Abstract
In this study, we investigated environmental oestrogen 17β-estradiol (E2) degradation by a mixed culture of Rhodococcus equi DSSKP-R-001(RS) and Comamonas testosteroni QYY20150409(CT). The results demonstrated that the two strains exhibited synergistic E2 degradation activity, showing that multispecies bacterial systems can improve microbial survival and activity. The E2 degradation rate for the mixed culture system was 94% under conditions in which E2 was the sole source of carbon and growth energy, while the degradation rates of the R. equi DSSKP-R-001 and C. testosteroni QYY20150409 single strains were 86% and 76%, respectively. Comparing and analyzing the degradation products of E2, intermediate cross-metabolism products were observed in the culture containing the two bacteria. The metabolic products of benzene were essentially removed during the process of E2 degradation in the mixed culture, which showed stronger degradation ability than cultures of the individual strains. The results from this investigation on the degradation of E2 by individual and mixed bacterial cultures provide a theoretical basis for the microbial remediation of environmental oestrogens.
Introduction
The common endocrine disruptor 17β-estradiol (E2) is prevalent in many environments, especially wet environments, heavily polluting many areas and causing associated harm, since even at very low concentrations (1.0 ng/L), E2 significantly impacts organisms [Citation1–4]. Oestrogen also affects the sex composition of wild amphibian populations [Citation5]. It has the characteristics of refractory degradation, a wide pollution range, and it can easily undergo bioconcentration in the bodies of humans or other animals. Environmental oestrogen pollutants have become a global environmental problem, therefore necessitating work aimed at exploring suitable and efficient pollution control methods for the treatment of such environmental oestrogen pollution [Citation6,Citation7]. Preventing E2 migration is key to environmental protection and reducing pollution, and according to previous reports, biological degradation is the primary method of preventing E2 migration [Citation8,Citation9].
Most studies on the biodegradation of E2 are focused on the aerobic degradation of E2 by different bacterial species, and few studies have investigated the degradation of E2 in environments with a mixture of bacteria. Shah et al. [Citation10] described the complete degradation of environmental contaminants by the interaction of various microorganisms. Rhodococcus is one of the most important bacterial genera in the actinomycetes order, as they are very important for biodegradation technology. Different strains of Rhodococcus are capable of degrading a wide range of aromatic compounds, hydrocarbon alkyl, steroid and persistent exogenous chemicals [Citation11–15]. In the study by Xu et al. [Citation16], Rhodococcus sp. and Sphingomonas sp. can preferentially convert E2 to Oestrone (E1), and after the formation of 4-OH-E1 the final non-oestrogen product is produced subsequent to the cleavage of the 4-OH-E1 ring. Bergstrand et al. [Citation17] identified a number of genes involved in steroid degradation that are present in actinomycetes. Comamonas testosteroni can completely digest substrates that have steroids as the sole carbon and energy source, owing to its steroid-degrading compounds. Horinouchi et al. [Citation18] described that the 3-ketosteroid 9α-hydroxylase gene (kshA) and 3,4-dihydroxy-9,10-seconandrosta-1,3,5(10)-triene-9,17-dione dioxygenase gene (hsaC) in Mycobacterium and C. testosteroni was involved in the cleavage of the steroid A,B-ring and was a key enzyme in the degradation of microbial steroids.
Natural steroidal oestrogens (also known as C18 steroids) share the same four-ring molecular framework composed of one phenolic group, two cyclohexanes and one cyclopentane ring. The structural differences in C18 lie in the positional configuration of C16 and C17 on the d-ring. For example, oestrogen ketone (E1) possesses a carbonyl group at the C17 position, while 17β-estradiol (E2) possesses a hydroxyl group at the C17 position. The C17 hydroxyl group of E2 can also point downwards or upwards on the molecular plane, forming either α- or β-compounds [Citation7]. No single microorganism can completely degrade all pollutants through metabolic reactions, but a microbial community composed of many different microorganisms can be effectively used for bioremediation [Citation19]. A study showed that a synergistic effect between strains is common among bacteria involved in environmental pollutant degradation and that no individual microorganism can perform all the degradation processes for environmental pollutants [Citation10]. The emergence of the mixed culture model has opened a new door to understanding microorganisms and has had a profound influence on the study of microbial ecology and symbiosis, as well as other fields [Citation20].
To date, some progress has been made using mixed culture methods in the degradation of environmental contaminants. In 2015, Chen et al. [Citation21] jointly cultured Pseudomonas sp. MO2A and Debaryomyces maramus CW36 in liquid medium to degrade polychlorinated biphenyls (PCBs), and the mixed bacterial–yeast culture was more effective at removing PCBs than any single microorganism assayed. Studies by Ding et al. [Citation22] and Shan et al. [Citation23] have shown that mixed-bacterial cultures can increase the degradation rate of crude oil through a synergistic effect between strains. Lade et al. [Citation24] used a mixed fungal–bacterial culture to degrade textile effluent and observed that a combination of microbial species could completely detoxify the dye and effluent, demonstrating potential environmental applications. Chen et al. [Citation25] used a mixed Acinetobacter sp. XM-02 and Pseudomonas sp. XM-01 culture to effectively improve alkane hydrocarbon and degrade crude oil. Thus, compared with single-strain cultures, mixed microbial cultures can improve the degree of mineralization of environmental pollutants and possess significant potential to degrade environmental pollutants more efficiently.
In this study, we investigated the degradation of environmental E2 by a mixture of microbial Rhodococcus equi DSSKP-R-001(RS) and C. testosteroni QYY20150409(CT). The results provide a theoretical basis for the microbial remediation of environmental oestrogen contamination and indicate that bacterial systems are the best choice to degrade environmental pollutants from both an economic and an environmental point of view.
Materials and methods
Chemicals
The E2 standard (99% purity) was purchased from Aladdin. HPLC-grade acetonitrile for liquid chromatography was purchased from Sigma.
Bacterial strains and growth conditions
In this study, R. equi DSSKP-R-001(RS) and C. testosteroni QYY20150409(CT) were deposited at the Institute of Genome Microbiology, Accession No. CGMCC No. 1239 2 and No. 1.15223, respectively. A mixed culture of the above two bacterial strains (H) was grown in Luria-Bertani (LB) medium and inorganic minimal salt medium (MSM) at 30 °C with shaking at 120 r/min.
The LB medium contained 10 g/L tryptone, 5 g/L yeast extract and 10 g/L NaCl, pH 7.4. The MSM medium contained 4.26 g/L NaHPO4, 2.65 g/L KH2PO4, 0.2 g/L MgSO4·7H2O, 1.5 g/L (NH4)2SO4, and 0.02 g/L CaCl2, pH 7. A total of 1 mL of a trace element solution was added to the MSM, which contained 0.024 g/L NiCl2·6H2O, 0.19 g/L CoCl2·6H2O, 0.002 g/L CuCl2·2H2O, 0.061 g/L MnSO4·H2O, 0.024 g/L Na2MoO4·2H2O, 0.07 g/L ZnCl2, and 0.006 g/L H3BO3.
Effect of E2 concentration on E2 degradation by single bacteria
This study used different initial E2 concentrations to assess the effects of different oestrogen concentrations on microbial growth and degradation. The initial concentrations of E2 were 0.5 mg/L and 1 mg/L, and they were added to 100 mL of MSM. In a 250 mL flask, a bacterial suspension of 3% OD600nm = 1 was inoculated and was cultured at 30 °C, 120 r/min, and the remaining amount of E2 in the flasks with the degrading bacteria at different initial concentrations of E2 was measured.
Biodegradation of E2 and analytical methods
For the biodegradation experiment, the laboratory-preserved RS and CT strains were grown in MSM media containing 50 mg/L E2 as the sole carbon source. RS and CT were mixed at a 1:1 ratio and diluted to 3% in MSM media. Cultures were grown at 30 °C on a rotary shaker at 120 r/min for 120 h, with sampling performed at 4, 8, 12, 24, 48, 96 and 120 h. An ELX800 enzyme-labelling instrument was used to measure the concentration of bacterial cells (OD600nm). Three parallel data sets were collected for each sample, and the average concentration was calculated from the three data groups to estimate strain growth.
High performance liquid chromatography
Samples were pre-treated and then analyzed via high-performance liquid chromatography (HPLC) in triplicate. In brief, the samples were placed on glass fibre filter paper, which was then inserted into a conical bottle. Next, 80 mL of methanol was added, and the solution was sonicated in an ultrasonic bath. Sterile syringes were used to remove 1–1.5 mL of the above solution, which was passed through a 0.22-µm organic filter prior to injection into the HPLC column for testing.
In this experiment, E2 concentrations were determined by HPLC. Sample separation was performed using a Zorbax Eclipse Plus C18 column (150 × 4.6 mm, 3.5 mm). The mobile phase consisted of water and acetonitrile at a ratio of 1:1 (v/v), run at 0.8 mL/min in the isocratic elution mode. A total of 10 µL of sample was loaded for each run, with the column temperature set at 40 °C and a detection wavelength of 275 nm. The observed E2 retention time was 10.74 min, as calibrated using standard samples.
Liquid chromatography mass spectrometry
E2 metabolites were analyzed with a PE Sciex API 2000 MDS LC/MS System (Applied Biosystems) after the samples had been separated by HPLC. The electrospray ionization (ESI) method in negative ion mode was used to scan in the 50–450 Da range. According to first-order mass spectrometry, the product is the mother ion, which enters the collision chamber and reacts with the high-purity nitrogen gas. The temperature was set to 0 °C, the collision energy (CE) was set at 35 eV, and the ion mass spectra were obtained by the MS2 analyzer and receiver.
Results and discussion
Growth of the mixed bacterial culture
Environmental steroid contaminants often have complex heteroaromatic or heterocyclic structures, which make them chemically stable and complicate the removal methods. Such compounds are difficult to degrade completely by simple pure microbial cultures. Rhodococcus and C. testosteroni have an impressive ability to degrade steroid substrates and use steroids as the sole source of carbon [Citation17,Citation26]. Both CT and RS grew in MSM with E2 as the sole carbon source, as shown in . The bacterial cell densities in the CT, RS and H cultures gradually increased over time with no observable lag time, indicating that the strains could quickly adapt to the growth medium.
During the first 4 h of culture, the two strains competed for the sole substrate in the media, so the OD600nm value for the mixed culture was lower than that observed for the single-strain cultures. After 4 h, the OD600nm value of the mixed culture was higher than that of the single-strain cultures, indicating that the two-strain mixed culture system promoted the growth of these strains. After the 12 h time point, the bacterial cell densities in the cultures were observed to be H > RS > CT. CT was in the logarithmic growth phase at 12 h, but the OD600nm value was lower for this strain than for RS and H. From 12–48 h, bacterial growth was stable for these three systems. In the first 48 h, the cell density of the RS culture was consistently greater than that of the CT culture. Initially, the growth rate of the RS culture was faster than that of CT, but the OD600nm value of the two strains was almost equal at 96 h. At this time, the OD600nm value of the mixed culture was 0.159, more than either of the single-strain cultures. The number of CT and RS cells remained approximately at the initial 1:1 ratio, demonstrating synergy between the two strains and that their metabolism and growth rates were not slowed. With the phenomenon of mutual promotion, the number and activity of microorganisms in mixed bacterial solution were improved.
Degradation of E2 by mixed bacteria
In order to improve the degradation rates and efficacy for the mixed bacterial culture, the initial E2 concentration used in this study was 50 mg/L. This concentration of E2 did not inhibit the growth of these bacterial strains. Compared to the single culture, the mixed bacterial H was tolerant to this concentration of E2. The initial concentration of 50 mg/L E2 did not inhibit the growth of the strains, and the mixed culture H showed a stronger ability to resist E2 when compared with the pure cultures.
The rate of E2 degradation by the CT, RS and H cultures increased rapidly during the 0–48 h period (). E2 degradation by RS was greater than that observed by CT. The rate of E2 degradation by the mixed bacterial culture H increased linearly. The degradation ability by the mixed bacterial culture always exceeded that of the pure culture strain, and the degradation ability by the single strain in mixed bacterial culture improved. At 48 h, the CT culture exhibited a 65% degradation rate, whereas the E2 degradation rate by the mixed culture was as high as 77%, a notable improvement over that observed for the single-strain cultures. After 12 h, the E2 degradation rate of the mixed culture was consistently greater than that observed for the two single-strain cultures. The E2 degradation rates for all three cultures remained high, reaching values greater than 65%. Thus, the mixed culture model can improve the degradation rate of E2 through the cross metabolism of species, which showed that E2 metabolites could be utilized by the strains. After 48 h, the degradation rate continued to increase in the CT, RS and H cultures, gradually slowing down as the culture time advanced. In the 96 h culture, the E2 degradation rate was stable, demonstrating that the strains could use the E2 metabolites as a source of nutrients.
The E2 degradation rate for the mixed culture system was 94% with E2 as the sole source of carbon and growth energy, while the degradation rates of the RS and CT single strains were 86% and 76%, respectively. The degradation rates for the individual bacterial cultures were lower than that observed for the mixed bacterial culture, indicating that it took longer to degrade E2 by pure cultures.
This study set different initial E2 concentrations to assess the effect of initial concentration on microbial growth and degradation. The results are shown in . When the substrate concentrations were greater than 50 mg/L, the growth rates (based on OD600nm values) of strains RS and CT were lower. When the substrate concentration was less than 5 mg/L, the two strains could completely degrade E2 in the culture solution within 96 h, and use it as a nutrient source to grow and reproduce. When the substrate concentration was 10 and 20 mg/L, the cells of the two strains rapidly multiplied, and the degradation rate of E2 was relatively high. When the substrate concentration was higher than 50 mg/L, the degradation rate of E2 by strain RS and strain CT decreased rapidly, and the OD600nm value levelled out to a plateau. Therefore, the addition of 50 mg/L of E2 was sufficient for the growth of both strains, and E2 greater than 50 mg/L inhibited both strains.
Analysis of the E2 degradation products
The E2 degradation products were separated by HPLC and analyzed by LC–MS/MS. According to the bacterial E2 degradation curve, the degradation rate tended to be stable in the 96 h culture. In addition, the rate of E2 degradation was very low, so the 96 h time point was selected to identify E2 metabolites. The results shown in are the HPLC chromatograms of the E2 metabolites in CT, RS and H culture samples collected at the 96-h time point, respectively. Peak 4 corresponds to the retention time (RT) for the E2 substrate chromatographic peak at 10.74 min; peaks 1–3 are E2 metabolites, while the remaining unmarked peaks are background peaks.
Figure 3. HPLC determination of metabolites produced by the degradation of E2 by CT, RS and H cultures.

For the mass spectrometry analysis of E2 metabolites, we used the negative ion scanning mode to obtain the mass spectra of the culture samples. The mass-to-charge ratio (m/z) corresponds to the molecular weight of the product minus the proton, i.e. [M − H]. Partial HPLC peaks are shown in . The LC–MS/MS analysis did not detect additional metabolites, possibly because they were present at concentrations that were below the limit of detection.
Figure 4. Mass spectrometry analysis of the products of E2 degradation by the CT, RS and H cultures.
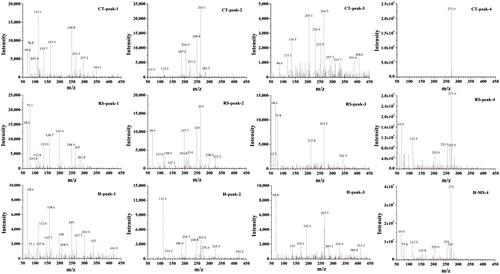
As shown in , a mass spectrometry analysis of the E2 degradation products showed that the degradation of E2 in CT, RS and H cultures resulted in 5, 4 and 7 species of final metabolites, respectively. The m/z values for metabolites in the CT, RS and H culture samples were 117.1, 163.0, 221.2, 253 and 349.1; 158.7, 214, 282.8 and 298.9; and 82.7, 117.0, 158.6, 220.5, 240.8, 283.3 and 300.9, respectively. Two-level mass spectrometry data were analyzed and reference data sets were retrieved from the NIST mass spectrometry online database. The results are shown in . These data allow the products of E2 degradation by CT and RS to be inferred as b, d, f, h, l, c, e, i, and j in in and .
Table 1. E2 degradation products produced by strain CT, strain RS and the mixed bacterial culture H.
Of the seven metabolites in the mixed culture, three ones were unique to the mixed culture and were inferred from their mass spectra to be a, g, and k in .
The metabolites a, g and k produced by E2 degradation in the mixed culture were not detected in the individual CT and RS cultures, as they were produced by cross-metabolism of mixed bacterial strains. Both a and g are organic acids that do not generate secondary pollution. By comparing the E2 degradation products, E2 degradation in the mixed culture was observed to result in the production of metabolites observed from E2 degradation by the single strains.
Furthermore, new metabolites produced from the cross-metabolic intermediate products were observed in the mixed bacterial culture. The final metabolic product essentially resulted in the removal of the metabolite containing the benzene functional group. Thus, E2 degradation is more thorough by degradation of mixed cultures than that observed in the single-strain cultures, and greater degradation ability was exhibited in the mixed culture system than in the single culture systems. Bacterial competition can be avoided by the existence of metabolic diversity among bacteria that degrade natural oestrogen [Citation27], as the strains are competing not only for substrates but also for metabolic intermediates.
Analysis of the mechanisms of E2 degradation by the mixed bacterial culture
In the study conducted by Zhang et al. [Citation28], four modes of E2 degradation were identified in the studied strains. Among these, 29 strains, including Achromobacter xylosoxidans, Rhodococcus sp. EMS-1, could convert E2 into E1, while 30 strains, including strain R. equi Y 50155, Novosphingobium sp. JEM-1, could complete the degradation of E2 or E1. The steps in the degradation processes were as follows: In the first instance, E2 is used as the sole carbon source, and undergoes rapid decomposition and metabolism to produce energy. Second, the strain converts E2 to E1 and further degrades E1. In third instance, the strain can only convert E2 into E1, and cannot complete the further degradation of E1. In the fourth instance, the strain is capable of converting E2 to other products. The mixed strains in this study were able to convert E2 into E1 and to further degrade it into compounds such as organic acids without further polluting the environment or producing additional byproducts that are difficult to degrade [Citation28].
Microbial degradation of pollutants is an enzymatic process, and the type and activity of a given enzyme plays a key role in the process of E2 degradation [Citation29]. Many bacterial dioxygenases can add hydroxyl groups to polycyclic aromatic hydrocarbons, which can undergo open-loop cleavage by key E2 degradation enzymes and enter into the tricarboxylic acid cycle to generate carbon dioxide and water [Citation30,Citation31]. In this study, we investigated the E2 degradation pathway in a mixed culture system and showed that the efficiency of E2 degradation can be improved by using multiple bacterial strains.
In the E2 degradation experiment, we analyzed the E2 metabolites in the mixed culture according to their molecular formulae. The degradation of E2 has been shown to typically start from E1 under anaerobic, anoxic and aerobic conditions [Citation7,Citation27]. In the E2 degradation pathway for the mixed culture, E1 was formed by the hydroxylation of E2 at the c-17 position prior to further degradation of E1. In the Sphingomonas bacterial oestrogen degradation pathway studied by Chen et al. [Citation32], after preferentially degrading E2 into E1, 4-OH-E1 is also produced through OecB hydroxylase activity. The 3,4-dihydroxy-9,10-secoandrosta-1,3,5(10)-triene-9,17-dione (3,4-DHSA) product of the hydroxylation of E1 C9-α appeared in the study conducted by Shtratnikova et al. [Citation33], as well as the analogues of products f, g, c, 9,17-dioxo-1,2,3,4,10,19-hexanorandrostan-5-oic acid (DOHNAA), 9-hydroxy-17-oxo-1,2,3,4,10,19-hexanorandrostan-5-oic acid, and 2-hydroxyhexa-2,4-dienoic acid (2-HHD).
Three different metabolic pathways for the degradation of E1 in the mixed culture were observed, as shown in .
In the A pathway, hydroxylation of E1 occurs at C-4, producing 4-OH-E1 [Citation4]. Further hydroxylation at the C-16 position forms metabolite k, a previously unreported substance that was only observed in the degradation products of the mixed culture. Metabolite k is then further degraded by the tricarboxylic acid cycle.
In the B pathway, 4-OH-E1 is cleaved between C-4 and C-5, leading to its microbial-mediated oxidation and the formation of substances described in Yu et al. [Citation27]. The C-5 position is then oxidized to a carboxyl group through a series of redox reactions, resulting in the formation of intermediate metabolite i [Citation34], a substance that was among the degradation products for the RS strain and the mixed culture. The bond between C-9 and C-10 is cleaved to form the metabolites a and g, which only appeared among the degradation products of H.
In the C pathway, the hydroxylation of E1 at C-9α forms a keto group, where the B ring is cleaved, ring A is hydroxylated at C-4, and the metabolite is cleaved between C-4 and C-5, leading to its microbial-mediated oxidation and the formation of intermediate metabolite l. This substance appeared among the degradation products in the CT culture. C-5 and C-6 are cleaved to form the metabolites b and f, substances that appeared among the degradation products of the CT culture and in the mixed culture. Finally, further degradation occurs to form carbon dioxide and water.
Microorganisms require the participation of more than a dozen enzymes to completely mineralize them into non-oestrogen-active substances [Citation35], making it difficult for a single strain to completely degrade them into non-polluting substances. There is also a concern that such exogenous microorganisms may compete with other native microorganisms, leading to a decline in their viability or degradative abilities. This necessitates coordination among multiple strains to improve their survival and ability to degrade environmental pollutants.
Since many steroid contaminants have similar chemical structures, a variety of microorganisms are selected to form a unified biological system for the degradation of environmental steroid contaminants by mixing these bacteria, so that they can cooperate to more effectively function to degrade steroid contaminants in line with current production practices. However, the rational selection of synergistic degrading bacteria and the degradation mechanisms which they employ need to be further explored. Current bioinformatics technologies coupled with the in-depth study of such degradation mechanisms will allow for a major breakthrough in the research of mixed bacteria culture.
Conclusions
This study investigated the degradation of environmental oestrogen E2 by a mixture of R. equi DSSKP-R-001(RS) and C. testosteroni QYY20150409(CT) and verified that there was synergy in the coexistence of the two strains, which could improve microbial survival and activity for multi-species bacterial systems. The rate of E2 degradation by mixed bacteria was 94% when E2 served as the sole carbon source. Comparative analysis of the degradation products of E2 showed that the intermediate product of cross-metabolism in the two-strain mixture, H, is the degradation product characteristic of a pure culture strain. The metabolic products of benzene were also removed more thoroughly in the multi-strain E2 degradation process, which more effectively degraded E2 than a single bacterial species. Using liquid chromatography–mass spectrometry, three pathways of E2 degradation using a mixed bacterial culture were suggested. This study of the degradation products of a mixed culture system provides a theoretical basis for microbial remediation of environmental oestrogens.
ORCID details
Hongliang Huo 0000-0002-1022-8231
Disclosure statement
No potential conflict of interest was reported by the authors.
Funding
This study was supported by the Natural Science Foundation of China under Grant No. 51478096 and the Natural Science Foundation of Jilin, China under Grant No. 20180101083JC.
References
- Urase T, Kikuta T. Separate estimation of adsorption and degradation of pharmaceutical substances and estrogens in the activated sludge process. Water Res. 2005;39:1289–1300.
- Hinteman T, Schneider C, Schöler HF, et al. Field study using two immunoassays for the determination of estradiol and ethinylestradiol in the aquatic environment. Water Res. 2006;40:2287–2294.
- Gong J, Huang Y, Huang W, et al. Multiphase partitioning and risk assessment of endocrine-disrupting chemicals in the Pearl River, China. Environ Toxicol Chem. 2016;35:2474–2482.
- Yang L, Cheng Q, Lin L, et al. Partitions and vertical profiles of 9 endocrine disrupting chemicals in an estuarine environment: effect of tide, particle size and salinity. Environ Pollut. 2016;211:58–66.
- Lambert MR, Giller GS, Barber LB, et al. Suburbanization, estrogen contamination, and sex ratio in wild amphibian populations. Proc Natl Acad Sci USA. 2015;112:11881–11886.
- Santoro N, Worsley R, Miller KK, et al. Role of estrogens and estrogen-like compounds in female sexual function and dysfunction. J Sex Med. 2016;13:305–316.
- Adeel M, Song X, Wang Y, et al. Environmental impact of estrogens on human, animal and plant life: a critical review. Environ Int. 2017;99:107–119.
- Writer JH, Ryan JN, Keefe SH, et al. Fate of 4-nonylphenol and 17β-estradiol in the Redwood River of Minnesota. Environ Sci Technol. 2012;46:860–868.
- Yu CP, Deeb RA, Chu KH. Microbial degradation of steroidal estrogens. Chemosphere. 2013;91:1225–1235.
- Shah V, Zakrzewski M, Wibberg D, et al. Taxonomic profiling and metagenome analysis of a microbial community from a habitat contaminated with industrial discharges. Microb Ecol. 2013;66:533–550.
- Montersino S, Te PE, Orru R, et al. 3-Hydroxybenzoate 6-hydroxylase from Rhodococcus jostii RHA1 contains a phosphatidylinositol cofactor. Front Microbiol. 2017;8:1110.
- Táncsics A, Benedek T, Szoboszlay S, et al. The detection and phylogenetic analysis of the alkane 1-monooxygenase gene of members of the genus Rhodococcus. Syst. Appl. Microbiol. 2015;38:1–7.
- Kong FX, Sun GD, Liu ZP. Degradation of polycyclic aromatic hydrocarbons in soil mesocosms by microbial/plant bioaugmentation: performance and mechanism. Chemosphere. 2018;198:83–91.
- Wang H, Hu J, Xu K, et al. Biodegradation and chemotaxis of polychlorinated biphenyls, biphenyls, and their metabolites by Rhodococcus spp. Biodegradation. 2018;29:1–10.
- Goswami L, Manikandan NA, Dolman B, et al. Biological treatment of wastewater containing a mixture of polycyclic aromatic hydrocarbons using the oleaginous bacterium Rhodococcus opacus. J Clean Prod. 2018;196:1282–1291.
- Xu J, Zhang L, Hou J, et al. iTRAQ-based quantitative proteomic analysis of the global response to 17β-estradiol in estrogen-degradation strain Pseudomonas putida SJTE-1. Sci Rep. 2017;7:41682.
- Bergstrand LH, Cardenas E, Holert J, et al. Delineation of steroid-degrading microorganisms through comparative genomic analysis. MBio. 2016;7:e00166-16.
- Horinouchi M, Koshino H, Malon M, et al. Steroid degradation in Comamonas testosteroni TA441: identification of metabolites and the genes involved in the reactions necessary before D-ring cleavage. Appl Environ Microbiol. 2018;84:e01324–e01318.
- Shah V, Jain K, Desai C, et al. Metagenomics and integrative ‘-omics’ technologies in microbial bioremediation: current trends and potential applications. In: Marco D, editor. Metagenomics: current innovations and future trends. UK: Caister Academic Press; 2011.
- Nai C, Meyer V. From axenic to mixed cultures: technological advances accelerating a paradigm shift in microbiology. Trends Microbiol. 2018;26:538–554.
- Chen F, Hao S, Qu J, et al. Enhanced biodegradation of polychlorinated biphenyls by defined bacteria-yeast consortium. Ann Microbiol. 2015;65:1847–1854.
- Ding MY, Huang J, Li YQ. The degradation of crude oil by marine microorganisms. Acta Sci Circumst. 2001;41:85–95.
- Shan J, Jia Y, Liu J, et al. Two pseudomonas act on hydrocarbon and their synergistic effect. Microbiology. 2002;29:55–58.
- Lade HS, Waghmode TR, Kadam AA, et al. Enhanced biodegradation and detoxification of disperse azo dye Rubine GFL and textile industry effluent by defined fungal-bacterial consortium. Int Biodeter Biodegr. 2012;72:94–107.
- Chen Y, Li C, Zhou Z, et al. Enhanced biodegradation of alkane hydrocarbons and crude oil by mixed strains and bacterial community analysis. Appl Biochem Biotechnol. 2014;172:3433–3447.
- Mcleod MP, Warren RL, Hsiao WW, et al. The complete genome of Rhodococcus sp. RHA1 provides insights into a catabolic powerhouse. Proc Natl Acad Sci USA. 2006;103:15582–15587.
- Yu Q, Wang P, Liu D, et al. Degradation characteristics and metabolic pathway of 17β-estradiol (E2) by Rhodococcus sp. DS201. Biotechnol Bioprocess Eng. 2016;21:804–813.
- Zhang C, Li Y, Wang C, et al. Occurrence of endocrine disrupting compounds in aqueous environment and their bacterial degradation: a review. Crit Rev Environ Sci Technol. 2016;46:1–59.
- Holert J, Yücel O, Suvekbala V, et al. Evidence of distinct pathways for bacterial degradation of the steroid compound cholate suggests the potential for metabolic interactions by interspecies cross-feeding. Environ Microbiol. 2014;16:1424–1440.
- Cajthaml T, Kresinová Z, Svobodová K, et al. Microbial transformation of synthetic estrogen 17alpha-ethinylestradiol. Environ Pollut. 2009;157:3325–3335.
- Sih CJ, Tai HH, Tsong YY, et al. Mechanisms of steroid oxidation by microorganisms. XIV. Pathway of cholesterol side-chain degradation. Biochemistry. 1968;7:808–818.
- Chen YL, Yu CP, Lee TH, et al. Biochemical mechanisms and catabolic enzymes involved in bacterial estrogen degradation pathways. Cell Chem Biol. 2017;24:712.
- Shtratnikova VY, Schelkunov MI, Fokina VV, et al. Genome-wide bioinformatics analysis of steroid metabolism-associated genes in Nocardioides simplex VKM Ac-2033D. Curr Genet. 2016;62:643–656.
- Crowe AM, Casabon I, Brown KL, et al. Catabolism of the last two steroid rings in Mycobacterium tuberculosis and other bacteria. MBio. 2017;8:e00321–e00317.
- Zheng D, Wang X, Wang P, et al. Genome sequence of Pseudomonas citronellolis SJTE-3, an estrogen- and polycyclic aromatic hydrocarbon-degrading bacterium. Genome Announc. 2016;4:e01373–e01316.