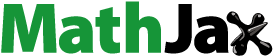
Abstract
The development of microfluidics-based quantitative real-time polymerase chain reaction for molecular diagnosis is becoming a burgeoning field of research. Here, we present a compact microfluidic real-time PCR platform integrated with a thermal cycler and an online fluorescence detection module, including a disposable microfluidic chip fabricated in polydimethylsiloxane. The fluorescence detection module is able to continuously monitor the PCR reaction in the microfluidic chip over thermal cycles, and a large amount of signal data can be rapidly analysed by a built-in computer. In order to further evaluate the performance of the developed PCR platform, we carried out a series of systemic verifications, such as precision of temperature control, sensitivity and specificity. Under the optimal conditions, the limit of detection of the target molecule reached approximately 1.0 × 102 copies/µL, with a correlation coefficient of 0.9966. In addition, hepatitis B virus could be detected quickly by this real-time PCR system in about half an hour. Furthermore, the faint target fluorescence signal emitted from the digital microfluidic chip, which contains thousands of micro-reaction chambers with a volume of 100 pL, could be easily detected on this microfluidic platform. Overall, the results indicated that the developed microfluidic PCR platform can be used for sensitive and specific detection in molecular diagnosis. More importantly, owing to adopting the pattern of modularization design, this platform is considerably compact and portable. If necessary, special custom-made chips could be cooperated with this portable platform to satisfy the needs of various molecular detection tests.
Introduction
Quantitative real-time polymerase chain reaction (qRT-PCR) has shown high-speed development and widespread applications since its invention. It is characterized by specific, sensitive and fast quantitative measurements, and is by far the most enabling technology in testing target nucleic acid copy number [Citation1,Citation2]. The technique first adds fluorescent dyes or fluorescent probes in the PCR reaction system. Then, the PCR reaction process is monitored through the accumulation of fluorescent signal. Finally, a standard curve is drawn for quantitative analysis of an unknown template [Citation3]. As the most advanced nucleic acid molecular diagnostic method in the world, qRT-PCR is widely used in clinical and biological researches. Furthermore, as a gold standard for molecular diagnosis, a strong consensus on its high sensitivity and good repeatability has been reached [Citation4,Citation5]. For example, in the past, hepatitis B virus (HBV), a highly infectious blood-borne agent related to liver, was just screened by the immunological screening technique. However, it was time-consuming and still falling behind in sensitivity. By contrast, the application of nucleic acid amplification testing (NAT) based on fluorescence-based qRT-PCR has improved the viral safety in blood screening [Citation6,Citation7]. Currently, all clinical central laboratories in China have adopted the qRT-PCR technique for HBV detection.
The qRT-PCR instrument has been widely used in the field of life science and clinical medicine, for example, in molecular diagnosis [Citation8], disease control [Citation9] and foodborne pathogen detection [Citation10]. However, the vast majority of commercial devices are in danger of relying too much on the ordinary eight-tube design, which may strongly limit the development of the fluorescence PCR technique [Citation11]. Thus, efforts have recently focused on the miniaturization or automation of the diagnostic equipment. In recent years, the development of microfluidic chip processing techniques based on microelectronics provides a suitable carrier for fluorescence PCR detection [Citation12]. Microfluidics is a new technology which refers to the use of micro-channels on a chip for manipulating microquantities of fluid. It is also known as ‘Lab on a Chip’. Meanwhile, the inherent characteristics of microfluidic chips, such as usability, affordability and portability, can replace the conventional laboratory testing [Citation13,Citation14]. Particularly, when compared to macro-scale testing, it holds great preponderance in molecular diagnosis, such as a lower reagent consumption and shorter analysis time, all of which enable the fluorescence PCR technique to undergo a greater development in future [Citation15,Citation16].
In order to develop a rapid and compact device for molecular diagnosis, we present a novel microfluidic real-time PCR device, which integrates a high-precision fluorescence module to detect the accumulation of fluorescent signal, and is even capable of detecting faint fluorescence signals in a digital microfluidic chip. In addition, the system has a built-in computer for real-time data processing. Using this platform, we successfully amplified 1.0 × 102 copies/µL target gene fragment in less than an hour. In addition, a low copy level virus, such as HBV, could be easily detected in about half an hour. Above all, the developed device is able to distinguish faint fluorescence signals in our digital microfluidic chip, indicating that this detecting platform shows broad application prospects in the field of molecular diagnosis.
Materials and methods
Reagents and procedure
HBV DNA reference material (BDS-BW-010) was purchased from BDS Biological Technology (Guangzhou, China). pUC18 plasmid DNA and PCR test kit (SYBR Premix Ex Taq II, Probe qPCR Mix) were purchased from TaKaRa Bio, Inc. (Dalian, China). PCR primers and the probe were designed against HBV core protein (C) gene (GenBank accession No. KU668337.1) and pUC18 plasmid DNA (GenBank accession No. LC129268.1) by Primer Premier 5.0 and Primer Express 3. The details of the primers and the probe are shown in . All primers and the probe were synthesized by Sangon Bio, Inc. (Shanghai, China).
Table 1. List of primers and probe sequences for amplification of target genes.
The PCR reaction mixtures (20 µL) used to amplify the target fragment of pUC18 plasmid DNA consisted of 2 × SYBR premix Ex Taq II (10 µL), 10 µmol/L forward primer (0.8 µL) and reverse primer (0.8 µL), DNA template (2 µL) and ddH2O (6.4 µL). The PCR mixtures were loaded into an eight-chamber chip and were placed on the thermal cycler of our device (). For the PCR procedure, the setup was as follows, initial denaturation at 95 °C for 30 s; followed by 45 cycles of denaturation at 95 °C for 10 s, annealing at 56 °C for 30 s and extension at 72 °C for 30 s. For the detection procedure, the fluorescence signal was captured at the end of each cycle by a fluorescence detection module. In the end, to identify the specificity of the PCR products using a melting curve analysis, the linear heating programme increased the temperature from 80 °C to 95 °C at increments of 0.1 °C per second, and the fluorescence signal was captured at every 1 °C. DNA samples of different concentrations were tested using this reaction system, and the PCR efficiency was calculated by the slope of standard curves by equation: E = 10(−1/S)−1, here E is the PCR efficiency, S is the slope.
The probe qPCR reagents mixture (20 µL) to detect HBV consisted of 2× probe qPCR mix (10 µL), 10 µmol/L forward primer (0.8 µL) and reverse primer (0.8 µL), DNA template (2 µL), probe (0.5 µL) and ddH2O (5.9 µL) up to the final volume. The thermal cycling programme kept the reaction mixtures at 95 °C for 30 s, followed by 45 cycles of 10 s at 95 °C and 30 s at 60 °C. Images were captured at the end of each cycle, so a total of 45 images were recorded for subsequent analysis.
Microfluidic PCR chip fabrication
The PCR microchip was made in polydimethylsiloxane (PDMS) by rapid prototyping using adhesive transfer tape [Citation17,Citation18]. Sylgard 184 (Dow Corning, USA) was mixed with a matched curing agent in a weight ratio of 10:1, then the mixture was poured into the master mould and heated for 30 min at 120 °C. After cooling, the PDMS replica was removed from the mould, and carved to match the tempered glass substrate (34 × 38 × 0.33 mm3). The chip had eight reaction chambers, and each had two through holes for an inlet and an outlet that were punched out of PDMS using a sharpened needle. The bottom of the chip and a clean tempered glass sheet were treated simultaneously for 50 s using a plasma cleaner (PDC-MG, Ming heng, China) and brought into conformal contact to form an irreversible seal [Citation19]. Finally, the targeted micro-chamber required for PCR in chip was obtained. Furthermore, the digital microfluidic chip (Supplementary Figure S1) was demoulded from another mould which was made by photolithography process, and the other processes were the same as described above.
Apparatus and device construction
The integrated microfluidic real-time PCR device () consists of a fluorescence detection system, a set of thermal cycling control modules and a built-in computer. The dimensions of the apparatus are 320 mm × 275 mm × 450 mm. The internal structure is illustrated in . The light emitting diode (LED) is considered an excellent light source for real-time fluorescence detection due to its extraordinary advantages, such as stable and reliable performance. It only requires low driving power, which will allow the equipment to become portable. Thus, we designed a compact fluorescence detection system with two blue LEDs (475 ± 10 nm, CREE, USA), which can be used for exciting the fluorescent dye. Each LED was equipped with a filter set (excitation wavelength: 470 ± 25 nm; emission wavelength: 525 ± 25 nm, Bd-optic, China), so the target light could pass through the optical filter. Then, the signals are captured by a CCD camera (MC25, Mshot, China) at the end of each cycle. In the end, all of the images or signals are analysed immediately by a built-in computer.
A Peltier-based thermal cycler was assembled consisting of a thermo-electric cooler (TEC; FPH1-12707AC, Fulianjing Electronics, China) module and a heat sink equipped with a water-cooling radiator. In order to achieve spatial thermal homogeneity across the heating surface, a 3-mm thick silver sheet was placed on top of the TEC. A slot was dug in the middle of the silver sheet to embed a Pt1000 sensor (Hayashi Denko, Japan) as the silver sheet was glued to the TEC surface, so the Pt1000 sensor could be used for monitoring the temperature changes and recording real-time data. In addition, the TEC is powered by a switching power supply (CUS350W-24, TDK, Japan) and four relays provide polarity-switching options for heating and cooling using the same TEC module. A microcontroller (STC89C52, HongJing, China) and an analog to digital converter (AD7705, Analog, USA) control the TEC and send data.
Results and discussion
Temperature control
The precise control of temperature at three stages and maintaining it stable at each point are critical for a satisfactory PCR amplification, especially for annealing or denaturation. That is why, the minimum information for publication of quantitative real-time PCR experiments (MIQE) point out that the temperature differences could affect the completion of two processes [Citation20]. Thus, the precision of qPCR results would be directly affected. Generally, the entire temperature control system is controlled by an embedded computer system, and a higher accuracy and lower temperature overshoot are always achieved by proportional integral derivative (PID) control algorithm. Here, in order to improve the PCR control system and achieve accurate control of the thermoelectric cooler, we developed a composite control strategy, that is, Bang-Bang control and feed-forward control with dynamic structural PID control [Citation21,Citation22]. Bang-Bang control played the dominant role when the actual temperature was lower than the control point. On the contrary, if the actual temperature exceeded the temperature control point, feed-forward control will be in dominant status. More importantly, with its strong applicability, the methodology could be adopted in single-station or multiple-station control systems. The mathematical models of these two methods are shown below.
Bang-Bang control
(1)
(1)
where u is the voltage of output; e is the servo error; ε is the switching threshold.
Feed-forward control
(2)
(2)
where Tsetpoint is the setpoint temperature, and Tambient is the ambient temperature; p is the ratio between the applied voltage change and the temperature change; l is the parameter that needs to be optimized.
Variable structural PID control
(3)
(3)
where up, ud and ui are the proportional voltage, differential voltage, and integral voltage of controller output, respectively; e is the servo error; m and n are switching thresholds.
Composite control
(4)
(4)
where uBang-Bang is the voltage of Bang-Bang control; uf is the voltage of feedforward control; uv_PID is the variable structural PID control.
The comparisons of the temperature curves between the chip chamber and TEC during the PCR thermal cycling process are shown in . The two curves followed the same pattern at the points of temperature variation, and there was no overshoot at any turning point. This will contribute to enhancing the amplification efficiency and shortening the reaction time [Citation23]. Particularly, the cooling rate of this platform can reach 3.1 degrees per second, and the heating rate can even reach 5.3 degrees per second, which was superior in the performance compared to traditional tubular PCR devices [Citation24]. For example, Light Cycler 96/480 (Roche, Germany), which is one of the most widely used platforms for molecular detection, has a maximum heating rate and an average cooling rate of 4.4 and 2.2 degrees per second, respectively [Citation25,Citation26]. Therefore, compared with the Light Cycler 96/480 system, the developed platform has a good performance in terms of speed, indicating that it would achieve more rapid detection in molecular diagnosis.
Performance evaluation
In order to evaluate the performance of this real-time PCR platform, we performed several systematic verification assays. Specifically, pUC-18 DNA plasmid was serially diluted to concentrations ranging from 1.0 × 102 to 1.0 × 109 copies/µL to determine the limit of detection. The real-time fluorescence detection results showed typical amplification curves (). As the amplification proceeded, the higher concentration of template corresponded to an earlier rise in the curves, and vice versa. Ultimately, when all the amplification curves reached a plateau, the fluorescence intensity tended to be stable and did not change dramatically in the course of time. The assay was performed three times to confirm the results. As described above, this real-time fluorescence detection system has a fairly broad dynamic detection range, which can span more than eight orders of magnitude, so it would fully satisfy the requirements of molecular detection with different concentrations. In addition, amounts as low as 100 copies/µL DNA could be easily detected within an hour. Thus, this detection limit could apply to the detection of most target samples, particularly for a wide variety of clinical specimens whose gene copy number is ultralow. What is more, the above-stated performance could be achieved in a reaction volume of less than 10 µL, as chambers of arbitrary sizes can be easily designed and fabricated on a microfluidic chip. Notably, this is one of the important factors for the rapid development of microfluidics in recent years [Citation27]. For example, the tubular PCR device in common use (Light Cycler 96/480) can only select programmed volumes, such as 10 µL or 20 µL. However, in cases when a large number of samples are tested, the option to work with smaller volumes would greatly reduce the amount of reagent consumption. We see this as an advantage of the developed platform, suggesting great applicability in molecular detection [Citation28].
Repeatability verification
Next, we performed a series of tests to verify the repeatability of our detection system (). The results showed high repeatability at every concentration of template DNA with a low coefficient of variation (CV). It is noteworthy that the quantification cycle (Cq) values were inversely related to the initial template concentrations, that is, higher initial template DNA concentrations corresponded to lower Cq values. According to the MIQE guidelines, Cq values exceeding 40 are generally considered suspect [Citation20]. In our real-time detection system, all the Cq values were less than 40, and the amplification results showed curve characteristics typical of qRT-PCR, even with the lowest concentration of template DNA, demonstrating that our platform could detect DNA amounts as low as 100 copies/µL. In addition, we used the data presented in to produce a standard curve based on the results from the amplification of different template concentrations (). Generally, an adequate PCR efficiency should be 90–110%, and 100% represents the most optimized efficiency in PCR amplification [Citation20]. In this study, the slope of −3.352 revealed a fairly high PCR efficiency (98.76%) in our real-time detection system, as determined by the equation: E = 10(−1/S) −1, where E is the efficiency and S is the slope [Citation29]. A good linear relationship was observed with the best correlation, represented by an R2 value of 0.9966, thus indicating that good results could be obtained on the microfluidic chip, suggesting that our platform has great potential for gene quantification.
Applicability to HBV detection
In order to demonstrate the capability of our platform for virus detection in real-life biological samples, we added HBV DNA reference material to serum to act as the target template. TaqMan PCR assay was performed in our platform, and the relevant results are shown in . A negative (no-template) control was tested in the same microfluidic chip to guarantee the absence of false-positive results, and there was no amplification signal in this negative control. In contrast, the validation group with HBV DNA showed the typical features of an amplification curve, with great repeatability in three replicate samples. These results showed that our platform demonstrated great performance in detecting HBV DNA in serum within 30 min, thus will greatly shorten the window period of HBV detection, suggesting that the microfluidic chip could satisfy the urgent requirements of real sample detection in molecular diagnosis.
Potential application and future improvements
Currently, the biggest bottleneck of molecular diagnostic equipment lies in poor versatility, which is why different kinds of tests cannot be carried out on specific devices. However, the rise of microfluidic technology has made this pattern change greatly. In this study, a rapid microfluidic platform with the fluorescence detection system was developed, and the experiments demonstrated that the device could be used for real-time fluorescence detection. What is more, its applied value goes even beyond that, as it can also achieve absolute quantification of a gene if using a novel digital microfluidic chip (Supplementary Figure S2). Particularly, for the gene quantification assays carried out in the digital microfluidic chip, no data need to be recorded during the whole process of the tests; only several images are required to be captured by the CCD camera at the end of the test. Then, the information of target images can be ‘read’ and positive points are easily counted using image recognition software [Citation30,Citation31]. In other words, the number of green fluorescent points shown in Supplementary Figure S3 was directly related to the initial template concentration. Ultimately, the gene copy number of the test sample could be calculated by a formula. For absolute gene quantification, the developed device and the attached digital chip have their own unique advantages. On the part of precision, the digital MIQE guidelines point out that the precision of absolute quantification could be directly affected by the number of partitions, and also be increased with an increasing number of partitions [Citation32–34]. Hence, the digital chip (Supplementary Figure S2) with a total of 41,600 individual microcavities was designed (Supplementary Figure S1). Among of them, 33,280 microcavities in four rows were used for absolute quantification of the target gene, and the remaining 8320 microcavities in one independent row were used for a negative control. As far as the partitions are concerned, the designed chip is superior to the digital chip of Thermo Fisher Scientific (20,000 partitions) [Citation33]. On the part of testing costs, the digital chip is one of the main consumables used for assays, and the cost is less than $2 per test if using our chip. This is more cost effective as compared to an estimate of $70 per test in the above-mentioned commercial digital chip. Hence, performing experiments using our chip would be more economical.
Presently, two kinds of chips have been matched with the platform, possibly making it easier to achieve relative or absolute quantification of genes in one device. However, the device needs further developments and improvements, too. For example, the detection throughput of the designed chip is still not high enough. Thus, more studies are needed to enhance the throughput of the chip, so as to satisfy the increasing demands of molecular detection.
Conclusions
In the present study, a rapid microfluidic platform with a real-time fluorescence detection system for molecular diagnosis was developed, tackling several major challenges. At the functional level, the proper LEDs used in the system excited the fluorescence products for a very short period of time in each thermal cycle, which significantly reduced the photobleaching. A unique PID control algorithm achieved precise temperature control. The rapid heating rate of 5.3 °C/s and cooling rate of 3.1 °C/s could significantly shorten the reaction time and enhance the efficiency of PCR amplification. At the system level, the developed detection system integrated a microfluidic PCR chip, real-time fluorescence detection and a data analysis system in a portable stand-alone platform, thus representing a novel PCR detection system with high potential for future use in both research and clinical applications.
Disclosure statement
The authors declare that there is no conflict of interest.
Additional information
Funding
References
- Kubista M, Andrade JM, Bengtsson M, et al. The real-time polymerase chain reaction. Mol Aspects Med. 2006;27(2/3):95–125.
- Ginzinger DG. Gene quantification using real-time quantitative PCR: an emerging technology hits the mainstream. Exp Hematol. 2002;30(6):503–512.
- Giulietti A, Overbergh L, Valckx D, et al. An overview of real-time quantitative PCR: applications to quantify cytokine gene expression. Methods. 2001;25(4):386–401.
- Melchers WJ, Kuijpers J, Sickler J, et al. Lab-in-a-tube: real-time molecular point-of-care diagnostics for influenza A and B using the cobas® Liat® system. J Med Virol. 2017;89(8):1382–1386.
- Felt JR, Yurkovich C, Garshott DM, et al. The utility of real-time quantitative polymerase chain reaction genotype detection in the diagnosis of urinary tract infections in children. Clin Pediatr. 2017;56(10):912–919.
- Lelie N, Bruhn R, Busch M, et al. Detection of different categories of hepatitis B virus (HBV) infection in a multi-regional study comparing the clinical sensitivity of hepatitis B surface antigen and HBV‐DNA testing. Transfusion. 2017;57(1):24–35.
- Blanco S, Balangero MC, Valle MC, et al. Usefulness of nucleic acid testing to reduce risk of hepatitis B virus transfusion-transmitted infection in Argentina: high rate of recent infections. Transfusion. 2017;57(3pt2):816–822.
- Liu W, Zhang M, Liu X, et al. A point-of-need infrared mediated PCR platform with compatible lateral flow strip for HPV detection. Biosens Bioelectron. 2017;96:213–219.
- Fu Z, Zhu Q, Ma Y, et al. Diplotypes of CYP2C9 gene is associated with coronary artery disease in the Xinjiang Han population for women in China. Lipids Health Dis. 2014;13(1):143–151.
- Antonelli A, Arena F, Giani T, et al. Performance of the BD MAX™ instrument with check-direct CPE real-time PCR for the detection of carbapenemase genes from rectal swabs, in a setting with endemic dissemination of carbapenemase-producing Enterobacteriaceae. Diagn Microbiol Infect Dis. 2016;86(1):30–34.
- Lu Z, Zhang JY, Xu LZ, et al. Design and elementary evaluation of a highly-automated fluorescence-based instrument system for on-site detection of food-borne pathogens. Sensors. 2017;17(3):442–460.
- Tan TN, Trinh KTL, Yoon WJ, et al. Integration of a microfluidic polymerase chain reaction device and surface plasmon resonance fiber sensor into an inline all-in-one platform for pathogenic bacteria detection. Sensors Actuat B Chem. 2017;242:1–8.
- Figeys D, Pinto D. Lab-on-a-chip: a revolution in biological and medical sciences. Anal Chem. 2000;72(9):330A–335A.
- Mark D, Haeberle S, Roth G, et al. Microfluidic lab-on-a-chip platforms: requirements, characteristics and applications. Chem Soc Rev. 2010;39(3):1153–1182.
- Sposito A, Hoang V, Devoe DL. Rapid real-time PCR and high resolution melt analysis in a self-filling thermoplastic chip. Lab Chip. 2016;16(18):3524–3531.
- Pin-ChuanChen, Kuan-ChinKuo. High-throughput microfluidic systems for disease detection. J Chin Inst Eng. 2014;37(5):670–675.
- Kim J, Surapaneni R, Gale BK. Rapid prototyping of microfluidic systems using a PDMS/polymer tape composite. Lab Chip. 2009;9(9):1290–1293.
- Xiang Q, Xu B, Fu R, et al. Real time PCR on disposable PDMS chip with a miniaturized thermal cycler. Biomed Microdevices. 2005;7(4):273–279.
- Xiong L, Chen P, Zhou Q. Adhesion promotion between PDMS and glass by oxygen plasma pre-treatment. J Adhes Sci Technol. 2014;28(11):1046–1054.
- Bustin SA, Benes V, Garson JA, et al. The MIQE guidelines: minimum information for publication of quantitative real-time PCR experiments. Clin Chem. 2009;55(4):611–622.
- Visioli A. A new design for a PID plus feedforward controller. J Process Contr. 2004;14(4):457–463.
- Qiu X, Yuan J, Wang Z. Feedforward variable structural proportional-integral-derivative for temperature control of polymerase chain reaction. Chinese J Chem Eng. 2006;14(2):200–206.
- Bu M, Perch-Nielsen IR, Sørensen KS, et al. A temperature control method for shortening thermal cycling time to achieve rapid polymerase chain reaction (PCR) in a disposable polymer microfluidic device. J Micromech Microeng. 2013 [cited 2018 Oct 13];23(7): 074002 [9 p.]. DOI: 10.1088/0960-1317/23/7/074002
- Chen H, Wu Y, Chen Z, et al. Full-automated thermal cycler in nucleic acid testing workstation. J Nanosci Nanotechnol. 2017;17(1):568–572.
- Schmitt M, Wieland U, Kreuter A, et al. C-terminal deletions of Merkel cell polyomavirus large T-antigen, a highly specific surrogate marker for virally induced malignancy. Int J Cancer. 2012;131(12):2863–2868.
- Andrews WJ, Brown ED, Dellett M, et al. Rapid quantification of microRNAs in plasma using a fast real-time PCR system. Biotechniques. 2018;58(5):244–252.
- Riordon J, Sovilj D, Sanner S, et al. Deep learning with microfluidics for biotechnology. Trends Biotechnol. 2018. DOI: 10.1016/j.tibtech.2018.08.005
- Nasseri B, Soleimani N, Rabiee N, et al. Point-of-care microfluidic devices for pathogen detection. Biosens Bioelectron. 2018;117:112–128.
- Kim JH, Kim JH, Wang P, et al. An improved quantitative real-time PCR assay for the enumeration of Heterosigma akashiwo (Raphidophyceae) cysts using a DNA debris removal method and a cyst-based standard curve. Plos One. 2016;11(1): e0145712. [17 p.]. DOI: 10.1371/journal.pone.0145712
- Wu Z, Bai Y, Cheng Z, et al. Absolute quantification of DNA methylation using microfluidic chip-based digital PCR. Biosens Bioelectron. 2017;96(1):339–344.
- Fu Y, Zhou H, Jia C, et al. A microfluidic chip based on surfactant-doped polydimethylsiloxane (PDMS) in a sandwich configuration for low-cost and robust digital PCR. Sensors Actuat B – Chem. 2017;245:414–422.
- Quan PL, Sauzade M, Brouzes E. dPCR: a technology review. Sensors. 2018;18(4):1271–1297.
- Majumdar N, Banerjee S, Pallas M, et al. Poisson plus quantification for digital PCR systems. Sci Rep–UK. 2017;7(1):9617–9626.
- Huggett JF, Foy CA, Vladimir B, et al. The digital MIQE guidelines: minimum information for publication of quantitative digital PCR experiments. Clin Chem. 2013;59(6):892–902.