Abstract
Rice is a crucial crop to meet global food demands, therefore, it is necessary to develop rapid, efficient and inexpensive methods for screening mutants of important genes in molecular breeding. In this research, a simple microfluidic chip-integrated one-step polymerase chain reaction (PCR) and high-resolution melting (HRM) analysis were designed for the rapid screening of rice mutants. In order to complete the above processes, we also installed a self-developed functional instrument integrated with a temperature control system and a fluorescence detection department. This microfluidic platform was validated by analysing genes related to insect resistance, phenotype and yield of two important varieties of rice, Oryza sativa L. ssp. japonica. and Oryza sativa L. ssp. indica (93–11). The results showed that the platform could detect single-nucleotide mutations between the wild and the mutant rice plants in 1 h and the accuracy was consistent with gene sequencing regarded as the golden standard of the mutants screening. Compared to the traditional method (requiring approximately 3 h), this platform can distinguish mutants from wild types more rapidly. This system is a simple, integrated, disposable and low-cost tool for high-throughput screening of mutants in molecular breeding. More important, this portable instrument can be used as a point-of-care platform for nucleic acid diagnostics in many scientific fields.
Introduction
Rice is an important crop worldwide, meeting the carbohydrate demands of more than half of the world’s population and its global yield is directly related to food security [Citation1]. Rice molecular breeding can increase the efficiency of phenotypic selection, expand the range of available alleles, overcome the shortcomings of original varieties, shorten the breeding cycle and improve rice quality. At present, traditional methods for mutants identification, such as TaqMan probe, sequencing, gene chip and mass-spectrometry analysis are complicated to operate, with high cost and fail to detect unknown mutations [Citation2]. Sanger sequencing is the golden standard for short DNA fragment analysis of the mutants. Although the cost of sequencing has been reduced, a large number of mutant samples still need a lot of money in the work of varieties screening. Therefore, more efficient and economical methods for mutation scanning are urgently required.
High-resolution melting (HRM) analysis, a closed-tube post-polymerase chain reaction (PCR) method, used to identify variations of nucleic acid sequence [Citation3], has been used to differentiate species in molecular breeding. It monitors mutations on the basis of changes in fluorescence caused by the release of an intercalating DNA dye from a DNA duplex at the melting temperature [Citation4]. Because of the advantages of HRM analysis, such as easy in operating, closed-system environment, which greatly decreases contamination risk, and not relying on costly molecular probes [Citation5], HRM analysis has become an ideal choice for scanning for novel variants or genotyping known variants [Citation6]. A range of studies have reported that HRM analysis of PCR amplicons is a powerful method for detecting sequence variations at any position. Compared to other mutation detection methods, this one has the advantages of sensitivity, ability to detect high levels of heterozygosity and convenient and rapid operation [Citation7–9]. For example, Han et al. have developed an HRM analysis system for screening and genotyping single-nucleotide polymorphism (SNP) markers in diploid and tetraploid Medicago sativa L. [Citation10]. Tan et al. have used HRM to distinguish wild type and mutant rice and discover breeder-friendly functional molecular markers related to phytic acid [Citation11]. Ganopoulos et al. have used a DNA based method coupled with HRM analysis for rice product traceability, using five different microsatellite markers to genotype Basmati and non-Basmati varieties [Citation12].
However, although HRM provides analysis of mutant alleles, the pre-treatment of plant samples remains complicated. Generally, in traditional method plant tissues were needed to be frozen with liquid nitrogen and ground into powder. Subsequently, extracted samples must be centrifuged several times to remove particulate material, with the nucleic acid finally being extracted in elution buffer. Completing these procedures requires at least 3 h from extracting DNA to PCR product analysis. In the recent years, one-step PCR without traditional DNA extraction has been developed and reported in several studies [Citation13,Citation14]. Generally, samples require heating for only a few minutes. After neutralization and centrifugation, only a small amount of supernatant is needed for direct PCR.
Microfluidic platforms have emerged as promising tools for analysis of nanolitre volumes in various fields of molecular biology [Citation15,Citation16]. The advantages of microfluidic chips have been demonstrated in applications including on-chip PCR, DNA analysis, enzyme assays and chemical synthesis [Citation17]. The traditional method for nucleic acid analysis in the laboratory comprises of lysis, adsorption, washing and elution. Especially for plant samples, liquid nitrogen needs during grinding, and the entire process is time consuming and complicated. Exploiting microfluidic chips in nucleic acid analysis can greatly reduce reagent consumption, shorten assay time and realize precise temperature [Citation18,Citation19]. In the recent decades, microfluidic chips for nucleic acid analysis are mainly concentrated in biomedicine and point-of-care test (POCT) [Citation20,Citation21], but there are few studies in plants, especially in the detection of mutations.
In this article, we present a simple microfluidic device that integrates reagent mixing, one-step PCR and HRM analysis on-chip for rice mutant identification. To perform the above mentioned experimental procedures, a microfluidic chip with multilayer structure was designed and fabricated. Pneumatic valves, regulated by air pressure, were used to control the fluid and to achieve liquid mixing between different chambers on the chip. To realize thermal cycling during PCR and HRM, we designed a temperature control system based on thermoelectric cooler (TEC) to meet the requirements of precise temperature. In order to record the fluorescence during each PCR cycle and HRM, a compact fluorescence detection system was also designed. Then, we integrated the above three units into a scientific experimental instrument. In order to validate this microfluidic platform, we analysed genes important in rice breeding, such as brown plant-hopper (BPH) resistance gene from varieties Oryza sativa L. ssp. japonica, Oryza sativa L. ssp. indica (93–11) and unknown mutations after radiation mutagenesis on this integrated system. We also sequenced the amplified products of PCR and the results demonstrated that the proposed system could distinguish between wild type and mutant, even single-base difference. Compared with traditional method, this microfluidic system complete the reagent mixing, one-step PCR and HRM analysis within 1 h on the integrated chip greatly saving time. The promising results indicated that the developed system may be an auxiliary tool for screening of mutants for molecular breeding and other research.
Materials and methods
Chip design and fabrication
The microfluidic chip was fabricated from polydimethylsiloxane (PDMS) due to its high biocompatible, high transparency and low absorption characteristics. The chip was composed of four layers: three PDMS layers and a glass substrate as the base of the chip (). As shown in , the main function of the top layer was to store reagents and position gas interfaces connected to a power source so that the liquid can flow in the direction of differential pressure in response to negative pressure. The layer for reagent mixing had microstructures on both sides, with a liquid storage chamber on the up surface and valves for controlling fluid on the lower surface. The middle layer was a film layer, which connects the upper and lower two layers. The third layer was designed to control the fluid, which has a fluid passage for the above pneumatic valve. The PCR chamber used for amplification and HRM analysis on the other surface was next to the glass. Four layers were formed by plasma to generate the chip.
Figure 1. Schematic illustration of the integrated microfluidic chip, showing the four layers and chambers.
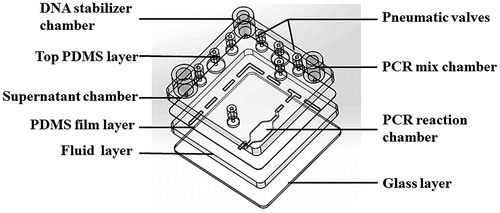
To control the fluid on the chip, we used pneumatic valves regulated by air pressure. The thickness of the film layer of the valve was about 100 μm. When 20 KPa pressure was applied to the channel, the gas exerts pressure on the film, deforming it and pressing the fluid channel downward to block the passage. When the pressure was removed, the PDMS valve film returns to its original position under its own force and the channel was unblocked. The pneumatic pump was composed of a series of pneumatic micro-valves in series and three pneumatic micro-valves form a simple PDMS peristaltic pump. By controlling the sequence of the three micro-valves, the liquid in the microchannel was expelled from one end of the pump to the other and thus, achieving flow and mixing of the liquid. In this chip, the fluid channel was 500 μm wide and 300 μm deep.
The microfluidic chip was fabricated through traditional protocols reported previously [Citation22]. Briefly, inverse patterns of the desired structures were produced through a machining process on metal moulds. Then, PDMS reagents were mixed at a mass ratio of 9:1 and baked at high temperature to create the microstructures. Finally, oxygen plasma was used to bind the four layers of the microfluidic chip.
Temperature control system
To control the temperature accuracy during the PCR and HRM assays, a sandwiched temperature control platform based on a thermoelectric cooler (TEC, FPH1-12707AC, Fulianjing Electronics, China) was designed according to the thermal cycling requirements. As shown in , a silver sheet (40 mm × 40 mm × 2 mm) was selected as the heat-transfer medium attached to the top of the TEC to attain spatial thermal homogeneity. And a slot was cut in the middle of the silver sheet for depositing a platinum sensor (Pt1000, Hayashi Denko, Japan), which was used to collect temperature information on the surface of the platform. The temperature signal was sent to amplifier circuit accurately through resistor bridge circuit and separated the analogue signal by 24-bit ADC converter, then sent to FPGA. Proportional integral derivative (PID) control algorithm was used to control the temperature on the chip adjusted to the set during PCR and HRM. A refrigerating radiator (NH-L9x65, NOCTUA, China) was used to dissipate heat rapidly during cooling process under the TEC.
Fluorescence acquisition and analysis
To confirm the fluorescence data obtained from each PCR cycle and incremental temperature point during HRM, a compact fluorescent detection system was designed. The system contained of two parts: a light emitting structure consisting of two light emitting diodes (LEDs, 475 ± 10 nm, CREE, Research Triangle Park, NC) with two filter set (excitation wavelength: 470 ± 25 nm; emission wavelength: 525 ± 25 nm, Bd-optic, China) and an optical imaging structure system based on a CCD camera (MC20, Mshot, China). The LEDs and filters were used for exciting the fluorescent dye. The CCD camera collected pictures during PCR and HRM analysis and converts fluorescent signals into numerical values by a built-in computer. Then, we integrated the temperature control system and the fluorescent detection system to be a scientific instrument with PCR and HRM function as shown in .
Sample preparation and experimental setup
Rice varieties
Indica and Japonica are two major subspecies of rice with different agronomic and culinary characteristics, which have been cultivated in China since ancient times. The entire genomes of the two species have been sequenced and widely used in breeding research as reference genome cultivars. Therefore, we selected Oryza sativa L. ssp. japonica. and Oryza sativa L. ssp. indica (93–11) as the experimental material for this study. In addition, we used mutants obtained through ion-beam irradiation, which expected to have some new mutation in certain domain.
Sample preparation
Rice seeds were disinfected with 5% NaClO for 3 min, then rinsed four times with sterile distilled water, then incubated on moistened filter paper in 9 cm diameter Petri dishes for 48 h at 28 °C in the dark, by which time seedling roots and shoots had emerged. The seedlings were exposed to culture conditions of 300 μmol m−2 s−1 and a 14 h photoperiod for 3 days. After 300 mg bud leaves were harvested, samples were immediately crushed in a tube and 90 μl lysis buffer (Herogen Biotechnology Co. Ltd., Shanghai, China) was added for lysis at 97 °C. The aim of this process was to accelerate cell lysis and to release nucleic acid. After the samples had cooled to room temperature, the mixture was centrifuged at speed of 2000 × g for 3 min and 5 μl supernatant was removed and added to the chip.
Specific PCR primers
The DNA sequences for BPH resistance gene, MYB transcription factor gene and unknown gene on chromosome1 of the respective varieties of rice were obtained from the National Center for Biotechnology Information (http://www.ncbi.nlm.nih.gov) (NCBI, USA) and the China Rice Data Center (http://www.ricedata.cn/index.htm). Primers for the specific sequences were designed in Primer Premier 5 software (). Primer pairs for all target regions were analysed for specificity with Primer-BLAST software in NCBI.
Table 1. The PCR primer sequences.
One-step PCR and HRM analysis on the microfluidic chip
To assess the ability of the integrated system to detect mutations, several rice genotypes were used for analysis. In addition, 5 μl extraction solution used for neutralizing superfluous proteins and impurities (Herogen Biotechnology Co. Ltd., Shanghai, China) and 10 μl PCR master mix (Roche, Switzerland) including 4 μmol L−1 primer mix, Taqenzyme, dNTP and Mg2+ were added to the chip. After mixed by the peristaltic pump, the reagent was transferred into the immobilization chamber for PCR and HRM. The PCR cycling programme was as follows: 95 °C for 10 min; 40 cycles of 95 °C for 20 s, 55 °C for 25 s and 72 °C for 25 s. The HRM process was performed at 95 °C for 60 s, then 40 °C for 60 s and the temperature was then ramped from 75 °C to 95 °C with a step of 0.1 °C for HRM. The CCD camera would collect singles at each temperature step.
Electrophoresis
To confirm the quality of the DNA extracted from rice leaves on the integrated chip, the DNA was transferred from the chip and analysed by slab-gel electrophoresis on a 1% (w/v) agarose gel.
Sequencing
To verify the accuracy of the HRM results, the PCR amplicons were sequenced by Sanger sequencing method at The Beijing Genomics Institute (BGI) and the data were interpreted using the Chromas software.
Results and discussion
Microfluidic implementation of nucleic acid analysis
Microfluidics miniaturizes nucleic acid extraction operations, such as lysis, adsorption, washing and elution on a functionally integrated chip. In this research, we adopted the improved hyperthermia pyrolysis method to extract genomic DNA from rice leaves. This method, compared to solid phase extraction based on silicon material or magnetic beads, is easier to implement on a microfluidic chip with a simple structure. As shown in , the leaves were boiled, the supernatant was transferred onto the chip and then uniformly mixed back and forth with extraction solution used for neutralizing superfluous proteins and impurities by a peristaltic pump. Subsequently, the above mixing operation is carried out between the extracted DNA and the PCR Master Mix, which are evenly mixed into the reaction chamber for PCR and HRM. All of these operations on the chip were completed in just 1 h and thus, saving substantial time compared to traditional laboratory approaches.
To confirm the quality of the DNA extracted from rice leaves on the integrated chip, the DNA was transferred from the chip and analysed by slab-gel electrophoresis. shows the quality of DNA extracted from different rice samples. The electrophoresis bands were clear and showed integrity, indicating that high-quality genomic rice DNA had been extracted on the integrated chip.
The instrument performance
Accurate temperature control is the key to PCR amplification and HRM analysis. In this research, a temperature control system based on TEC was designed. To meet the fast temperature response in the PCR stage, a U-type pipe heat dissipation structure was used according to the working mechanism of TEC, which improved the cooling efficiency of the system (). To achieve high-precision temperature control during the HRM analysis process, the feed-forward and piecewise PID algorithm was chosen as the control algorithm for the temperature control system. According to the difference between the measured temperature value and the set temperature value, we set different thresholds and adjusted the PID control parameters to make the temperature reach the set temperature rapidly and to minimize fluctuation in the set range.
The heating/cooling rate of the temperature control system directly affects the completion time of PCR amplification and HRM reaction. In order to evaluate the rate and the stability of this system, we set the temperature according to the PCR for many cycles. The results showed that the heating rate of the system was 4.5 °C s−1 and the cooling rate was 4 °C s−1 (), superior to those of conventional tube PCR (with an average rate of 3 °C s−1). In the heating curve, there was an overshoot when the temperature reached at the highest point. However, the temperature decreased rapidly and remained steady at the set point, which completed in 2 s. All of these aspects were based on the material of microfluidic chip and the design of the temperature control system. We used glass as the base of the microfluidic chip, to improve heat-transfer, increase the speed of thermal cycling and shorten the analysis time. The accuracy and precision of the instrument are also crucial. After changing of the temperature from 70 °C to 75.9 °C, the result revealed the high reliability with R2 = 0.9994 ().
Figure 5. Performance of the temperature control system. (a) Temperature cycle controlled by the microfluidic platform according to the planned PCR programme. From this curve, we calculated that the heating rate of the system was 4.5 °C s−1 and the cooling rate was 4 °C s−1. (b) Accuracy of the platform assessed by setting the temperature rise from 70 °C to 75.9 °C according to the HRM progress. The result revealed the high reliability with R2 = 0.9994.
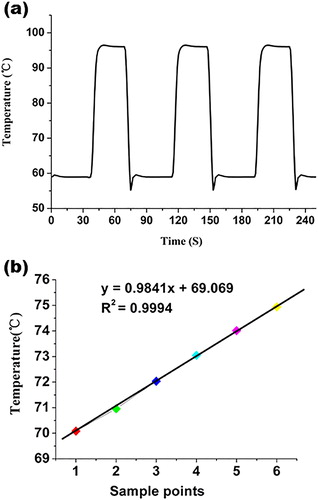
As HRM can distinguish small differences between DNA sequences, even single-base mutations. To realize this function, the temperature uniformity of the instrument must be ensured during the melting process. In order to verify the temperature uniformity of the system, we also used infra-red thermal imager to measure the real time temperature of different spots on the surface of the platform at each desired temperature in PCR. The result demonstrated that the uniformity of the platform can fully meet the requirement of HRM.
PCR and HRM analysis of rice mutants on the instrument
In this research, a microfluidic system integrated heating module and fluorescence detection system was designed and fabricated (). To investigate whether mutations could be detected by this microfluidic system, we used Oryza sativa L. ssp. japonica and Oryza sativa L. ssp. indica (93–11) mutant strains produced by ion-beam irradiation as the experimental material, and selected genes important in breeding, such as a pest-resistance gene and a transcription factor-encoding gene, as targets. To verify the accuracy of the genotyping results achieved by HRM on the chip, the products were also sequenced.
BPH is a migratory monophagous herbivore that has become the most damaging insect pest affecting rice [Citation23,Citation24]. Applying HRM to the selecting of carrying novel alleles of BPH resistance genes can accelerate the identification of genotypes of BPHS varieties. In this research, we used the novel disposable microfluidic chip to identify small differences in BPH resistance gene between the two rice cultivars. As indicated in , the two cultivars had clearly different melting profiles. Compared to the ability of capillary electrophoresis to discriminate PCR products of the same size, HRM is more sensitive at distinguishing between alleles.
Figure 6. HRM analysis of several important genes in rice breeding on the microfluidic system. HRM analysis of a BPH resistance gene (a), point mutation at chromosome 1 (b), and Oryza sativa L. with unknown mutations after radiation mutagenesis (c).
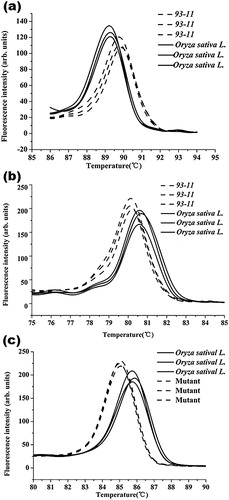
Although the genomes of Oryza sativa L. ssp. japonica and Oryza sativa L. ssp. indica (93–11) have been sequenced, there are still many genes with unknown functions. Using the integrated chip system for screening of mutants in breeding programmes can accelerate identification of the functions of individual genes. We selected one fragment on chromosome 1 which may be related with the yield of rice, amplified the target gene from the two cultivated varieties and analysed the product through HRM on-chip. From the melting curves in , there was an obvious difference in the sequence of this gene. It indicated that a base mutation may have occurred in this fragment between the two cultivated varieties. Then we sequenced the PCR product by the Sanger sequencing in BGI. The result indicated that there was a one-base mutant in this fragment, with the Oryza sativa L. ssp. indica (93–11) having the base of cytosine (C) and the Oryza sativa L. ssp. japonica having thymine (T) (Supplemental Figure S1), which may be related to the yield of the two cultivated varieties. This experiment demonstrated that the system could analyse the gene sequence of different varieties of rice and may be a useful tool for researching the function of unknown genes.
Brittle culm is an important constraint to high-yielding stable rice production. This mutation can lead to a decrease in the mechanical strength of stalks, thereby reducing the lodging resistance of rice [Citation25,Citation26]. In this study, we analysed a brittle culm mutant generated by ion-beam irradiation. The melting cures indicated that there were typical differences between the two species in the gene encoding the R2R3-MYB transcription factor, which is related to cellulose synthesis and the mechanical strength of rice (). We sequenced these fragments and found a 13 bp deletion (TCAGATACATCAC) in the mutant (Figure S1). Therefore, this integrated microfluidic system is useful to identify and screen for unknown mutants for improving the speed and efficiency of rice breeding.
Conclusions
This work demonstrated a new microfluidic instrument for the identification of mutants in rice molecular breeding. The system consisted of three units: a disposable chip, a U-type temperature control system based on TEC and a fluorescent detection system for recording the intensity variation. On the chip, several reactions like regent mixing, PCR and HRM were completed within 1 h. Compared to conventional methods, including manual extraction of nucleic acids, PCR in tube and capillary electrophoresis or mass-spectrometry analysis (requiring approximately 3 h), this chip greatly saves time while avoiding cross contamination. To evaluate the potential of the developed instrument, we measured the accuracy, uniformity and rate of the temperature control system, which were important for HRM analysis. In order to validate this microfluidic instrument, we selected several important genes related to insect resistance, phenotype and yield from two important varieties of rice, Oryza sativa L. ssp. japonica and Oryza sativa L. ssp. indica (93–11) as experimental material. From the results, when compared to the golden standard of Sanger sequencing, the microfluidic system demonstrated the ability to detect the mutants. Moreover, the chip in our research was easily to manufacture, low in cost and therefore, especially suitable for screening for mutants from large numbers of samples in breeding work. In summary, we successfully developed a new microfluidic system and innovatively applied it for a rapid and automatic mutation screening in molecular breeding. In the future, this simple, integrated, disposable, low-cost benchtop system has high potential as a point-of-care platform for nucleic acid diagnostics.
Supplemental Material
Download PDF (247.5 KB)Acknowledgements
The authors thank Professor Yuejin Wu, Institute of Technical Biology and Agriculture Engineering, Hefei Institutes of Physical Science, Chinese Academy of Sciences, for provision of the wild and the mutational varieties of rice.
Disclosure statement
No potential conflict of interest was reported by the authors.
Additional information
Funding
References
- Feng F, Li Y, Qin X, et al. Changes in rice grain quality of Indica and Japonica type varieties released in China from 2000 to 2014. Front Plant Sci. 2017;8:1863.
- Kao PC, Ding ST, Lin EC, et al. A bead-based single nucleotide polymorphism (SNP) detection using melting temperature on a microchip. Microfluid Nanofluid. 2014;17:477–488.
- Wittwer CT, Reed GH, Gundry CN, et al. High-resolution genotyping by amplicon melting analysis using LCGreen. Clin Chem. 2003;49:853–860.
- Krypuy M, Ahmed AA, Etemadmoghadam D, et al. High resolution melting for mutation scanning of TP53 exons 5-8. BMC Cancer. 2007;7:168.
- Wong MH, Henderson J, Drenth A. Identification and differentiation of Phyllosticta species causing freckle disease of banana using high resolution melting (HRM) analysis. Plant Pathol. 2013;62:1285–1293.
- Montgomery JL, Sanford LN, Wittwer CT. High-resolution DNA melting analysis in clinical research and diagnostics. Expert Rev Mol Diagn. 2010;10:219–240.
- Giffard PM, Andersson P, Wilson J, et al. CtGEM typing: discrimination of Chlamydia trachomatis ocular and urogenital strains and major evolutionary lineages by high resolution melting analysis of two amplified DNA fragments. PLoS One. 2018;13:e0195454.
- Tamburro M, Ripabelli G. High resolution melting as a rapid, reliable, accurate and cost-effective emerging tool for genotyping pathogenic bacteria and enhancing molecular epidemiological surveillance: a comprehensive review of the literature. Ann Ig. 2017;29:293–316.
- Karbalaie Niya MH, Basi A, Koochak A, et al. Sensitive high-resolution melting analysis for screening of KRAS and BRAF mutations in Iranian human metastatic colorectal cancers. Asian Pac J Cancer Prev. 2016;17:5147–5152.
- Han Y, Khu DM, Monteros MJ. High-resolution melting analysis for SNP genotyping and mapping in tetraploid alfalfa (Medicago sativa L.). Mol Breed. 2012;29:489–501.
- Tan Y-Y, Fu H-W, Zhao H-J, et al. Functional molecular markers and high-resolution melting curve analysis of low phytic acid mutations for marker-assisted selection in rice. Mol Breed. 2013;31:517–528.
- Ganopoulos I, Argiriou A, Tsaftaris A. Adulterations in Basmati rice detected quantitatively by combined use of microsatellite and fragrance typing with High Resolution Melting (HRM) analysis. Food Chem. 2011;129:652–659.
- Cheung SF, Yee MF, Le NK, et al. A one-pot, isothermal DNA sample preparation and amplification platform utilizing aqueous two-phase systems. Anal Bioanal Chem. 2018;410:5255–5263.
- Xiong D, Song L, Geng S, et al. One-step PCR detection of Salmonella pullorum/gallinarum using a novel target: the flagellar biosynthesis gene flhB. Front Microbiol. 2016;7:1863.
- Russom A, Ahmadian A, Andersson H, et al. Single-nucleotide polymorphism analysis by allele-specific extension of fluorescently labeled nucleotides in a microfluidic flow-through device. Electrophoresis. 2003;24:158–161.
- Fernandez-Carballo BL, McBeth C, McGuiness I, et al. Continuous-flow, microfluidic, qRT-PCR system for RNA virus detection. Anal Bioanal Chem. 2018;410:33–43.
- Park S, Zhang Y, Lin S, et al. Advances in microfluidic PCR for point-of-care infectious disease diagnostics. Biotechnol Adv. 2011;29:830–839.
- Hung TQ, Chin WH, Sun Y, et al. A novel lab-on-chip platform with integrated solid phase PCR and Supercritical Angle Fluorescence (SAF) microlens array for highly sensitive and multiplexed pathogen detection. Biosens Bioelectron. 2017;90:217–223.
- Amasia M, Kang S-W, Banerjee D, et al. Experimental validation of numerical study on thermoelectric-based heating in an integrated centrifugal microfluidic platform for polymerase chain reaction amplification. Biomicrofluidics. 2013;7:014106.
- Prakash R, Pabbaraju K, Wong S, et al. Integrated sample-to-detection chip for nucleic acid test assays. Biomed Microdev. 2016;18:44.
- Kim YT, Lee D, Heo HY, et al. Total integrated slidable and valveless solid phase extraction-polymerase chain reaction-capillary electrophoresis microdevice for mini Y chromosome short tandem repeat genotyping. Biosens Bioelectron. 2016;78:489–496.
- Zhu L, Zhu C, Deng G, et al. Rapid identification of H5 avian influenza virus in chicken throat swab specimens using microfluidic real-time RT-PCR. Anal Methods. 2014;6:2628.
- Hu J, Xiao C, He Y. Recent progress on the genetics and molecular breeding of brown planthopper resistance in rice. Rice (NY). 2016;9:30.
- Wang H, Ye S, Mou T. Molecular breeding of rice restorer lines and hybrids for Brown Planthopper (BPH) resistance using the Bph14 and Bph15 genes. Rice (NY). 2016;9:53.
- Kotake T, Aohara T, Hirano K, et al. Rice Brittle culm 6 encodes a dominant-negative form of CesA protein that perturbs cellulose synthesis in secondary cell walls. J Exp Bot. 2011;62:2053–2062.
- Ye Y, Liu B, Zhao M, et al. CEF1/OsMYB103L is involved in GA-mediated regulation of secondary wall biosynthesis in rice. Plant Mol Biol. 2015;89:385–401.