Abstract
Caffeic acid phenethyl ester (CAPE), a hydrophobic constituent of poplar propolis of valuable biological activity, was immobilized in poly(ethylene oxide)-b-poly(ε-caprolactone)-b-poly(ethylene oxide) (PEO-b-PCL-b-PEO) copolymer micelles to improve its solubility in water. CAPE was loaded in the micelles by dialysis, achieving ca. 50% encapsulation efficiency. The drug loaded micelles were characterized with a mean diameter of 39 nm, narrow size distribution and slightly positive zeta-potential (approximately 2 mV). The in vitro release profile showed an improved dissolution behavior of micellar CAPE than pure CAPE. In vitro studies on human hepatoma HepG2 and neuronal SH-SY5Y cells demonstrated that the empty PEO-b-PCL-b-PEO micelles were not cytotoxic, whereas the drug loaded micelles exerted cytotoxic effects proportional to CAPE content. The protective activity of pure and micellar CAPE was investigated in a model of H2O2 induced oxidative damage in HepG2 and SH-SY5Y cells. In both cell types, micellar CAPE exhibited better protective activity against the oxidative damage than pure CAPE at very low concentrations (0.1 µg/mL), which is far from the cytotoxic concentration of CAPE-loaded micelles (71 µg/mL). Consequently, the developed micellar formulation has an improved activity against oxidative damage in hepatic and neuronal cells as comparing to pure CAPE.
Introduction
Many plant- or microorganism-derived compounds have shown high potential as therapeutic agents against infections, inflammations or other diseases such as diabetes, cancer, etc. However, the success in clinical trials has been often compromised, partly due to the low bioavailability of these compounds. The low bioavailability requires large quantities of the compounds to be administered in order to achieve the desired therapeutic effects. Nevertheless, the large dose of the compounds could compromise the safety of the product, leading to increased toxicity or low patient compliance.
Discovery of new drug formulations based on natural products possessing biological activity is one of the most intensively studied approaches that could overcome these limitations. The use of nanotechnology in the last three decades has shown immense success in the field of drug delivery [Citation1–5]. The most common types of nanoparticles used for drug delivery are polymer nanoparticles, solid lipid nanoparticles, crystal nanoparticles, liposomes, micelles and dendrimers. The physicochemical properties of drugs determine the type of nanoparticles used in a given delivery application. For instance, the liposomal aqueous compartments can accommodate hydrophilic drugs, whereas a lipophilic drug is better suited for delivery with micelles. Actually, the physical entrapment of lipophilic drugs in polymeric micelles can improve their solubility and bioavailability in in vivo conditions. The compatibility between the solubilizate and the hydrophobic micellar core is one of the key factors for achieving an efficient drug loading and a controlled release profile. A polymer is a good solubilizing agent for a drug when favourable interactions between polymer/drug pairs exist. In addition, the strong interaction between the core-building polymer and the drug slows down the rate of drug release from the carrier.
Caffeic acid phenethyl ester (CAPE) is a natural compound found in a variety of plants and also as a component of propolis from honeybee hives. CAPE possesses broad biological activities, in particular antioxidant, anti-inflammatory, anticancer, etc. [Citation6–11]. CAPE can prevent doxorubicin-induced cardiotoxicity [Citation12], cisplatin-induced nephrotoxicity [Citation13] and focal cerebral ischemia in rats [Citation14]. Most studies suggest that some of the protective effects of CAPE mainly correlate with its antioxidant properties [Citation15–18]. A recent study discussed that CAPE is more effective as an antioxidant than caffeic acid and it inhibited tert-butyl-induced oxidation in HepG2 cells to a higher degree [Citation19]. However, the higher antioxidant activity is related to the higher lipophilicity of CAPE compared to caffeic acid. The latter limits the wide in vivo applications of CAPE, since the low water solubility hinders both the formulation of stable drug dosage form and further absorption of CAPE. Thus, the challenge in that case is to develop a drug delivery system capable of providing solubilization and stability of CAPE, and, on the other hand, of maintaining its pharmacological activity. A study has reported that alcohols should be used with caution as solvents in CAPE formulations, since they can lead to a formation of new bioactive caffeic acid esters in vivo [Citation20]. The use of polymeric nanocarriers could be considered another alternative, which is still not intensively evaluated. Derman [Citation21] employed the single emulsion solvent evaporation method to prepare CAPE-loaded poly(lactide-co-glycolide) (PLGA) nanoparticles of relatively high loading capacity. However, a substantial burst release (32.59–66.72%, depending on the composition) attributed to adsorption of CAPE onto particle surface was observed. Another study reports high anticancer activity of CAPE embedded into poly(ethylene oxide)-b-poly(ε-caprolactone) micelles [Citation22]. These studies demonstrate that the encapsulation of CAPE in polymeric nanocarriers can improve its biopharmaceutical properties and anticancer activity. In our opinion, it is important to explore the impact of nanosized delivery systems on the antioxidant activity of CAPE.
The aim of the present work was to develop CAPE-loaded core-shell PEO-b-PCL-b-PEO micelles as a drug delivery system, aiming to improve the solubility and eventually the antioxidant activity of CAPE. The study was focused on the evaluation of the protective properties of pure and micellar CAPE, which was performed by applying in vitro model of oxidative damage in hepatoma HepG2 and neuronal SH-SY5Y cells. Examination of the biocompatibility of the newly developed micelles on both cell lines was also assessed.
Materials and methods
Materials
Poly(ε-caprolactone) diol (HO-PCL35-OH, CAPA® 2402, molar mass 4000 g.mol−1, kindly donated by Solvay Chemicals) and methoxy poly(ethylene glycol) (PEO113-OH, molar mass 5000 g.mol−1, Fluka) were precipitated in cold methanol (–40 °C), filtered and dried under vacuum at 40 °C overnight. α-Bromoisobutyryl bromide (BIB, Sigma-Aldrich), triethylamine (Et3N, Fluka), 4-pentynoic acid (Acros), copper (I) bromide (CuBr, Sigma-Aldrich), N,N,N',N',N" pentamethyldiethylene-triamine (PMDETA, Sigma-Aldrich), sodium azide (NaN3, Fluka), 4-dimethylaminopyridine (DMAP, Sigma-Aldrich), (3-(4,5-dimethylthiazol-2-yl)-2,5- diphenyltetrazolium bromide) (MTT, Sigma-Aldrich), N-(3-dimethylaminopropyl)-N'-ethylcarbodiimide hydrochloride (EDC, Merck), caffeic acid (Merck), phenethyl alcohol (Merck), p-toluenesulfonic acid (Fluka), methanol (Merck), tetrahydrofuran (Merck), diethyl ether (Merck), 1,4-dioxane (Merck), acetone (Fisher Chemicals), toluene (Merck), silica gel (SiO2, 70–230 mesh, Merck) and activated neutral aluminum oxide (Al2O3, Fluka) were used as received. N,N-dimethylformamide (Sigma-Aldrich) was dried over diphosphorus pentoxide and distilled. Dichloromethane (Sigma-Aldrich) and chloroform (Sigma-Aldrich) were stirred overnight over calcium hydride and subsequently distilled.
Human hepatoma HepG2 and neuroblastoma SH-SY5Y cell lines were obtained from the European Collection of Cell Cultures (ECACC, Salisbury, UK). Both cell cultures were maintained in 75 cm2 flasks at 37 °C in a humidified atmosphere with 5% CO2. For each experiment, cells were plated in 96-well plates. HepG2 were cultured in Dulbecco's modified Eagle medium (DMEM) with 4.5 g/L glucose, supplemented with 10% fetal bovine serum, 2 mmol/L L-glutamine and 1 mmol/L sodium pyruvate; SH-SY5Y cells were cultured in RPMI (Roswell Park Memorial Institute) 1640 medium, supplemented with 10% fetal bovine serum and 2 mmol/L L-glutamine.
Synthesis of PEO-b-PCL-b-PEO block copolymer
Synthesis of alkyne terminated PEO113 macroreagent
Polyethylene glycol monomethyl ether (1.25 g, 0.25 mmol, 1 eq.) was dried by three azeotropic distillations with toluene and dissolved in dry dichloromethane (20 mL) under inert atmosphere in 50 mL round-bottom flask. Pentynoic acid (49.1 mg, 0.5 mmol, 2 eq.) was added at room temperature to the above solution, followed by EDC (95.9 mg, 0.5 mmol, 2 eq.) and DMAP (30.5 mg, 0.25 mmol, 1 eq.). The reaction was left to stir for 24 h, after that was diluted with 60 mL of dichloromethane and extracted with 2 × 75 mL of distilled water. The organic phase was dried over MgSO4, filtered and the solvent was evaporated. The product was redissolved in 10 mL of dichloromethane and precipitated in 100 mL of chilled diethyl ether. Yield 88%. 1H-NMR (CDCl3, δ ppm): 4.27 (t, -CH2OC(O)- group), 3.80–3.50 (m, 450 H, -OCH2CH2O-), 3.36 (s, 3H,CH3O-PEO), 2.57–2.52 (m, -CH2-CH2–C≡CH group), 2.52–2.47 (m, -CH2-CH2–C≡CH group) and 1.98 (t, –C≡CH group).
Synthesis of azide terminated PCL35 macroreagent
PCL35-diol (7.0 g, 1.75 mmol, 1 eq.) was dissolved in approximately 100 mL toluene and about 20 mL of azeotrope solvent was distilled. Triethylamine (NEt3) (0.98 mL, 7.0 mmol, 4 eq.) was added to the 250 mL two-neck round bottom flask at room temperature followed by BIB (1.61 g, 7.0 mmol, 4 eq.) that was added dropwise. The reaction was stirred for 24 h at room temperature and the product was filtered from HBr:NEt3 salt. Next, the solution was concentrated on a rotary evaporator and stirred on activated carbon overnight. The product was precipitated in 300 mL of cold methanol, filtered and finally dried at 40 °C in a vacuum oven. The as-obtained Br-PCL35-Br (0.5 g, 0.125 mmol, 1eq.) and NaN3 (0.162 g, 2.5 mmol, 20 eq.) were then dissolved in 4 mL of anhydrous N,N-dimethylformamide under inert atmosphere in a 50 mL two-neck round bottom flask. The temperature was increased and the reaction was carried out at 50 °C for 48 h. The solution was concentrated under reduced pressure on rotary evaporator, diluted with 50 mL of dichloromethane, washed with 50 mL of water and 2 × 50 mL portions of brine. All aqueous layers were further extracted with 60 mL of dichloromethane and the combined solutions were dried on MgSO4 and evaporated to dryness under reduced pressure. The product was redissolved in 10 mL of dichloromethane and precipitated into 100 mL of cold methanol. The product was further filtrated and washed well with methanol to give PCL carrying clickable azide terminal groups (N3-PCL-N3). Yield 80%. 1H-NMR (CDCl3, δ ppm): 4.2–3.9 (m, -CH2-O-CO-), 2.35–2.15 (m, -CH2-CO(O)-), 1.92 (s, -C-CH3 end groups), 1.7–1.55 (m, -CH2-CH2-O-) and 1.45–1.25 (m, -CH2-CH2-CH2-C(O)-).
Synthesis of PEO113-b-PCL35-b-PEO113 triblock copolymer
N3-PCL35-N3 (0.2 g, 0.05 mmol, 1 eq), PEO113-C≡CH (1.12 g, 0.2 mmol, 4 eq) and CuBr (0.143 g, 10.0 mmol, 20 eq) were added in a flask under nitrogen atmosphere. After three freeze-pump-thaw degassing cycles, anhydrous N,N-dimethylformamide (6 mL) was added via a syringe and the solution was purged with nitrogen and stirred vigorously for 20 min. PMDETA (250.0 µL, 12.0 mmol, 24 eq) was added via a syringe and the solution was degassed by nitrogen for additional 20 min. The reaction mixture was heated up to 30 °C and stirred for 24 h. After addition of 30 mL tetrahydrofuran, the solution was passed through a neutral Al2O3 column to remove the main part of copper salts. The solution was evaporated on a rotary evaporator and the product dissolved in water was finely purified by dialysis in water. Selective removal of the excess of pristine PEO was achieved by ultrafiltration by membrane of cut-off MW= 10 000 g mol−1. Yield =90%. 1H-NMR (CDCl3, δ ppm): 4.25–4.20 (m, -CH2-C H2-O- of PEO), 4.20–4.15 (m, -CH2-C H2-O- of PCL), 4.05 (t, -C H2-O-CO- of PCL), 3.85–3.45 (m, -O-CH2-CH2O- of PEO), 3.37 (s, C H3-O- of PEO), 3.35–3.28 (m, -C-C H2-CH2-C(O) of pent. acid), 3.00–2.90 (m, C-CH2-C H2-C(O) of pent. acid), 2.30 (t, -C H2-CO(O)- of PCL), 1.75–1.55 (m, -C H2-CH2-C(O)- of PCL) and 1.43–1.30 (m, -CH2-C H2-CH2- of PCL).
Synthesis of CAPE
Caffeic acid (1.80 g, 10.0 mmol) and phenylethyl alcohol (17.9 mL, 150 mmol) were dissolved in 100 mL benzene, 100 mg p-toluenesulfonic acid were added and the solution was stirred for four days under reflux in a Dean-Stark apparatus. After that the reaction mixture was separate on a Sephadex column eluted with methanol and CAPE was finally purified by column chromatography on silica gel eluted with petrol ether-acetone (from 5% to 25% acetone).
Preparation and characterization of CAPE-loaded micelles
Empty and CAPE loaded PEO-b-PCL-b-PEO micelles were prepared by the dialysis method. The copolymer (10 mg) and CAPE (2 mg) were dissolved in 4 mL of 1,4-dioxane and the solution was stirred (700 rpm) for 30 min. Micellisation was induced by addition of approximately 2 mL purified water to the organic solution. The resulted micellar dispersion was poured into dialysis membrane (MWCO 6000–8000 g/mol, Spectrum Labs) and dialyzed against water with a refreshment of the external water every 1 hour.
The encapsulation efficiency was determined as a difference between initial concentration of CAPE and its concentration detected in the aqueous fractions collected during the dialysis. CAPE was determined by ultraviolet–visible spectroscopy (UV-Vis) spectrophotometry at λ = 330 nm (Thermo Scientific). The encapsulation efficiency (EE) was calculated using the equation: Encapsulation efficiency = (Total amount of drug – Free drug)/Total amount of drug.
The size, polydispersity index and zeta-potential of empty and CAPE-loaded micelles were evaluated by photon correlation spectroscopy and electrophoretic laser doppler velocimetry (Zetamaster analyser, Malvern Instruments, UK). The samples were measured at 25 °C with a scattering angle of 90°.
Atomic force microscopy (AFM) images were obtained using a Bruker NanoScope V9 Instrument operating at 1.00 Hz scan rate under ambient conditions. Two microlitres of non-filtered micelle solution (0.5 g/L) were placed onto a freshly cleaned glass substrate (1 cm2) and spin-casted at 2000 rpm for a minute. AFM measurements were performed in ScanAsyst (Peak Force Tapping) mode.
In vitro release study
In vitro release of CAPE from the developed micelles was studied in acid and phosphate buffer (pH =1.2 and 7.4, respectively) by dialysis method. Exact volume of the micellar dispersion was introduced into a dialysis membrane bag, which was placed into 100 mL buffer containing 10% ethanol. The release medium was gently stirred (50 rpm) at 37 °C. At predetermined time intervals, samples were withdrawn from the buffer medium and the concentration of the released CAPE was determined as described above.
In vitro cytotoxicity assay
MTT-dye reduction assay
HEP G2 or SH-SY5Y cells were seeded in 96-well microplates at a density 2 × 104 cells/well and allowed to attach to the well surface for 24 h at 37 °C in a humidified atmosphere with 5% CO2. After incubation, different concentrations of blank micelles, pure or micellar CAPE (0.1–71 µg/mL) were added to cells and incubated for a period of 24 h. For each concentration, a set of at least eight wells were used. After the treatment, 10 µL MTT solution (10 mg/mL in PBS) aliquots were added to each well. The microplates were further incubated for 3 h at 37 °C and the obtained formazan crystals were dissolved by the addition of 110 µL 5% formic acid in 2-propanol per well [Citation23]. The absorbance was measured at 560 nm using a Labexim ELISA reader.
Lactate dehydrogenase (LDH) leakage
Stock dispersions of the blank micelles, pure or micellar CAPE (0.1–71 µg/mL) were diluted with growth medium in order to obtain different concentrations. After that, they were added to cells (2 × 104 cells/well) and incubated for periods of 24 h. Eight wells were used for each concentration. LDH leakage from the cells was determined using a commercial LDH cytotoxicity detection kit according to the manufacturer’s protocols (Clontech, USA). LDH activity was assessed in the conditioned media and the detected amounts were calculated as a percentage of the solvent–treated control (GraphPad Prizm Software).
Antioxidant protection assay in a model of H2O2-induced oxidative damage
The model of oxidative damage in HepG2 and SH-SY5Y cells was achieved by hydrogen peroxide (H2O2) treatment of the cells. The cells were seeded in 96-well plates at appropriate density (2 × 104/well for HepG2 cell line and 3 × 104/well for SH-SY5Y cell line) and allowed to attach overnight. Then, they were pre-treated with solutions of pure and micellar CAPE (0.1–71 µg/mL) in culture medium for 1 h. Subsequently, HepG2 cells were subjected to 300 µmol/L H2O2 in PBS for 1 h; SH-SY5Y cells were treated with 1 mmol/L H2O2 in PBS for 15 min. Then the contents of the wells were exchanged with culture medium. After 24 hours, the amount of the attached, viable cells was evaluated by MTT assay. Negative controls (not treated with hydrogen peroxide) were considered to be a measure for 100% protection and positive controls (hydrogen peroxide-treated) for 0% protection.
Statistical analysis
Cell viability tests results are expressed as mean values from at least three independent experiments. The cell survival data were normalized as percentage of the untreated control set as 100% viability and the results were expressed as mean values and standard deviation (±SD). Statistical analysis was performed by one-way analysis of variance (ANOVA) with post hoc multiple comparisons procedure (Dunnet’s test) to assess the statistical differences in case of normal distribution. Values of p < 0.05, p < 0.01 and p < 0.001 were considered as statistically significant.
Results and discussion
Preparation and evaluation of the copolymer carrier
Well-defined PEO-b-PCL-b-PEO triblock copolymer was synthesized by copper mediated ‘click’ coupling reaction of preliminary modified commercial PEO monomethyl ether and PCL-diol [Citation24]. The biodegradable PCL is selected for its hydrophobicity and ability to solubilize poorly water-soluble drugs, while PEO is expected to provide excellent solubility of the system and prolonged circulation in the blood stream by slowing down the recognition and elimination of the nanocarriers by the reticuloendothelial system due to the so-called ‘stealth’ effect [Citation25,Citation26]. In addition, PCL and PEO are approved by the Food and Drug Administration (FDA) for biomedical applications in humans [Citation27].
Alkyne functionalized PEO113-C≡CH macroreagent was synthesized by esterification of PEO113-OH with 4-pentynoic acid. The structure of PEO113-C≡CH macroreagent was confirmed by 1H-NMR spectroscopy (). In addition to the peaks of PEO, the new characteristic signals of alkyne protons at 1.98 ppm and two types of methylene protons at 2.57–2.53 and 2.52–2.47 ppm, respectively, were clearly observed. PCL macroreagent (N3-PCL35-N3) was obtained by a two-step procedure involving esterification and azide end-group functionalisation. Firstly, commercial HO-PCL35-OH was reacted with α-bromoisobutyryl bromide in THF in the presence of TEA. In a second step, the clickable azide end-groups were introduced by nucleophilic substitution of the C–Br bond with NaN3 in DMF at 120 °C for 3 hours. The conversion of the bromide into an azide was confirmed by appearance in the 1H-NMR spectrum () of a signal arising from the methyl groups adjacent to the azide group (δ = 1.92 ppm). The ‘click’ reaction between PEO113-C≡CH and N3-PCL35-N3 macroreagents was carried out in N,N-dimethylformamide in the presence of CuBr/PMDETA catalytic complex at 30 °C for 24 h. An excess of PEO113-C≡CH was used to ensure complete coupling reaction. After purification, the obtained triblock copolymer was characterized by 1H-NMR spectroscopy and gel permeation chromatography (GPC) analysis. Both analyses confirmed that the ‘click’ reaction was complete, since all signals characteristic for PEO and PCL blocks were present in the 1H-NMR spectum (), whereas the GPC curve of the triblock copolymer was monomodal and shifted to a higher molar mass as compared to the two macroreagents (). Thus, a well-defined PEO113-b-PCL35-b-PEO113 triblock copolymer of rather low dispersity (1.3) was synthesized by the azide-alkyne ‘click’ reaction.
Preparation and characterization of CAPE-loaded micelles
CAPE was synthesized by acid catalyzed direct esterification of caffeic acid with phenethyl alcohol in benzene [Citation28]. Toluene-p-sulfonic acid was used as a catalyst. The yield of CAPE was 17.5% and the content of Z-isomer was less than 3% (NMR). Next, CAPE-loaded polymeric micelles were prepared by the dialysis method. The triblock copolymer and CAPE were dissolved in 1,4-dioxane, which is a common solvent for PCL, PEO and CAPE and, then, a portion of water (20 vol.%) was added dropwise to induce the micellisation process. Finally, the organic solvent was replaced by water via dialysis to yield a stable aqueous colloid solution. Based on the intrinsic properties of the two polymers and CAPE in water, one may assume formation of micelles comprising a PCL core, containing CAPE and a hydrophilic PEO shell (, top). The loading efficiency of CAPE under the described procedure reached approximately 50%.
Figure 3. Sketch of the formation of CAPE-loaded PEO-b-PCL-b-PEO micelles (top) and hydrodynamic diameter distribution of empty and CAPE-loaded micelles obtained by DLS (bottom).
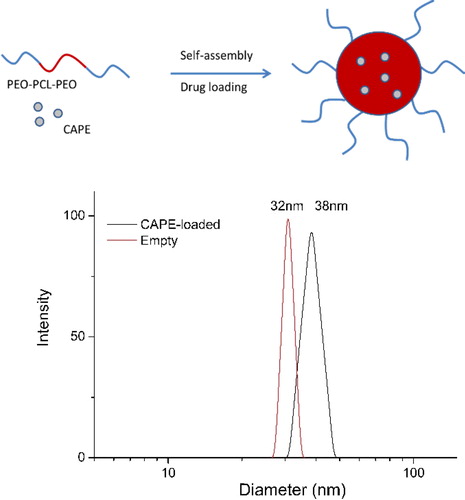
The polymeric micelles were investigated by dynamic light scattering without filtration at a scattering angle of 90° (, bottom). The autocorrelation functions were single-exponential and the size distributions monomodal. The drug-loaded micelles had a mean hydrodynamic diameter of 39 nm, narrow size distribution and slightly positive zeta-potential (approximately 2 mV). The empty micelles exhibited similar characteristics, revealing that the immobilization of CAPE did not trigger notable changes in the micellar system at the experimental conditions reported. Micellar morphology was further visualized by AFM. represents a typical AFM micrograph of the CAPE-loaded PEO-b-PCL-b-PEO micelles deposited on a glass substrate from aqueous dispersion. Generally, spherical objects possessing a diameter of approximately 30 nm were observed. These results were consistent with DLS data and confirmed the formation of nanosized micellar carriers.
Figure 4. Representative 3D AFM height image of CAPE-loaded micelles (A) and cross-section through one of the micelles (B).
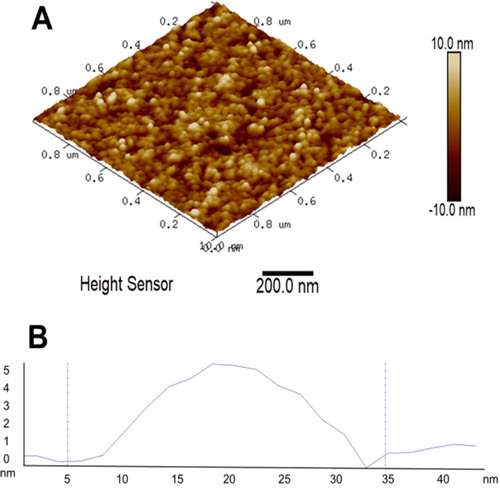
The in vitro release of CAPE was examined in acid and phosphate buffers (pH of 1.2 and 7.4) aiming to consider more options for the route of administration (e.g. oral or parenteral) (). The results showed a weak burst effect and sustained release after that in both media. The burst release of CAPE was comparable with the initial fraction dissolved at incubation of free CAPE under the same conditions (not shown). However, free CAPE was not completely dissolved, whereas micellar CAPE was completely released within 144 hours. This fact suggested that the incorporation of CAPE in the micelles could improve the problem with the low solubility of free CAPE.
Figure 5. In vitro release of CAPE from the developed PEO-b-PCL-b-PEO micelles in acid (pH =1.2) and phosphate buffer (pH =7.4).
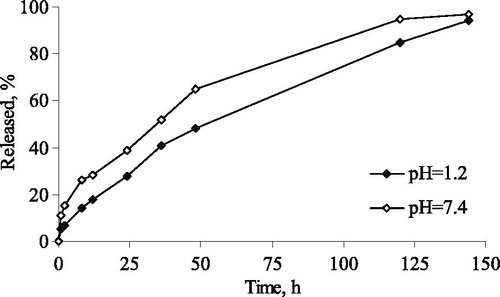
The safety profile of the developed micelles was investigated by evaluation of potential cytotoxic effects on selected cells. Human hepatoma HepG2 and neuronal SH-SY5Y cells were used taking into account that liver and neuronal cells are common target sites of nanoparticle toxicity and oxidative stress-induced injury [Citation29,Citation30]. The possible toxic effects of empty and CAPE-loaded micelles were determined by measurements of cell viability (MTT-assay) and cell membrane integrity (LDH-release) after 24 h continuous exposure. The treatment of HepG2 cells with the empty micelles showed neither decrease in the cell viability, nor impairment in the cell membrane integrity (). The treatment with the CAPE-loaded micelles revealed that with the increase of the concentration of the loaded CAPE, the viability decreased. The cell membrane integrity was also influenced, since an increase in the LDH-leakage was detected at the highest tested concentration (). A similar trend, although less pronounced, was observed with the empty and CAPE-loaded micelles on neuronal SH-SY5Y cells (). Thus, the empty PEO-b-PCL-b-PEO micelles were practically devoid of cytotoxic effects, suggesting their safety profile. The CAPE-loaded micelles demonstrated cytotoxic effects at the highest concentration, which correlated with the data for the cytotoxic activity of CAPE [Citation31,Citation32].
Figure 6. Effects of empty and CAPE-loaded micelles on HepG2 cell viability, assayed by the MTT reduction method (A) and LDH release (B). Note: Data are mean values ± SD. Groups were compared by one-way ANOVA with Dunnet’s post-test, comparing all columns vs. control. (CTRL) ***p < 0.001.
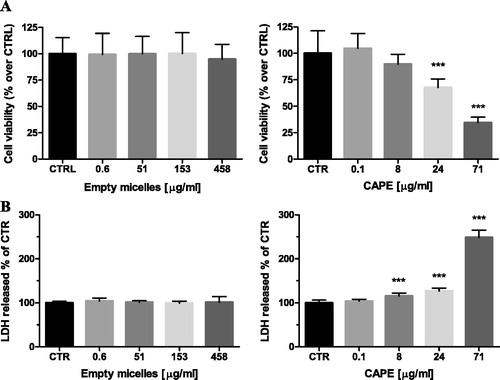
Figure 7. Effects of empty and CAPE-loaded micelles on SH-SY5Y cell viability, assayed by the MTT reduction method (A) and LDH release (B). Results are expressed as mean values ± SD. Note: Data are mean values ± SD. Groups were compared by one-way ANOVA with Dunnet’s post-test, comparing all columns vs. control. (CTRL) ***p < 0.001.
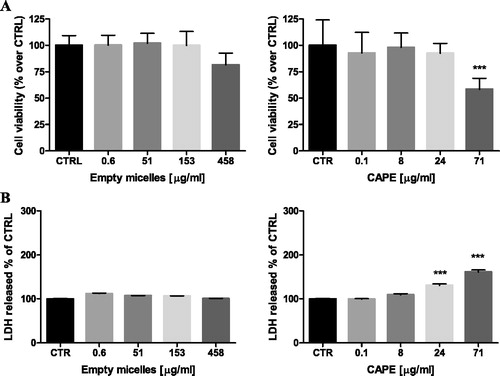
Protective effects of pure and micellar CAPE in a model of H2O2-induced oxidative damage in hepatoma and neuronal cells
The safety profile of the micelles, together with the efficient solubilization of CAPE in the micellar core, allowed us to evaluate the antioxidant potential of the newly developed micellar system. The examination of the antioxidant protection was an important step in the characterization of the micellar formulation, since micellization could affect the activity of the drug. The screening of CAPE-loaded micellar formulation as a protective agent against H2O2-induced oxidative damage was performed on liver HepG2 and neuronal SH-SY5Y cells. H2O2 is commonly used as an inducer of oxidative damage in in vitro models. The mechanism of H2O2-induced cell injury includes production of reactive hydroxyl radicals by Fenton’s reaction which reacts directly with cellular components to damage cellular components (proteins, lipids, DNA, etc.). HepG2 cells were selected for the study, taking into account previous reports for protective activity of CAPE in chemotherapy-induced hepatotoxicity [Citation33–35]. SH-SY5Y cells were chosen for the study because of their well-documented appropriateness in many neuroprotection/neurotoxicity studies [Citation36,Citation37].
HepG2 and SH-SY5Y cells were pre-incubated (1 h) with empty micelles, free and micellar CAPE, and after that treated with H2O2. H2O2-treatment led to a significant cell viability loss in liver and neuronal cells, thus confirming its toxicity (). The pre-incubation with the empty micelles showed no protection on both types of cells, whereas pure and micellar CAPE protected the cells against oxidative damage although to a different degree. The lowest tested concentration of pure CAPE (0.1 µg/mL) was less protective against the oxidative damage in the hepatoma HepG2 cells compared to the micellar CAPE, in particular protection by 20 and 33%, respectively (). In the neuronal SH-SY5Y cells, the lowest CAPE concentration was not protective at all, whereas the micellar CAPE showed protection by 18% (p < 0.01) (). The increase of the concentration of the micellar CAPE (8 and 24 µg/mL) showed similar protection with that exerted by pure CAPE. It could be noted that neuronal SH-SY5Y cells were more sensitive, since the antioxidant effects of pure and micellar CAPE were more pronounced than those on HepG2 cells. For example, the pretreatment of SH-SY5Y cells with micellar CAPE (8 µg/mL) restored viability to 89% vs. H2O2-treatment (p < 0.001). At the same concentration, the viability of HepG2 cells was restored to 52% vs. H2O2-treatment. The incubation with the highest concentration of micellar CAPE (71 µg/mL) showed moderate antioxidant activity in both the neuronal SH-SY5Y (56% increase in cell viability, p < 0.001) and HepG2 cells (35% increase in cell viability, p < 0.05), whereas pure CAPE was not effective.
Figure 8. Protective effects of micellar and pure CAPE in a model of H2O2-induced oxidative damage in HepG2 (A) and SH-SY5Y (B) cells. Note: Data are mean values ± SD. Groups were compared by one-way ANOVA with Dunnet’s post-test, comparing all columns vs. control (CTRL) *p < 0.05; **p < 0.01, ***p < 0.001.
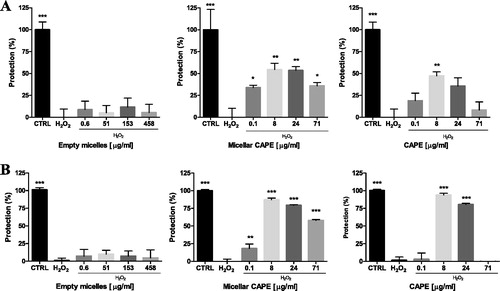
The results of our experiments revealed better antioxidant protection by micellar CAPE vs. pure CAPE in liver HepG2 and neuronal SH-SY5Y cells. The observed antioxidant activity of CAPE in H2O2-induced oxidative damage was consistent with the reported data of other researchers. In an in vitro model of oxidative damage induced by tert-butyl hydroperoxide, Lee et al. [Citation16] reported a dose-dependent reduction in HepG2 cytotoxicity, decreased lipid peroxidation and decreased reactive oxygen species (ROS) levels by CAPE, most probably due to ROS scavenging and DNA protection against oxidative stress-induced damage. A recent work confirmed protective effects of free CAPE (10 and 20 µg/mL) in an in vitro model of tert-butyl hydroperoxide induced cytotoxicity in HepG2 cells [Citation19]. The authors concluded that the cytoprotection may be mediated by activation of nuclear factor erythroid 2-related factor (Nrf2). Taking into account the above reports, the most important observation in the present study was that micellar CAPE protected liver and neuronal cells even at a lower concentration, in particular at 0.1 µg/mL. We speculate that the better protective effects of micellar CAPE might be attributed to the improved solubility of CAPE.
Conclusions
The incorporation of CAPE in PEO-b-PCL-b-PEO micelles could overcome lower solubility of pure CAPE, which that further resulted in the achievement of formulation with high colloid stability suitable for different routes of application. The micellar CAPE demonstrated better protective properties than pure CAPE in a model of oxidative damage in two human cell lines. The results present strong evidence about the promising role of CAPE as an active substance with potential therapeutic activity in pathologies associated with oxidative injury. Thus, the newly developed CAPE-loaded micellar system might be further studied as an effective protective agent against oxidative stress injury in hepatic and neuronal tissues.
Disclosure statement
No potential conflict of interest was reported by the authors.
Funding
This work was supported by the Bulgarian National Science Fund under grant number DN-09/1-2016.
References
- Watkins R, Wu L, Zhang C, et al. Natural product-based nanomedicine: recent advances and issues. Int J Nanomedicine. 2015;10:6055–6074.
- Xin Y, Yin M, Zhao L, et al. Recent progress on nanoparticle-based drug delivery systems for cancer therapy. Cancer Biol Med. 2017;14:228–241.
- Wakaskar RR. Brief overview of nanoparticulate therapy in cancer. J Drug Target. 2018;26:123–126.
- Son GH, Lee BJ, Cho CW. Mechanisms of drug release from advanced drug formulations such as polymeric-based drug-delivery systems and lipid nanoparticles. J Pharmaceut Invest. 2017;47:287–296.
- Tran S, DeGiovanni PJ, Piel B, et al. Cancer nanomedicine: a review of recent success in drug delivery. Clin Transl Med. 2017;6:44–21. [cited 2018 Aug 10][p.] DOI:10.1186/s40169-017-0175-0
- Sud'ina GF, Mirzoeva OK, Pushkareva MA, et al. Caffeic acid phenethyl ester as a lipoxygenase inhibitor with antioxidant properties. FEBS Lett. 1993;329:21–24.
- Chen YJ, Shiao MS, Wang SY. The antioxidant caffeic acid phenethyl ester induces apoptosis associated with selective scavenging of hydrogen peroxide in human leukemic HL-60 cells. Anticancer Drugs. 2001;12:143–149.
- Michaluart P, Masferrer JL, Carothrs AM, et al. Inhibitory effects of caffeic acid phenethyl ester on the activity and expression of cyclooxygenase-2 in human oral epithelial cells and in a rat model of inflammation. Cancer Res. 1999;59:2347–2352.
- Orban Z, Mitsiades N, Burke TRJr, et al. Caffeic acid phenethyl ester induces leukocyte apoptosis, modulates nuclear factor-kappa B and suppresses acute inflammation. Neuroimmunomodulation. 2000;7:99–105.
- Nomura M, Kaji A, Ma W, et al. Suppression of cell transformation and induction of apoptosis by caffeic acid phenethyl ester. Mol Carcinog. 2001;31:83–89.
- Akyol S, Ozturk G, Ginis Z, et al. In vivo and in vitro antineoplastic actions of caffeic acid phenethyl ester (CAPE): Therapeutic perspectives. Nutr Cancer. 2013;65:515–526.
- Fadillioglu E, Oztas E, Erdogan H, et al. Protective effect of caffeic acid phenethyl ester on doxorubicin-induced cardio toxicity in rats. J Appl Toxicol. 2004;24:47–52.
- Ozen S, Akyol O, Iraz M, et al. Role of caffeic acid phenethyl ester, an active component of propolis, against cisplatin-induced nephrotoxicity in rats. J Appl Toxicol. 2004;24:27–35.
- Tsai SK, Lin MJ, Liao PH, et al. Caffeic acid phenethyl ester ameliorates cerebral infarction in rats subjected to focal cerebral ischemia. Life Sci. 2006;78:2758–2762.
- Okutan H, Ozcelik N, Yilmaz HR, et al. Effects of caffeic acid phenethyl ester on lipid peroxidation and antioxidant enzymes in diabetic rat heart. Clin Biochem. 2005;38:191–196.
- Lee KJ, Choi JH, Hwang YP, et al. Protective effect of caffeic acid phenethyl ester on tert-butyl hydroperoxide-induced oxidative hepatotoxicity and DNA damage. Food Chem Toxicol. 2008;46:2445–2450.
- Bhimani RS, Troll W, Grunberger D, et al. Inhibition of oxidative stress in HeLa cells by chemopreventive agents. Cancer Res. 1993;53:4528–4533.
- Wang X, Stavchansky S, Bowman PD, et al. Cytoprotective effect of caffeic acid phenethyl ester (CAPE) and catechol ring-fluorinated CAPE derivatives against menadione-induced oxidative stress in human endothelial cell. Bioorg Med Chem. 2006;14:4879–4887.
- Tsai TH, Yu CH, Chang YP, et al. Protective effect of caffeic acid derivatives on tert-butyl hydroperoxide-induced oxidative hepato-toxicity and mitochondrial dysfunction in HepG2 cells. Molecules. 2017;22:702. DOI:10.3390/molecules22050702
- Celli N, Dragani LK, Murzilli S, et al. In vitro and in vivo stability of caffeic acid phenethyl ester, a bioactive compound of propolis. J Agric Food Chem. 2007;55:3398–3407.
- Derman S. Caffeic acid phenethyl ester loaded PLGA nanoparticles: effect of various process parameters on reaction yield, encapsulation efficiency, and particle size. J Nanomater. 2015;2015:1–12. Article ID 341848. [12 p.] DOI:10.1155/2015/341848
- Lee HY, Jeong YI, Kim EJ, et al. Preparation of caffeic acid phenethyl ester-incorporated nanoparticles and their biological activity. J Pharm Sci. 2015;104:144–154.
- Mosmann T. Rapid colorimetric assay for cellular growth and survival: application to proliferation and cytotoxicity assays. J Immunol Methods. 1983;65:55–63.
- Kolb HC, Finn MG, Sharpless KB. Click chemistry: diverse chemical function from a few good reactions. Angew Chem Int Ed Engl. 2001;40:2004–2021.
- Knop K, Hoogenboom R, Fischer D, et al. Poly(ethylene glycol) in drug delivery: pros and cons as well as potential alternatives. Angew Chem Int Ed Engl. 2010;49:6288–6308.
- Polyethylene glycol (PEG): a versatile polymer for pharmaceutical applications. Exp Opinion Drug Deliv. 2016;13:1257–1275.
- Cai S, Vijayan K, Cheng D, et al. Micelles of different morphologies-advantages of worm-like filomicelles of PEO-PCL in paclitaxel delivery. Pharm Res. 2007;24:2099–2109.
- Zhang P, Tang Y, Li NG, et al. Bioactivity and chemical synthesis of caffeic acid phenethyl ester and its derivatives. Molecules. 2014;19:16458–16476.
- Lenaerts V, Nagelkerke JF, Van Berkel TJC, et al. In vivo uptake of polyisobutylcyanoacrylate nanoparticles by the rat liver Kupffer, endothelial and parenchymal cells. J Pharm Sci. 1984;73:980–982.
- Fawaz F, Bonini F, Guyot M, et al. Influence of poly(DL-lactide) nanocapsules on the biliary clearance and enterohepatic circulation of indomethacin in the rabbit. Pharm Res. 1993;10:750–756.
- Lee YT, Don MJ, Hung PS, et al. Cytotoxicity of phenolic acid phenethyl esters on oral cancer cells. Cancer Lett. 2005;223:19–25.
- Morin P, St-Coeur PD, Doiron JA, et al. Substituted caffeic and ferulic acid phenethyl esters: synthesis, leukotrienes biosynthesis inhibition, and cytotoxic activity. Molecules. 2017;22:1124. DOI:10.3390/molecules22071124
- Iraz M, Ozerol E, Gulec M, et al. Protective effect of caffeic acid phenethyl ester (CAPE) administration on cisplatin induced oxidative damage to liver in rat. Cell Biochem Funct. 2006;24:357–361.
- Yilmaz HR, Sogut S, Ozyurt B, et al. The activities of liver adenosine deaminase, xanthine oxidase, catalase, superoxide dismutase enzymes and the levels of malondialdehyde and nitric oxide after cisplatin toxicity in rats: protective effect of caffeic acid phenethyl ester. Toxicol Ind Health. 2005;21:67–73.
- Albukhari AA, Gashlan HM, El-Beshbishy HA, et al. Caffeic acid phenethyl ester protects against tamoxifen-induced hepatotoxicity in rats. Food Chem Toxicol. 2009;47:1689–1695.
- Jämsä A, Hasslund K, Cowburn RF, et al. The retinoic acid and brain-derived neurotrophic factor differentiated SH-SY5Y cell line as a model for Alzheimer's disease-like tau phosphorylation. Biochem Biophys Res Commun. 2004;319:993–1000.
- Cheung YT, Lau WK, Yu MS, et al. Effects of all-trans-retinoic acid on human SH-SY5Y neuroblastoma as in vitro model in neurotoxicity research. Neurotoxicology. 2009;30:127–135.