Abstract
Vacuolar Na+/H+ antiporters (NHXs) play an important role in plant salt tolerance and a large number of NHX genes have been identified in many plant species. In this study, we isolated and characterized a novel vacuolar-type KvNHX1 cDNA from the halophyte Kosteletzkya virginica. It is 2047 bp long, with an open reading frame of 1632 bp, encoding a protein of 543 amino acids with a theoretical molecular weight of 60.03 kDa. Bioinformatics and phylogenetic analysis showed that it was highly similar to the known vacuolar-type NHXs, especially with 92% identity with GhNHX1 of Gossypium hirsutum from the same Malvaceae family. Quantitative RT-PCR showed that the expression analysis of KvNHX1was significantly increased by NaCl, abscisic acid (ABA) and polyethylene glycol (PEG), but its expression levels and patterns differed under different abiotic stresses, implying that KvNHX1 might be involved in various abiotic stress responses. In addition, overexpression of KvNHX1 enhanced the salt tolerance in transgenic tobacco lines with better growth, more chlorophyll, higher antioxidant enzyme activities and osmoregulation capability by accumulating more proline, Na+ and K+ in the leaves than the wild type under salt stress. Our result suggested that KvNHX1 may have potential use for improving the salt tolerance of plants via genetic engineering.
Introduction
Soil salinization is becoming a worldwide environmental problem that severely damages the fertility of soil and crop productivity. According to some statistics, the salt-affected cultivated lands account for a significant proportion of global cultivated lands and over one-third of irrigated lands are subjected to different degrees of salinization, resulting in tremendous loss in grain production and agricultural sustainability [Citation1,Citation2]. To guarantee food security for the burgeoning world population, it is pivotal to breed salt-tolerant crops that can grow in salt-affected farmland and other marginal lands such as coastal regions [Citation3–5]. Under salt stress, the highly water-soluble salts (mainly NaCl) in the soil cause osmotic stress, which hinders plant roots from absorbing water. Excess Na+ absorbed into the cytosol of plants results in ionic toxicity and oxidative stress which further disturb many important physiological and metabolic processes [Citation1,Citation6]. To survive in such a saline environment, plants have developed some elaborate mechanisms to prevent excessive Na+ accumulation in the cytosol, including restricting the influx of environmental Na+, increasing the efflux of Na+ from the cell and compartmentalizing Na+ into vacuoles from the cytosol. These processes are largely achieved by some transporters such as Na+/H+ antiporters (NHXs) located in the vacuolar membrane and the plasma membrane. The plasma membrane NHXs (usually designated SOS1) expel Na+ from the cytosol, while the vacuolar membrane NHXs transport Na+ into vacuoles [Citation7–9]. The compartmentation of Na+ in vacuoles not only relieves the toxic effect of Na+ on the cytosolic metabolism, but also serves as an osmoticum to lower the osmotic potential for water uptake and turgor maintenance [Citation1,Citation9,Citation10]. So vacuolar compartmentation of Na+ is an important strategy for salt tolerance and NHX genes encoding vacuole-type NHXs, which perform this function, have attracted a lot of research.
Since AtNHX1 from Arabidopsis thaliana was confirmed to improve salt tolerance of transgenic plants [Citation11,Citation12], a large number of vacuolar NHX genes have been isolated from many plant species such as rice [Citation13], cotton [Citation14], maize [Citation15], wheat [Citation16], Brassica napus [Citation17], chrysanthemum [Citation18] and other plant species. Some of these vacuolar NHX genes have been transformed in different plant species to improve their salt tolerance [Citation19]. For example, transgenic Arabidopsis respectively with TaNHX1, SsNHX1 and MsNHX1 showed enhanced salt tolerance as compared to wild-type (WT) control plants [Citation20–22]. Transgenic tobacco with GhNHX1 and BnNHX1 showed improved plant growth under salt stress [Citation14,Citation23]. Transgenic rice with AgNHX1 showed salt tolerance and exhibited increased survival of seedlings under salt stress [Citation24]. Transgenic wheat with AtNHX1 showed increased shoot and root dry weight under salt stress, and transgenic maize with AtNHX1 exhibited improved germination under salt stress [Citation25,Citation26]. All these results indicate that vacuolar NHX genes play important roles in plant salt tolerance and the introduction of NHX genes in transgenic plants has been effective in improving salt tolerance. Halophytes have developed perfect strategies to cope with high salinity and their high salt tolerance may partly be attributed to the high efficiency of vacuolar NHXs as compared to glycophytic homologs [Citation19,Citation27]. For example, transgenic plants with AgNHX1 (Atriplex gmelini), SsNHX1 (Suaeda salsa) or SaNHX1 (Spartina anglica) show tolerance up to 300–400 mmol L−1 NaCl compared to glycophytic counterparts [Citation24,Citation28,Citation29]. But for now, research on vacuolar NHX genes from halophytes is still limited and further research on the isolation of new vacuolar NHX genes from various halophyte species should be performed.
Kosteletzkya virginica is a halophytic plant native to coastal areas containing 0.3 to 2.5% sodium salt [Citation30]. Because of its economic values and excellent salt tolerance, this species has been recommended as a potential cash crop for saline agriculture. In our previous studies, the comparative transcriptome analysis of NaCl-treatment seedlings provided a large amount of salt-stress responsive genes [Citation31]. Thus, this work aimed to isolate a new vacuolar NHX gene from Kosteletzkya virginica and investigate its properties.
Materials and methods
Plant material and growth conditions
The seeds of Kosteletzkya virginica were collected from the Ecological Experiment Station of the Chinese Academy of Sciences at Yellow River Delta, Shandong Province, China. The germinated seeds were planted in plastic pots containing vermiculite and were irrigated with 1/2 Hoagland nutrient solution. Seedlings were cultured in an artificial greenhouse at an average temperature of 25 °C with 14 h light/10 h dark, 65% relative humidity and sufficiently watered with 1/2 Hoagland nutrient solution every 3 days. Two weeks old seedlings were used for further study.
Cloning and sequence analysis of KvNHX1
Using “sodium/hydrogen exchanger” as a key word to search differentially expressed genes in the transcriptome sequencing data of K. virginica, a total of 11 unigenes up-regulated more than three times were found. Through multiple sequence alignment of these unigenes using the BLAST program at the National Center for Biotechnology Information (NCBI) website, a candidate NHX gene sequence (Locus_26339_Transcript_6/9) with the longest open reading frame (ORF) was obtained and designated KvNHX1. After analysing its cDNA sequence by the ORF Finder program (NCBI), a pair of specific primers (KvNHX1-F and KvNHX1-R) were designed to clone a fragment including the longest ORF (sequences given in ).
Table 1. Primers used in the present study.
Total RNA was extracted from K. virginica seedlings with RNAiso Plus (TaKaRa, Japan) and its quality and quantity were checked by NanoDrop™ 2000/c Spectrophotometer (Thermo Scientific, USA). The first-strand cDNA was synthesized using a cDNA synthesis kit (TransGen AT321-01, China) and was used as the template to amplify KvNHX1 by polymerase chain reaction (PCR). The PCR products were purified and ligated into the pEASYTM-Blunt Zero cloning vector (TransGen CB501-02, China) and sequenced. Then the fragment was identified by alignment with the candidate NHX gene sequence mentioned above from the transcriptome database and analyzed by the BLAST program on NCBI.
The nucleotide and deduced amino acid sequences of KvNHX1 were analyzed with DNAMAN software and the BLAST program on NCBI. The conserved domain was scanned by CDD on NCBI. Transmembrane domains were predicted by TMpred software (https://embnet.vital-it.ch/software/TMPRED_form.html). Multiple sequence alignment was conducted among the deduced KvNHX1 protein and other homologous proteins with high identity by the Clustal X 2.1 program. Based on the multiple sequence alignment, the phylogenetic tree was constructed using the MEGA 5.0 program by the neighbour-joining method.
Stress treatment and expression analysis of KvNHX1
Two-week-old and homogeneous seedlings were treated with 1/2 Hoagland nutrient solution containing 300 mmol L−1 NaCl, 15% polyethylene glycol (PEG 6000) and 100 μmol L−1 abscisic acid (ABA), respectively, and then used for the expression analysis of KvNHX1 by quantitative real-time PCR (qRT-PCR) according to the instructions of SYBR®Green Realtime PCR Master Mix (Applied Biosystems, USA) as previously described [Citation32]. The treated seedlings were sampled at 0, 3, 6, 12, 24 and 48 hours and seedlings treated with 1/2 Hoagland nutrient solution were used as controls. Each treatment had three pot replications and six homogeneous seedlings from each pot were mixed together as a replication. All samples were rapidly frozen with liquid nitrogen and stored at –80 °C.
The total RNA of these samples was extracted with RNAiso Plus (TaKaRa, Japan) and the first-strand cDNA was synthesized with TransScript One-Step gDNA Removal and cDNA Synthesis SuperMix (TransGen AT311-02, China). The KvEF1-α gene [Citation33] was used as the reference gene and the specific primer sequences of KvEF1-α and KvNHX1 for qRT-PCR are listed in . The relative expression levels were quantified by the 2–ΔΔCT method [Citation34].
Generation and identification of transgenic tobacco over-expressing KvNHX1
The ORF of KvNHX1 was amplified by PCR and the restriction site was added with the forward primer KvNHX1-ORF-F carrying a restriction site for BamHI and the reverse primer KvNHX1-ORF-R carrying a restriction site for SalI (). The amplified fragments were ligated into the pEASYTM-Blunt Zero cloning vector (Transgen CB501-02, China) and sequenced. The ORF of KvNHX1 was digested from the identified cloning vector with BamHI/SalI and then ligated into the BamHI/SalI digested binary vector pBI121 under the control of the CaMV35S promoter. The recombinant vector pBI121-KvNHX1 was then introduced to Agrobacterium tumefaciens strain GV1301 by the freeze-thaw transformation method [Citation35] and was used for tobacco transformation. Transgenic tobacco plants were generated by Agrobacterium-mediated leaf disk transformation [Citation36] and were selected on Murashige and Skoog (MS) medium supplemented with 50 mg L−1 kanamycin during in vitro regeneration. The kanamycin-resistant lines were verified by PCR and RT-PCR with the specific primers (KvNHX1-ORF-F and KvNHX1-ORF-R).
Total RNA extraction from tobacco and first-strand cDNA synthesis referred to the above method used for K. virginica. The expression level of KvNHX1 in putative transgenic plants was also analyzed by the qRT-PCR used for the above KvNHX1 expression analysis. The NtEF-α gene was used as the reference gene and the specific primer sequences for qRT-PCR are listed in . The transgenic tobacco lines with high expression levels of KvNHX1 were selected to produce T1 generation seeds by self-pollination for further salt tolerance analysis.
Salt tolerance analysis of transgenic tobacco carrying KvNHX1
The surface-sterilized T1 seeds of transgenic lines (N4 and N7) were sown onto MS medium supplemented with 50 mg L−1 kanamycin and WT seeds were sown onto MS medium. Two-week-old seedlings of kanamycin-resistant N4 and N7 lines were positively confirmed by genomic PCR detection using the specific primers of KvNHX1. Then, these positive transgenic T1 seedlings (N4 and N7 lines) and WT seedlings were transferred to plastic pots containing vermiculite and were irrigated with 1/2 Hoagland nutrient solution. All the tobacco seedlings were cultured in the same conditions as those described for the K. virginica seedlings above. At the stage of 5–6 leaves, these seedlings were irrigated with 1/2 Hoagland nutrient solution containing 300 mmol L−1 NaCl and with only 1/2 Hoagland nutrient solution used as controls, respectively. After treatment for 14 days, their physiological and biochemical parameters were evaluated including fresh weight, dry weight, chlorophyll content, proline content, malondialdehyde (MDA) content and the activities of peroxidase (POD), superoxide dismutase (SOD) according to the method described in our previous study [Citation37]. The Na+ and K+ contents were determined by atomic absorption spectrophotometry as described by Yu et al. [Citation38].
Statistical analysis
All the data are presented as mean values from three replications. The standard deviation (±SD) and the significant differences among the means were calculated and analyzed using analysis of variance (ANOVA) followed by least significant difference tests (LSD).
Results and discussion
Cloning and sequence analysis of KvNHX1
The cloned cDNA of KvNHX was 2057 bp in length, consisting of an 1632-bp ORF, a 307-bp 5’-untranslated region (UTR) and a 118-bp 3’-UTR (GeneBank accession MG732927). Sequence alignment analysis revealed that the obtained sequence of KvNHX1 was indeed a part of the candidate NHX from the transcriptome database. It encoded a predicted protein of 543 amino acids with a theoretical molecular weight of 60.03 kDa and a theoretical pI of 6.50. The BLASTp result revealed that the putative protein belonged to the sodium/hydrogen exchanger superfamily () and shared 80 ∼ 92% identity with the known NHXs from other plants, especially 92% identity with GhNHX1 (NP_001313722.1) from Gossypium hirsutum, 90% identity with DzNHX (XP_022718946.1) from Durio zibethinus and CcNHX (OMO58010.1) from Corchorus capsularis. Hydropathy plot analysis by the TMpred program indicated that KvNHX1 had 12 transmembrane helices (TM1–TM12) in the hydrophobic N-terminal region and a longer hydrophilic C-terminal region (), the third transmembrane region (TM3) containing the amiloride-binding motif ‘FFIYLLPPII’ (), which was consistent with other vacuolar membrane NHXs [Citation14,Citation39]. Phylogenetic relationship analysis showed that KvNHX1 was clustered together with vacuolar-type NHX homologs and most closely to GhNHX1 of Gossypium hirsutum from the same Malvaceae family ().
Figure 1. Bioinformatics analysis of KvNHX1. (a) Conserved domain analysis of KvNHX1. (b) Hydrophilic analysis of KvNHX1. TM1–TM11 represent transmembrane helices. (c) Alignment of KvNHX1 protein sequences with homologous sequences of other species. TM1–TM11 transmembrane domains of KvNHX1 are shown by thick lines above the sequences and the amiloride-binding domain is framed with a red box in TM3. The accession numbers of the protein sequences were as follows: GhNHX1 (NP_001313722.1), DzNHX2 (XP_022718946.1), CcNHX1 (AAT36679.1), HuNHX2 (XP_021281573.1), TcNHX (EOY10658.1), JcNHX2 (XP_012089169.1), PeNHX (AQN76291.1). (d) Phylogenetic analysis of KvNHX1. The accession numbers of the protein sequences were as follows: GhNHX1 (NP_001313722.1), CcNHX1 (AAT36679.1), DzNHX2 (XP_022718946.1), HuNHX2 (XP_021281573.1), TcNHX (EOY10658.1), GmNHX (AAR27596.1), JcNHX2 (XP_012089169.1), RcNHX2 (NP_001310702.1), PeNHX (AQN76291.1), PtNHX1 (AAV84586.1).
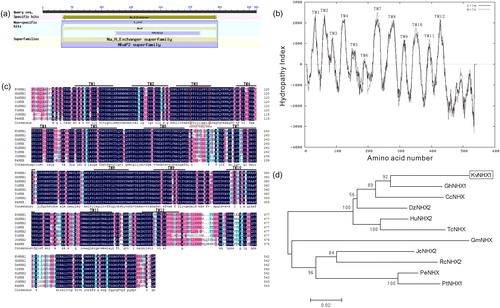
Expression analysis of KvNHX1 under abiotic stress by qRT-PCR
Quantitative RT-PCR showed that the expression analysis of KvNHX1 was significantly increased by NaCl, ABA and PEG, but its expression levels and patterns differed under different abiotic stresses. Under 300 mmol L−1 NaCl stress, the expression of KvNHX1 was significantly increased and peaked at 12 h and 24 h, the increase being 11.70-fold and 10.94-fold compared to the control, respectively. There was a decrease, however, at 48 h to 6.22 times that of control (). Its expression was also induced by 100 мmol L−1 ABA and reached the highest level (10.29-fold) at 6 h and then decreased to a stable level (). For 15% PEG 6000 stress, its expression gradually increased from 3 h to 24 h and peaked at 24 h (8.81-fold), then there followed a decline at 48 h (). In particular, the ABA-signalling pathway is important for plant tolerance to abiotic stresses including salt, drought, freezing, etc. It has been proved that the expression of NHX genes from Arabidopsis thaliana, Thellungiella halophila and Gossypium hirsutum was induced by ABA and involved an ABA-dependent pathway [Citation14,Citation40,Citation41]. Our results also showed that KvNHX1 expression was strongly induced by ABA treatment, suggesting that KvNHX1 might be involved in an ABA-dependent pathway and may act as a downstream gene on this signalling pathway in response to salt stress. In future studies, the promoter of KvNHX1 will be examined to identify some cis-elements responsive to salt, ABA and other stresses.
Figure 2. Expression analysis of KvNHX1 in leaves of Kosteletzkya virginica seedlings under abiotic stress by qRT-PCR. (a) 300 mmol L−1 NaCl treatment. (b) 100 μmol L−1 ABA treatment. (c) 15% PEG 6000 treatment. Note: Each data point represents the average of three replications and the error bars represents standard deviations based on three replications.
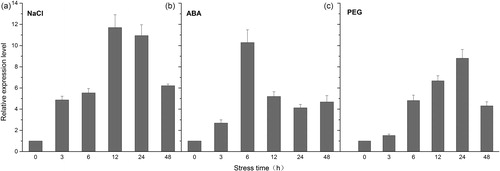
Generation and identification of transgenic tobacco
The recombinant vector pBI121-KvNHX1 was constructed and transformed into tobacco plants by the Agrobacterium-mediated method. After 30-day screening in vitro regeneration, a few kanamycin-resistant buds were induced from transgenic leaf discs on differentiation medium supplemented with 50 mg L−1 kanamycin. Then these positive buds were transferred to rooting medium supplemented with 50 mg L−1 kanamycin and finally 10 robust transgenic plants (designated N1–N10) were obtained. Genomic PCR detection using the specific primers of KvNHX1 showed 1600-bp bands in all these transgenic seedlings except WT (). RT-PCR analysis showed that the same bands were detected in DNA-PCR positive plants, but not in WT (), implying that KvNHX1 was integrated and expressed in the transgenic lines (N1–N10). Furthermore, qRT-PCR analysis indicated that the expression levels of KvNHX1 in N4 and N7 plants were higher than those in other plants (). Therefore, N4 and N7 transgenic lines were selected to produce T1 seeds for further analysis.
Salt tolerance analysis of transgenic tobacco
Salt stress can inhibit plant growth by causing osmotic stress, ionic stress and secondary oxidative stress with the overproduction of reactive oxygen species (ROS). It is thought that osmotic adjustment, ion compartmentation and antioxidant abilities are closely related to the salt tolerance of plants. Previous studies have shown that overexpression of NHX genes can improve the salt tolerance of transgenic plants, probably by maintaining ion homeostasis and enhancing the capacity of osmotic and antioxidant regulation [Citation42–44]. In this study, overexpression of KvNHX1 also conferred salt tolerance in transgenic tobacco lines. Under normal conditions, there was no significant difference in the chlorophyll content, fresh weight and dry weight between WT and transgenic lines. However, salt stress reduced the levels of these indices in all the seedlings, but the levels in the transgenic lines were higher than those in the WT plants (). In contrast, the proline content, MDA content and the activities of SOD and POD were also similar between WT and transgenic lines under normal conditions, but salt stress increased their levels in all the seedlings. In addition, the proline content and the activities of SOD and POD in the transgenic lines were higher than those in WT, except MDA content, which was lower than that in WT (). The above results showed that transgenic tobacco lines had stronger antioxidant enzyme activities and stronger osmoregulation capability than WT ones.
Figure 5. Proline content (a), MDA content (b) and the activities of SOD (c) and POD (d) in WT and transgenic lines.
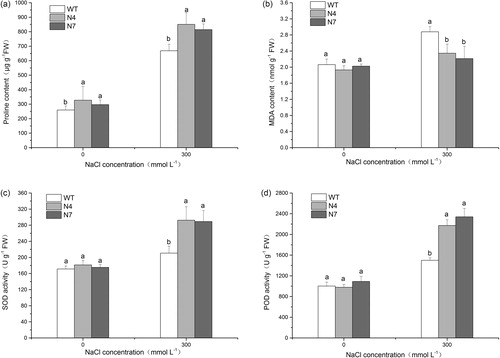
Furthermore, keeping a low cytosolic Na+ concentration and K+ homeostasis is an essential mechanism against salt stress in plants. Previous studies have shown that transgenic plants overexpressing NHX genes accumulate more Na+ than do WT plants under salinity, while the amount of K+ is either unaffected or slightly decreased under salt stress [Citation25,Citation45,Citation46]. In our study, transgenic lines accumulated more Na+, but lost less K+ than WT plants under salt stress, so the Na+ and K+ contents in transgenic lines were higher than in WT plants under salt stress (), which might be attributed to the activity of the vacuolar Na+/H+ antiporter KvNHX1.
Conclusions
In this study, we isolated and characterized a novel vacuolar-type KvNHX1 cDNA from the halophyte Kosteletzkya virginicas and developed transgenic tobacco plants overexpressing KvNHX1. The preliminary results indicated that overexpression of the KvNHX1 gene significantly enhanced salt tolerance in transgenic tobacco plants, which had better growth, more chlorophyll, higher antioxidant enzyme activities and osmoregulation capability by accumulating more proline, Na+ and K+ in the leaves than WT plants under salt stress. Our results suggested that KvNHX1 may have potential use for improving the salt tolerance of plants via genetic engineering. However, more research will be needed to focus on transgenic studies throughout all the developmental stages of plants rather than only the seedling stage and strict evaluation of transgenic plants in field conditions rather than in controlled laboratory or greenhouse conditions.
Disclosure statement
No potential conflict of interest was reported by the authors.
Additional information
Funding
References
- Munns R, Tester M. Mechanisms of salinity tolerance. Annu Rev Plant Biol. 2008;59:651–681.
- Fahad S, Hussain S, Matloob A, et al. Phytohormones and plant responses to salinity stress: a review. Plant Growth Regul. 2015;75:391–404.
- Chen P, Yan K, Shao H, et al. Physiological mechanisms for high salt tolerance in wild soybean (Glycine soja) from Yellow River Delta, China: photosynthesis, osmotic regulation, ion flux and antioxidant capacity. PLoS One. 2013;8:e83227. DOI: 10.1371/journal.pone.0083227
- Wu XH, Zhang HS, Li G, et al. Ameliorative effect of castor bean (Ricinus communis L.) planting on physico-chemical and biological properties of seashore saline soil. Ecol Eng. 2012;38:97–100.
- Li Z, Li G, Qin P. The prediction of ecological potential for developing salt-tolerant oil plants on coastal saline land in Sheyang Saltern, China. Ecol Eng. 2010;36:27–35.
- Shabala S, Cuin TA. Potassium transport and plant salt tolerance. Physiol Plant. 2008;133(4):651–669.
- Zhu JK. Plant salt tolerance. Trends Plant Sci. 2001;6:66–71.
- Niu X, Bressan RA, Hasegawa PM, et al. Ion homeostasis in NaCl stress environments. Plant Physiol. 1995;109:735–742.
- Blumwald E, Aharon GS, Apse MP. Sodium transport in plant cells. Biochim Biophys Acta. 2000;1465:140–151.
- Hasegawa PM, Bressan RA, Zhu JK, et al. Plant cellular and molecular responses to high salinity. Annu Rev Plant Physiol Plant Mol Biol. 2000;51:463–499.
- Apse MP, Aharon GS, Snedden WA, et al. Salt tolerance conferred by overexpression of a vacuolar Na+/H+ antiport in Arabidopsis. Science. 1999;285:1256–1258.
- Shi H, Lee BHWu SJ, Zhu JK. Overexpression of a plasma membrane Na+/H+ antiporter gene improves salt tolerance in Arabidopsis thaliana. Nat Biotechnol. 2003;21:81–85.
- Fukuda A, Nakamura A, Tanaka Y. Molecular cloning and expression of the Na+/H + exchanger gene in Oryza sativa. Biochim Biophys Acta. 1999;1446:149–155.
- Wu CA, Yang GD, Meng QW, et al. The cotton GhNHX1 gene encoding a novel putative tonoplast Na(+)/H(+) antiporter plays an important role in salt stress. Plant Cell Physiol. 2004; 45:600–607.
- Zörb C, Noll A, Karl S, et al. Molecular characterization of Na+/H+ antiporters (ZmNHX) of maize (Zea mays L.) and their expression under salt stress. J Plant Physiol. 2005;162:55–66.
- Cao D, Hou W, Liu W, et al. Overexpression of TaNHX2 enhances salt tolerance of ‘composite’ and whole transgenic soybean plants. Plant Cell Tiss Org. 2011;107(3):541–552.
- Wang J, Zuo K, Wu W, et al. Molecular cloning and characterization of a new Na+/H+ antiporter gene from Brassica napus. DNA Seq. 2003;14:351–358.
- Zhang H, Liu Y, Xu Y, et al. A newly isolated Na+/H+ antiporter gene, DmNHX1, confers salt tolerance when expressed transiently in Nicotiana benthamiana or stably in Arabidopsis thaliana. Plant Cell Tiss Org. 2012;110:189–200.
- Khan MS, Ahmad D, Khan MA. Trends in genetic engineering of plants with (Na+/H+) antiporters for salt stress tolerance. Biotechnol Biotechnol Equip. 2015;29:815–825.
- Brini F, Hanin M, Mezghani I, et al. Overexpression of wheat Na+/H+ antiporter TNHX1 and H+-pyrophosphatase TVP1 improve salt- and drought-stress tolerance in Arabidopsis thaliana plants. J Exp Bot. 2007;58:301–308.
- Li J, Jiang G, Huang P, et al. Overexpression of the Na+/H+ antiporter gene from Suaeda salsa confers cold and salt tolerance to transgenic Arabidopsis thaliana. Plant Cell Tiss Org. 2007;90:41–48.
- Bao-Yan AN, Luo Y, Jia-Rui LI, et al. Expression of a vacuolar Na+/H+ antiporter gene of Alfalfa enhances salinity tolerance in transgenic Arabidopsis. Acta Agron Sinica. 2008;34:557–564.
- Wang J, Zuo K, Wu W, J, et al. Expression of a novel antiporter gene from Brassica napus resulted in enhanced salt tolerance in transgenic tobacco plants. Biol Plantarum. 2004;48:509–515.
- Ohta M, Hayashi Y, Nakashima A, et al. Introduction of a Na+/H+ antiporter gene from Atriplex gmelini confers salt tolerance to rice. FEBS Lett. 2002;532:279–282.
- Xue ZY, Zhi DY, Xue GP, et al. Enhanced salt tolerance of transgenic wheat (Tritivum aestivum L.) expressing a vacuolar Na+/H+ antiporter gene with improved grain yields in saline soils in the field and a reduced level of leaf Na+. Plant Sci. 2004;167:849–859.
- Yin XY, Yang AF, Zhang KW, et al. Production and analysis of transgenic maize with improved salt tolerance by the introduction of AtNHX1 gene. Exp Cell Res. 2004;46:321–326.
- Mishra A, Tanna B. Halophytes: potential resources for salt stress tolerance genes and promoters. Front Plant Sci. 2017;8:829. DOI:10.3389/fpls.2017.00829
- Zhao FY, Zhang XJ, Li PH, et al. Co-expression of the Suaeda salsa SsNHX1 and Arabidopsis AVP1 confer greater salt tolerance to transgenic rice than the single SsNHX1. Mol Breeding. 2006;17:341–353.
- Lan T, Duan Y, Wang B, et al. Molecular cloning and functional characterization of a Na+/H+ antiporter gene from halophyte Spartina anglica. Turk J Agric For. 2014;35:535–543.
- Zhou GS, Xia YR, Ma BL, et al. Culture of seashore mallow under different salinity levels using plastic nutrient-rich matrices and transplantation. Agron J. 2010;102:395–402.
- Tang X, Wang H, Shao C, et al. Global gene expression of Kosteletzkya virginica seedlings responding to salt stress. PLoS One. 2015;10:e0124421. DOI:10.1371/journal.pone.0124421
- Tang X, Wang H, Chu L, et al. KvLEA, a new isolated late embryogenesis abundant protein gene from Kosteletzkya virginica responding to multiabiotic stresses. BioMed Res Int. 2016;2016:9823697. DOI: 10.1155/2016/9823697
- Tang X, Wang H, Shao C, et al. Reference gene selection for qPCR normalization of Kosteletzkya virginica under salt stress. Biomed Res Int. 2015;2015:823806. DOI: 10.1155/2015/823806
- Livak KJ, Schmittgen TD. Analysis of relative gene expression data using real-time quantitative PCR and the 2(-Delta Delta C(T)) Method. Methods. 2001;25:402–408.
- Chen H, Nelson RS, Sherwood JL. Enhanced recovery of transformants of Agrobacterium tumefaciens after freeze-thaw transformation and drug selection. Biotechniques. 1994;16:664–668, 670.
- Burow MD, Chlan CA, Sen P, et al. High-frequency generation of transgenic tobacco plants after modified leaf disk cocultivation with Agrobacterium tumefaciens. Plant Mol Biol Rep. 1990;8:124–139.
- Wang H, Tang X, Wang H, et al. Physiological responses of Kosteletzkya virginica to coastal wetland soil. Sci World J. 2015;2015:354581. DOI: 10.1155/2015/354581
- Yu B, Luo Q, Liu Y. Effects of salt stress on growth and ionic distribution of salt-born Glycine soja. Acta Agron Sinica. 2001;27:203–210.
- Liang M, Lin M, Lin Z, et al. Identification, functional characterization, and expression pattern of a NaCl-inducible vacuolar Na+/H+ antiporter in chicory (Cichorium intybus L.). Plant Growth Regul. 2015;75:605–614.
- Wu C, Gao X, Kong X, et al. Molecular cloning and functional analysis of a Na+/H+ antiporter gene ThNHX1 from a halophytic plant Thellungiella halophila. Plant Mol Biol Rep. 2009;27:1–12.
- Yokoi S, Quintero FJ, Cubero B, et al. Differential expression and function of Arabidopsis thaliana NHX Na+/H+ antiporters in the salt stress response. Plant J Cell Mol Biol. 2010;30:529–539.
- Zhang LQ, Niu YD, Huridu H, et al. Salicornia europaea L. Na+/H+ antiporter gene improves salt tolerance in transgenic alfalfa (Medicago sativa L.). Genet Mol Res. 2014;13:5350–5360.
- Wang B, Zhai H, He S, et al. A vacuolar Na+/H+ antiporter gene, IbNHX2, enhances salt and drought tolerance in transgenic sweetpotato. Sci Hortic. 2016;201:153–166.
- Li N, Wang X, Ma B, et al. Expression of a Na+/H+ antiporter RtNHX1 from a recretohalophyte Reaumuria trigyna improved salt tolerance of transgenic Arabidopsis thaliana. J Plant Physiol. 2017;218:109–120.
- Li W, Zhang Q, Kong X, et al. Salt tolerance is conferred in Arabidopsis by overexpression of the vacuolar Na+/H+ antiporter gene SsNHX2, an alternative splicing variant of SsNHX1, from Suaeda salsa. J Plant Biol. 2009;52:147–153.
- Guan B, Hu Y, Zeng Y, et al. Molecular characterization and functional analysis of a vacuolar Na+/H+ antiporter gene (HcNHX1) from Halostachys caspica. Mol Biol Rep. 2011;38:1889–1899.