coexpressing GroELS chaperone system. J Microbiol Biotechnol. 2009;19(1):72–77.
- Goltermann L, Sarusie MV, Bentin T. Chaperonin GroEL/GroES over-expression promotes aminoglycoside resistance and reduces drug susceptibilities in Escherichia coli following exposure to sublethal aminoglycoside doses. Front Microbiol. 2015;6:1572.
- Zingaro KA, Terry Papoutsakis E. GroESL overexpression imparts Escherichia coli tolerance to i-, n-, and 2-butanol, 1,2,4-butanetriol and ethanol with complex and unpredictable patterns. Metab Eng. 2013;15:196–205.
- Xia P, Turner TL, Jayakody LN. The role of GroE chaperonins in developing biocatalysts for biofuel and chemical production. Enzyme Eng. 2016;5:153.
- Hecker M, Schumann W, Volker U. Heat-shock and general stress response in Bacillus subtilis. Mol Microbiol. 1996;19(3):417–428.