Abstract
Xylanase is widely used in agriculture and food industry. To increase heterologous xylanase expression by Komagataella phaffii under the condition of mixed carbon source feeding, the atg30 gene was knocked out from this yeast to inhibit pexophagy for increasing sorbitol consumption. Komagataella phaffii atg30Δ was constructed through homologous recombination that resulted in the inhibition of pexophagy. When compared with the control, the yield of secretory xylanase increased by 4.1 ± 0.2%, 5.7 ± 0.5%, 11.8 ± 0.4% and 11.4 ± 0.7% under the conditions of 1.5% methanol and 0.5, 1, 1.5 and 2% sorbitol addition, respectively. The residual sorbitol analysis showed that sorbitol consumed by Komagataella phaffii atg30Δ increased by 121.3 ± 9.3%, 66.8 ± 15.2% and 31.2 ± 3.2% following 1, 1.5 and 2 sorbitol addition, respectively. Thus, non-methanol carbon source, co-feeding with methanol, provided more energy without pexophagy by atg30 gene knockout. Pexophagy inhibition increased the heterologous protein expression by Komagataella phaffii, which provided basis for increased yield of xylanase through pexophagy monitoring.
Introduction
Komagataella phaffii (previously known as Pichia pastoris) is a notable heterologous protein producer with advantages such as strong and strictly regulated alcohol oxidase1 promoter (PAOX1), high yield of heterologous protein, and high cell density fermentation. However, low methanol metabolism flux of K. phaffii leads to low energy supply for protein production [Citation1] and high cell death ratio (up to 35%) during high cell density fermentation [Citation2]. Different studies have considered mixed carbon source feeding, such as methanol mixed with glucose, glycerol [Citation3], lactic acid [Citation4], sorbitol [Citation5], etc., as a reasonable strategy to enhance the carbon metabolism of K. phaffii. However, the major issue associated with the mixed carbon source feeding strategy is the inhibition of PAOX1 in the presence of non-methanol carbon source, resulting in pexophagy [Citation6]. Therefore, there is an urgent need to increase the heterologous protein yield of K. phaffii by unlocking the inhibition of PAOX1 in the presence of non-methanol carbon source. Interestingly, sorbitol is used as a non-repressing carbon source to supply energy for heterologous protein expression. However, the specific growth rate and final biomass of K. phaffii in the presence of sorbitol as the carbon source have been reported to be lower than those achieved in the presence of glycerol [Citation7]. To increase sorbitol metabolism and heterologous protein production, atg30 knockout recombinant K. phaffii was constructed to inhibit pexophagy because protein Atg30, encoded by atg30, is an important component of pexophagy [Citation8]. In our previous study, xylanase, an important food-grade enzyme used in food industry, from Aspergillus niger ATCC 1015 was expressed by recombinant K. phaffii [Citation9]. Hence, in the present study, the yield of xylanase was enhanced for its wide applications.
Materials and methods
Strains, vectors and media
A. niger ATCC 1015 was purchased from American Type Culture Collection (Manassas, VA, USA). Escherichia coli Top10 competent cells were obtained from Takara Biotechnology Co. Ltd (Dalian, China). K. phaffii GS115, pPIC9K and zeocin were bought from Life Technologies (Carlsbad, CA, USA). All the restriction enzymes, DNA markers and biological kits were purchased from Takara Biotechnology Co., Ltd (Dalian, China). All the primers in this study () were synthesized by Sangon Biotech Inc (Shanghai, China), and all the chemicals were purchased from Sino Chemical Co. (Shanghai, China).
Table 1. Primers (Restriction endonuclease sites were marked by bold-underline).
YPD medium contained yeast extract, 10 g/L; peptone, 20 g/L; glucose, 20 g/L and agar powder (if necessary), 15 g/L. BMGY medium consisted of yeast extract, 10 g/L; peptone, 20 g/L; PBS buffer (pH 7.0), 100 mmol/L; glycerol, 20 g/L; YNB, 13.4 g/L and biotin 4Ч10−4 g/L. BMMY medium contained yeast extract, 10 g/L; peptone, 20 g/L; PBS (pH 7.0), 100 mmol/L; methanol, 5 mL/L; (NH4)2SO4, 20 g/L and biotin, 4Ч10−4 g/L. MD medium consisted of YNB, 13.4 g/L; biotin, 4Ч10−4 g/L; glucose, 10 g/L and ethanol, 10 mL/L. MM medium comprised (per L) YNB, 13.4 g; biotin, 4Ч10−4 g/L and methanol, 5 mL//L.
Construction of K. phaffii atg30Δ, complement strain and xylanase expression strain (K. phaffii xynB/atg30Δ)
The general molecular operations were conducted according to Molecular Cloning [Citation10]. Molecular manoeuvre in K. phaffii was performed according to EasySelect Pichia Expression Kit Manual (Thermo fisher Scientific). The atg30 upstream fragment with 5’ BglII site and 3’ XhoI was amplified by polymerase chain reaction (PCR) using primers up-atg30-F and up-atg30-R. Furthermore, primers down-atg30-F and down-atg30-R were used for PCR amplification of the atg30 downstream fragment with 3’ BglII site according to the genome sequence of K. phaffii GS115. Then, the upstream and downstream fragments were used as templates, and down-atg30-F and up-atg30-R were employed as primers to obtain homologous arm with a BglII site between atg30 upstream and downstream fragment. After adding PolyA by using rTaq enzyme, the homologous arm was ligated with vector pMD19-T and transformed into E. coli Top10 cells. The recombinant vector was digested using XhoI and ligated with XhoI-digested Kanamycin-HIS4 fragment obtained from pPIC9K using kan-his-F and kan-his-R as primers, and transformed into E. coli Top10 cells to obtain a recombinant vector pMD-atg30Δ. The primers in-atg30-F and in-atg30-R were used for the verification of atg30 of pMD-atg30Δ. After linearisation by BglII digestion, the recombinant vector pMD-atg30Δ was transformed into K. phaffii and K. phaffii xynB competent cells to obtain K. phaffii atg30Δ and K. phaffii xynB/atg30Δ strain, which were screened from HIS- plate and 200 µg/mL G418 MD plate, respectively.
For complement strain construction, the atg30 gene was obtained from the K. phaffii genome using atg30-F and atg30-R as primers. The obtained atg30 gene fragment was fused with pPICZA after BglII and XhoI digestion to produce recombinant pPICZA-atg30. The pPICZA-atg30 vector, after linearisation by DraI digestion, was transformed into K. phaffii atg30Δ competent cells to obtain the complement strain, which was verified by atg30 amplification using in-atg30-F and in-atg30-R as primers, and sequencing. The cloning and expression of xylanase gene (xynB) from A. niger ATCC 1015 has been described in our previous study [Citation9].
Cultivation of K. phaffii strains for pexophagy identification
The recombinant K. phaffii strains were cultivated in 50-mL tubes containing 5 mL of YPD medium at 30 °C and 200 rpm for 8–24 h. Then, the YPD supernatant was discarded by centrifugation (8437 g) to obtain the cell pellet. The cell pellet was transferred into MM medium and incubated for another 24 h for induction. Then, the cell pellet was transferred into a 250-mL flask containing 50 mL of MD medium (containing glucose for micropexophagy, containing ethanol for macrpexophagy) for cultivation at 30 °C and 200 rpm. Alcohol oxidase (AOX) and formate dehydrogenase (FDH) activities were determined at 2.0, 4.0 and 6.0 h cultivation.
To obtain the growth curves of the recombinant K. phaffii strains, the strains were cultivated in 50-mL tubes containing 5 mL of YPD medium at 30 °C and 200 rpm for 8–24 h. Subsequently, the culture broths were transferred into a 250-mL flasks containing 100 mL of YPD medium and incubated at 30 °C and 200 rpm for 48 h.
Cultivation optimization for xylanase expression by recombinant K. phaffii strains
The recombinant K. phaffii strains were cultivated in 50 mL of YPD medium at 30 °C and 200 rpm for 18–24 h to an optical density at 600 nm (OD600) of 2.0–8.0, and then transferred into 250-mL flasks containing 100 mL of BMGY medium at 4% inoculation ratio and incubated at 30 °C and 200 rpm for 24 h. The recombinant K. phaffii cells were harvested by centrifugation (8437 g, 5 min) and resuspended in 100 mL of BMMY medium in 250-mL flasks with different methanol concentrations. The default (control) cultivation conditions were pH of 5.0, 30 °C and 4% inoculation ratio.
For cultivation with sorbitol addition, the recombinant K. phaffii strains were cultured in 50 mL of YPD medium at 30 °C and 200 rpm for 18–24 h to an OD600 of 2.0–8.0, and then transferred into 100 mL of BMGY medium at 4% inoculation ratio and incubated at 30 °C and 200 rpm for 24 h. The recombinant K. phaffii cells were harvested by centrifugation (8437 g, 5 min) and suspended in 100 mL BMMY medium with 1.5% methanol for 24 h. Subsequently, 0.5, 1, 1.5 and 2% sorbitol was respectively added and 1.5% methanol was added every 24 h. The default (control) cultivation conditions were pH of 5.0, 30 °C and 4% inoculation ratio.
Analysis
The cell density of K. phaffii cells was measured using a spectrophotometer (U-1100, Hitachi Ltd., Tokyo, Japan) against distilled water at 600 nm after appropriate dilution. Determination of alcohol oxidase (AOX) and formate dehydrogenase (FDH) activities, ATP, ADP and AMP concentrations, and energy charge (EC) was conducted according to our previous study [Citation11]. Xylanase B activity was ascertained as described in our previous work [Citation9], and sorbitol determination process has been presented in an earlier study [Citation12].
Results and discussion
Construction of K. phaffii atg30Δ, complement strain and K. phaffii xynB/atg30Δ
The entire process of atg30 gene knockout and complementation is shown in Supplementary material, Figure S1. PCR amplification generated fragments 600 and 650 bp for upstream and downstream fragments, respectively (Supplementary material, Figure S1(A)). These two fragments were ligated into a 1250-bp fragment by overlap PCR (Supplementary material, Figure S1(B)). After polyA generation using rTaq enzyme, this 1250-bp fragment was ligated with vector pMD19-T to obtain pMD19-atg30Δ-DU. A 4110-bp Kanamycin-HIS4 fragment (Supplementary material, Figure S1(C)) was generated by PCR from vector pPIC9K. Both pMD19-atg30Δ-UD and the Kanamycin-HIS4 fragment were ligated after XhoI digestion to obtain pMD19-atg30Δ, which was confirmed by XhoI digestion and double enzymes (BglII and XhoI) digestion, resulting in a band of about 4000 bp and two bands of 7400 and 600 bp, respectively (Supplementary material, Figure S1(D)). After linearisation using BglII, pMD19-atg30Δ was transformed into K. phaffii GS115 and K. phaffii xynB to generate recombinant K. phaffii atg30Δ and K. phaffii xynB/atg30Δ, respectively, which were screened using MD plates with G418. Subsequently, the recombinant K. phaffii atg30Δ and K. phaffii xynB/atg30Δ were also confirmed by atg30 gene amplification with the K. phaffii GS115 genome as control (Supplementary material, Figure S1(E,F)).
Furthermore, the atg30 gene was complemented into K. phaffii atg30Δ to confirm atg30 gene knockout of K. phaffii atg30Δ. Briefly, the complete atg30 gene (2380 bp) was amplified from the K. phaffii GS115 genome (Supplementary material, Figure S1(G)) and ligated with vector pPICZA after digestion by BglII and XhoI. Subsequently, after linearisation by DraI, pPICZA-atg30 was transformed into K. phaffii atg30Δ, and screened using MD plate with 100 µg/mL zeocin. The genome of complement K. phaffii strain was used as the template to amplify part of atg30 (350 bp) (Supplementary material, Figure S1(H)). The results confirmed that the atg30 gene was knocked out successfully.
Characterisation of K. phaffii atg30Δ and K. phaffii atg30Δ complement
The growth curves of these K. phaffii strains were similar to that of K. phaffii GS115 (), suggesting that atg30 knockout did not significantly influence K. phaffii growth.
illustrates the AOX and FDH activities during the two pexophagy conditions (micropexophagy and macropexophagy). In the K. phaffii atg30Δ complement, the AOX activity significantly decreased during both the pexophagy conditions (), whereas the FDH activity significantly decreased only during micropexophagy (). These changes in the enzyme activities were similar to those of K. phaffii GS115 during pexophagy [Citation13], confirming that the atg30 gene was successfully complemented into the K. phaffii atg30Δ genome. In K. phaffii atg30Δ, the AOX activity did not decrease significantly under both the conditions of pexophagy (), indicating that the atg30 gene knockout inhibited pexophagy in the presence of non-methanol carbon source.
Effect of methanol addition on xylanase expression by K. phaffii strains
The effect of methanol addition on the cell density of K. phaffii xynB and K. phaffii xynB/atg30Δ was similar (). The cell densities of the strains increased with the increasing of induction time. After 96 h of induction, the cell densities of K. phaffii xynB increased from 13.0 ± 0.3, 13.0 ± 0.3, 13.3 ± 0.3 and 13.5 ± 0.3 OD600 to 20.2 ± 0.8, 20.9 ± 1.3, 19.2 ± 0.1 and 18.9 ± 0.6 OD600 at the conditions of 0.5, 1.0, 1.5 and 2.0% methanol addition, respectively. The cell densities of K. phaffii xynB/atg30Δ increased from 13.8 ± 0.6, 13.7 ± 0.5, 14.0 ± 0.4 and 14.6 ± 0.6 OD600 to 21.1 ± 0.5, 21.8 ± 0.4, 22.6 ± 0.5 and 21.2 ± 0.3 OD600 at the conditions of 0.5, 1.0, 1.5 and 2.0% methanol addition, respectively, after 96 h of induction. In both the K. phaffii strains, the xylanase activities continuously increased with time till 48 h of induction. Subsequently, the xylanase activities of K. phaffii-xynB remained stable at 48–96 h of induction, whereas those of K. phaffii xynB/atg30Δ continued to increase till 72 h of induction. The xylanase activities of K. phaffii xynB remained stable, even in the presence of 1.5% and 2.0% methanol. It must be noted that the xylanase activities in both the K. phaffii strains increased with increasing methanol concentration of up to 1.5% methanol, and did not increase further under the condition of 2% methanol addition. The highest xylanase activities achieved in K. phaffii xynB and K. phaffii xynB/atg30Δ were 864.4 ± 36.1 and 956.1 ± 34.7 U/mL at 1.5% methanol, respectively ().
Effect of sorbitol addition with 1.5% methanol on xylanase expression by K. phaffii strains
The effect of sorbitol addition on the cell density of the two K. phaffii strains was similar as shown in . The cell densities of K. phaffii xynB increased from 14.1 ± 0.4, 15.0 ± 0.2, 15.3 ± 0.6 and 15.1 ± 0.7 OD600 to 22.6 ± 0.5, 23.5 ± 0.4, 23.5 ± 0.4 and 24.0 ± 0.5 OD600 at the conditions of 0.5, 1.0, 1.5 and 2.0% sorbitol addition, respectively, after 96 h of induction. The cell densities of K. phaffii xynB/atg30Δ increased from 15.0 ± 0.2, 14.9 ± 0.4, 15.1 ± 0.2 and 14.7 ± 0.2 OD600 to 22.9 ± 0.2, 22.4 ± 0.6, 23.2 ± 0.4 and 22.8 ± 0.2 OD600 at the conditions of 0.5, 1.0, 1.5 and 2.0% sorbitol addition, respectively, after 96 h of induction. Furthermore, the cell densities of both the K. phaffii strains grown in the presence of different concentrations of sorbitol showed similar curves. The xylanase activities in the two K. phaffii strains increased with time following the addition of different concentrations of sorbitol (), and, in particular, addition of 0.5% and 1% sorbitol led to higher xylanase activities. The final xylanase activities of K. phaffii xynB/atg30Δ after 96 h of 0.5, 1, 1.5 and 2% sorbitol addition were 1365.3 ± 55.2, 1340.6 ± 79.5, 1115.2 ± 11.9 and 1140.7 ± 40.9 U/mL, which increased by 4.1 ± 0.2%, 5.7 ± 0.5%, 11.8 ± 0.4% and 11.4 ± 0.7%, respectively, with the highest activity of xylanase (1365 U/ml ± 55.2) achieved following 0.5% sorbitol and 0.5% methanol addition.
Figure 4. Effect of sorbitol and 0.5% methanol addition on cell density and xylanase activity of recombinant K. phaffii strains. Cell density (A) and xylanase activity (B) of K. phaffii xynB; Cell density (C) and xylanase activity (D) of K. phaffii xynB/atg30Δ.
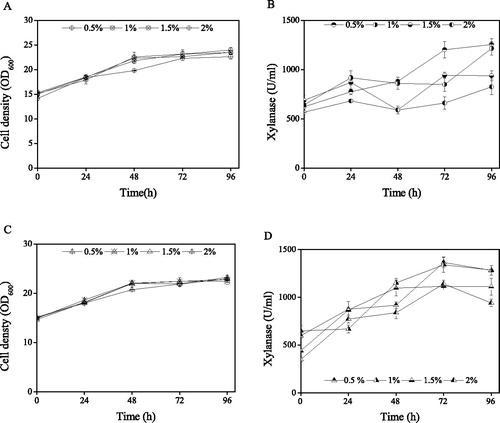
As an indicator of pexophagy, EC remained at a relatively constant value of 0.5, demonstrating non-pexophagy conditions with sorbitol addition (). The residual sorbitol content in the two recombinant K. phaffii cultures showed that more sorbitol was consumed by K. phaffii xynB/atg30Δ, which provided more energy for protein expression. Furthermore, whereas the residual sorbitol level in the two recombinant K. phaffii cultures was similar under the condition of 0.5% sorbitol addition, it was lower in the K. phaffii xynB/atg30Δ culture under the conditions of 1, 1.5 and 2% sorbitol addition, when compared with that in the K. phaffii xynB culture. After 96 h induction, the final sorbitol concentrations in the cultures of K. phaffii xynB and K. phaffii xynB/atg30Δ were 1.8 ± 0.1, 3.3 ± 0.2, 4.1 ± 0.1, 4.5 ± 0.1%, and 1.9 ± 0.2, 2.7 ± 0.1, 2.8 ± 0.1, 3.4 ± 0.1%. The amount of sorbitol consumed by K. phaffii xynB/atg30Δ increased by 121.3 ± 9.3%, 66.8 ± 15.2% and 31.2 ± 3.2% under the condition of 1, 1.5, and 2 sorbitol addition, respectively ().
Prospects
Research on the application of K. phaffii for heterologous proteins and metabolites production has attracted numerous researchers and industrial partners [Citation14,Citation15]. However, a major limitation of heterologous protein production by K. phaffii is the dissolved oxygen content, with low dissolved oxygen content causing methanol toxicity to K. phaffii cells, and high dissolved oxygen content resulting in high cell death ratio owing to low methanol concentration [Citation16]. Although mixed carbon source feeding has been reported to increase the carbon metabolism flux and energy generation, it inhibits PAOX1, resulting in pexophagy [Citation6], which decreases heterologous protein production [Citation17]. Atg30 has been identified as an initial acceptor of the pexophagy process [Citation8]. Therefore, we knocked out the atg30 gene to interrupt pexophagy (), causing retention of peroxisomes in K. phaffii cells under the condition of non-methanol carbon source addition, which demonstrated the significance of PAOX1 () although the relationship between pexophagy and PAOX1 inhibition is not clear. Furthermore, under the condition of 1, 1.5 and 2% sorbitol addition as a non-methanol carbon source following atg30 gene knockout in K. phaffii cells, the consumption of sorbitol increased by 89.7, 66.4 and 31.1%, respectively, revealing that carbon metabolism increased with pexophagy inhibition. As a non-repressing carbon source, sorbitol has been often employed for K. phaffii cultivation in previous studies [Citation18], with higher sorbitol consumption resulting in increased heterologous protein production [Citation19]. Accordingly, in the present study, atg30 gene knockout in K. phaffii cells further increased the sorbitol consumption resulting in higher xylanase yield.
Xylanase is widely used in food, animal feed, brewing, paper and pulp industry, and components extraction from biomass, because of its breaking of xylan [Citation20]. Therefore, xylanase is highly demanded to utilize all kinds of biomass as a renewable source. Recently, several kinds of xylanases were discovered to endeavour a range of diverse applications [Citation21]. Heterologous expression of xylanase is a hot topic for researchers and industrial engineers [Citation9,Citation22]. One important property of xylanase for its further application is its safety. Aspergillus niger ATCC1015 is a safe fungal species of GRAS state approved by the United States’ Food and Drug Administration (FDA) [Citation23]. High-level expression of Aspergillus niger ATCC 1015 xylanase is a benefit for its application, even in food industry. K. phaffii is advantageous for its high cell density fermentation and high level of heterologous protein expression. In this study, the xylanase yield was increased by 4.1 ± 0.2%, 5.7 ± 0.5%, 11.8 ± 0.4% and 11.4 ± 0.7%, through 0.5, 1.0, 1.5 and 2.0% sorbitol addition. These results showed a promising further high-level xylanase production at the condition of K. phaffii high cell density fermentation.
Conclusions
The sorbitol consumption of recombinant K. phaffii was increased by atg30 gene knockout to enhance xylanase expression as heterologous protein. This result was attributed to pexophagy inhibition of K. phaffii at the condition of supplemental carbon source addition. Our results showed the potential of heterologous protein biosynthesis by recombinant K. phaffii, and further application of xylanase.
Funding
This work was supported by the National Natural Science Foundation of China (Nos. 31870045 and 21306112) and the State Key Laboratory of Pollution Control and Resource Reuse Foundation (PCRRF16001).
Supplemental Material
Download PDF (766.6 KB)Disclosure statement
No potential conflict of interest was reported by the authors.
References
- Heyland J, Fu J, Blank LM, et al. Carbon metabolism limits recombinant protein production in Pichia pastoris. Biotechnol Bioeng. 2011;108:1942–1953.
- Hohenblum H, Borth N, Mattanovich D. Assessing viability and cell-associated product of recombinant protein producing Pichia pastoris with flow cytometry. J Biotechnol. 2003;102:281–290.
- Jorda J, Jouhten P, Camara E, et al. Metabolic flux profiling of recombinant protein secreting Pichia pastoris growing on glucose: methanol mixtures. Microb Cell Fact. 2012;8:11.
- Xie J, Yang R, Zhou Q, et al. Efficiencies of growth and angiostatin expression in cultures of Pichia pastoris fed with mixed carbon sources. Chem Biochem Eng Q. 2013;27:235–244.
- Jia L, Mpofu E, Tu T, et al. Transcriptional analysis for carbon metabolism and kinetic modeling for heterologous proteins productions by Pichia pastoris in induction process with methanol/sorbitol co-feeding. Process Biochem. 2017;59:159–166.
- Zhang P, Zhang WW, Zhou XS, et al. Catabolite repression of Aox in Pichia pastoris is dependent on hexose transporter PpHxt1 and pexophagy. Appl Environ Microbiol. 2010;76:6108–6118.
- Berrios J, Flores M-O, Díaz-Barrera A, et al. A comparative study of glycerol and sorbitol as co-substrates in methanol-induced cultures of Pichia pastoris: temperature effect and scale-up simulation. J Ind Microbiol Biotechnol. 2017;44:407–411.
- Farré JC, Manjithaya R, Mathewson RD, et al. PpAtg30 tags peroxisomes for turnover by selective autophagy. Dev Cell. 2008;14:365–376.
- Liu T, Zhang J. High-level expression and characterization of Aspergillus niger ATCC 1015 xylanase B in Komagataella phaffii. Appl Biol Chem. 2018;61:373–381.
- Green MR, Sambrook J. Molecular cloning: A laboratory manual. 4th ed. New York (NY): Cold Spring Harbor Laboratory Press; 2012.
- Zhang J, Liu T. Energy charge as an indicator of pexophagy in Pichia pastoris. Front Microbiol. 2017;8:963.
- Zhang JG, Wang XD, Zhang JN, et al. Oxygen vectors used for S-adenosylmethionine production in recombinant Pichia pastoris with sorbitol as supplemental carbon source. J Biosci Bioeng. 2008;105:335–340.
- Tuttle DL, Dunn W. Divergent modes of autophagy in the methylotrophic yeast Pichia pastoris. J Cell Sci. 1995;108:25–35.
- Looser V, Brühlmann B, Bumbak F, et al. Cultivation strategies to enhance productivity of Pichia pastoris: a review. Biotechnol Adv. 2015;98:1177–1193.
- Yang Z, Zhang Z. Engineering strategies for enhanced production of protein and bio-products in Pichia pastoris: a review. Biotechnol Adv. 2018;36:182–195.
- Singh S, Gras A, Fiez-Vandal C, et al. Large-scale functional expression of WT and truncated human adenosine A2A receptor in Pichia pastoris bioreactor cultures. Microb Cell Fact. 2008;7:28.
- Zhang WH, Potter KJH, Plantz BA, et al. Pichia pastoris fermentation with mixed-feeds of glycerol and methanol: growth kinetics and production improvement. J Ind Microbiol Biotechnol. 2003;30:210–215.
- Inan M, Meagher MM. Non-repressing carbon sources for alcohol oxidase (AOX1) promoter of Pichia pastoris. J Biosci Bioeng. 2001;92:585–589.
- Azadi S, Mahboubi A, Naghdi N, et al. Evaluation of sorbitol-methanol co-feeding strategy on production of recombinant human growth hormone in Pichia Pastoris. Iran J Pharm Res. 2017;16:1555–1564.
- Moreira LRS, Filho E. Insights into the mechanism of enzymatic hydrolysis of xylan. Appl Microbiol Biotechnol. 2016;100:5205–5214.
- Collins T, Gerday C, Feller G. Xylanases, xylanase families and extremophilic xylanases. FEMS Microbiol Rev. 2005;29:3–23.
- Zhang M, Cai G, Zheng E, et al. Transgenic pigs expressing beta-xylanase in the parotid gland improve nutrient utilization. Transgenic Res. 2019;22:189–198.
- Frisvad JC, Moller LLH, Larsen TO, et al. Safety of the fungal workhorses of industrial biotechnology: update on the mycotoxin and secondary metabolite potential of Aspergillus niger, Aspergillus oryzae, and Trichoderma reesei. Appl Microbiol Biotechnol. 2018;102:9481–9515.