Abstract
In this study, spherical hollow mesoporous silica (HMS) particles with average size of 550 nm were loaded with dopamine-receptor agonist pramipexole, as a model drug. High drug loading (55%) was achieved by its incorporation in the reservoir structure of HMS. The newly developed drug-delivery systems were further double-coated with bioadhesive polymers chitosan and sodium alginate to achieve sustained release. The double chitosan/sodium alginate coating diminished the initial burst effect and lowered pramipexole release at two pH values (1.2 and 6.8) as shown by in vitro release studies. The haemolytic assay and MTT-test in human neuroblastoma SH-SY5Y cells showed good safety profile of the novel pramipexole-loaded HMS particles. Moreover, a more pronounced protection of human neuroblastoma SH-SY5Y cells against H2O2-induced oxidative damage was observed upon treatment with pramipexole-loaded (non-coated or chitosan-coated) HMS particles compared to the free drug. In conclusion, we found that HMS particles might be of great interest as a promising drug-delivery system for pramipexole. The coating with biopolymer chitosan modified both the drug-delivery process and the in vitro protection against oxidative stress in neuroblastoma SH-SY5Y cells.
Introduction
Although the exact mechanisms of Parkinson’s disease (PDs) are not fully understood, it is believed that excessive free radical formation, mitochondrial damage, oxidative stress and exposition to environmental toxins could be one of the key mechanisms leading to neuronal damage. Pramipexole (2-amino-4,5,6,7-tetrahydro-6-propylamino-benzethiazole-dihydrochloride) is indicated for the treatment of PDs, alone or in combination with levodopa. Its introduction in PDs therapy effectively reduced the dose of levodopa by approximately 30%, thus diminishing the common side effects from levodopa treatment [Citation1, Citation2]. Pramipexole is a full intrinsic dopamine-receptor agonist highly selective for the D2 receptors [Citation3]. In addition, pramipexole exhibits substantial neuroprotective effects by mechanisms independent of the dopaminergic receptors agonism [Citation4–7]. It was reported that pramipexole acts as a mitochondria-targeted antioxidant, inhibiting the mitochondrial hydrogen peroxide release and by increase in mitochondrial aconitase activity [Citation4, Citation8].
Besides the indisputable therapeutic efficacy, it was reported that the repeated daily administration of conventional forms of highly water soluble pramipexole (water solubility of about 200 mg/mL at 20–25 °C) is often accompanied by fluctuations in plasma drug concentrations, thus leading to dose-dependent side effects [Citation9]. A successful approach to overcome the problem is the incorporation of pramipexole in modified-release drug-delivery systems [Citation10]. The potential advantages of sustained released forms are mainly related to the simplification of patient’s administration scheme, reducing the number of daily intakes and attenuation of some dose-dependent adverse events.
Recent advantages in nanotechnology allow drugs to be encapsulated in appropriate drug release carriers aimed to provide a better neuroprotection by stimulating the drug delivery to damaged neurons [Citation11]. Mesostructured matrix silica materials have great advantages as drug-delivery carriers due to their good physicochemical properties. Generally, ordered mesoporous silica exhibit well defined pore structure, tuneable size and uniform shape of the pores, extremely high free surface area and relatively high total pore volume. Their inorganic nature and stable chemical structure lead to excellent thermal, hydrothermal, hydrolytic and mechanical stability [Citation12–17]. Systems based on mesoporous silica have been reported as dual pH-responsive mesoporous silica nanoparticles for efficient combination of chemotherapy and photodynamic therapy redox-triggered, enzymatic dependent and temperature responsive drug-delivery systems [Citation18–22]. Hollow mesoporous silica particles (HMS) have been developed as a new class of micro- and nano-capsules based on mesoporous silica material with a wide range of practical applications, including catalysis, confined synthesis, environmental protection and controlled release [Citation23–32]. In recent years, HMS particles have been widely explored as stimuli-responsive carriers [Citation33–37]. These particles combine the advantages of reservoir design and mesoporous structure, while possessing a higher storage capacity compared to conventional mesoporous materials [Citation38]. HMS are appropriate carriers for sustained drug release because their hollow core structure allows easier drug diffusion into the cavities through a high number of mesopores on the shell [Citation39–41]. It is generally accepted that reservoir structures with mesoporous shells can facilitate mass diffusion and drug transportation better than conventional hollow spheres of solid shells [Citation42]. Nevertheless, there are some particularities in the structure of HMS as possible drug-delivery carriers that should be kept in mind. All HMS’s mesopores are connected to the central core, so that poor control over drug release for the separate pores can lead to loss of the core’s content. This issue is much challenging especially for water soluble drugs, such as pramipexole. High water solubility can hinder the drug’s incorporation into sustained release forms because of the drug’s tendency to rapidly leach out of the dosage form upon exposure to an aqueous medium, such as gastrointestinal fluid. Several strategies have been proposed to overcome this problem. For example, Zhu et al. used chemically modified HMS as a suitable sustained release carrier for ibuprofen [Citation42]. They use a chemical functionalization with organic or inorganic groups which alters the physical/chemical properties and substantially changes the drug release profile. Polymer coating of mesoporous silica, which result in chances in particle permeability is another common and simple approach for drug release modification. A wide range of polymers have been used in the development of different modified-release and stimuli-responsive drug-delivery systems. The biocompatible and biodegradable natural biopolymers chitosan and sodium alginate are considered as appropriate coating agents. Sodium alginate is extensively investigated for use in biomedical applications [Citation43]. In acidic conditions (pH 1.2) sodium alginate precipitates as water insoluble alginic acid [Citation44], which makes it suitable for the outer shell of the new pramipexole delivery system. Thus, the alginate-based outer shell protects and keeps intact the inner, chitosan-based shell, in the passage through the stomach. On the other hand, chitosan possesses better bioadhesion at about pH 6.5 [Citation45, Citation46]. The introduction of chitosan in drug-delivery systems results in better intestinal mucoadhesion and promotes the bioavailability of the drug.
Here, we present the development of a new drug-delivery system based on pramipexole loading in HMS spheres. The drug loaded HMS particles were coated with sodium alginate or chitosan aiming to modify the release rate of pramipexole. The safety studies are an important step in the characterization of the new drug-delivery systems. Physico-chemical properties, such as particles size, charge or the type of coating agent might correlate with the toxicity responses following the contact with the cells in the biological system. Thus, we performed in vitro toxicity evaluation of pramipexole-loaded HMS particles by determination of their possible interaction with red blood cells (haemolysis assay). The cytotoxicity study in human neuroblastoma SH-SY5Y cells was performed to evaluate the safety profile of HMS as an appropriate drug-delivery system. Additionally, the antioxidant activity of pramipexol loaded HMS particles was determined in a model of H2O2-induced oxidative stress in neuroblastoma SH-SY5Y cells in vitro to prove the preservation of its biological activity.
Methods
Pramipexole loading and post-coating of the HMS mesoporous particles
The drug-loading procedure that we used was solvent impregnation. The procedure was carried out in water at 37 °C and applied 0.1 g of pramipexole per 0.1 g of HMS. After 24 h incubation time, the solvent (water) was vacuum evaporated and the drug loaded particles were dried under vacuum at room temperature overnight. The particles were washed several times with 95% of ethanol separated by vacuum-filtration (0.1 µm) and dried under vacuum for 24 h.
Polymer coating procedure: pramipexole-loaded HMS particles were incubated in 0.12% aqueous solution of sodium alginate/chitosan for 2 h using an electromagnetic stirrer. After the incubation time, the HMS/Prami dispersions were centrifuged at 15,000 rpm (Dragon Lab D2012 centrifuge) for 15 min, rinsed with distilled water, separated by second centrifugation, and finally dried under vacuum for 24 h. The samples were abbreviated as HMS/Prami (non-coated), HMS/Prami/Alg (alginate-coated), HMS/Prami/Chit (chitosan-coated) and HMS/Prami/Chit/Alg (double chitosan/alginate-coated).
Drug loading capacity
Drug loading of the particles (uncoated and coated) was calculated by determination of the amount of non-encapsulated pramipexole found in the supernatant during the loading/coating procedure. The amount of pramipexole in the supernatant was determined spectrophotometrically (262 nm, Thermo Scientific Evolution 300). The concentration of pramipexole was calculated according to the standard curve (r > 0.998).
Characterization of the particles
Transmission electron microscopy (TEM) characterization of HMS-based structures was performed by using a JEOL JEM 2100 HR STEM (200KV; point-resolution 0.23 nm).
Photon correlation spectroscopy and electrophoretic light scattering (Malvern Zetasizer Nano ZS, UK) was used as a method for determination of particle size and zeta-potential measurements. The particles (0.5 mg/mL) were dispersed in distilled water immediately before measurements, while scattering angles of 90° and 25 °C were used for the survey. The measurements were made in triplicate.
The texture of the parent HMS carrier and loaded and coated samples was studied by low-temperature nitrogen adsorption in a Quantachrome NOVA 1200e (USA) Instrument. The specific surface areas were determined from Brunauer Emmett Teller (BET) equation. The total pore volumes, mesopore size distributions and mean pore diameters were obtained by the NLDFT method.
Powder X-ray diffraction patterns were collected on a Bruker D8 Advance diffractometer with Cu Kα radiation and LynxEye detector within the range from 5.3 to 80° 2θ with a constant step of 0.02° 2θ and counting time of 52.5 s/step.
Attenuated total reflection infra-red (ATR-FTIR) spectra were recorded by spectrometer Nicolette 400. The IR spectra, in absorbance mode, were obtained over the spectral region of 400 to 4000 cm−1.
In vitro release studies
Dissolution tests were performed in acid and phosphate buffers with pH values 1.2 and 6.8, respectively. The dissolution tests were performed by the Paddle method with a stirring rate of 100 rpm at 37 °C (Erweka DT6, Germany). Samples were withdrawn at appropriate time intervals and replaced by fresh buffer. The withdrawn samples were centrifuged at 15,000 rpm (Dragon Lab D2012 centrifuge) for 15 minutes and the concentration of the released pramipexole was determined by ultraviolet (UV)-spectrophotometry at a wavelength of 262 nm [Citation47].
Haemolysis test
The haemolysis assay was performed, as described previously [Citation48]. Human erythrocytes were separated from blood by centrifugation in saline buffer and were subsequently resuspended in phosphate buffer (pH 7.4). Various concentrations of HMS (0.025, 0.05, 0.1, 0.2 and 1.0 mg/mL), positive (Triton X-100) and negative (water) controls were put into 96-well plates. After that, erythrocyte suspension was added to each well. Following incubation for 1 h at 37 °C, the plates were centrifuged (500 g) and the supernatant was transferred to new 96-well plates. The absorbance of haemoglobin was measured at 430 nm in a Synergy 2 plate reader (BioTek instruments). The results were represented as % over positive controls’ haemoglobin absorbance values with the negative controls’ values, accepted as zero haemolysis.
Cell culture and cultivation
SH-SY5Y cells were obtained from Sigma Aldrich (ECACC cell lines). They were routinely cultured in RPMI containing 10% (v/v) foetal calf serum, 2 mmol/L glutamine, 50 mg/mL penicillin and 100 mg/mL streptomycin and were kept at 37 °C in humidified 5% CO2 atmosphere.
MTT-dye reduction assay
SH-SY5Y cells were seeded in 96-well microplates at a density of 2 × 104 cells/well and were allowed to attach to the well surface for 24 h at 37 °C in humidified atmosphere with 5% CO2. After incubation, different concentrations of free or loaded pramipexole (concentrations corresponding to 1, 10, 20, 100 and 200 µg/mL) and empty HMS particles (2.14, 21.75, 43.45, 217.35 and 434.65 µg/mL) were added to cells, and incubated for 24 h. For each concentration, a set of at least 8 wells were used. The cell viability was estimated by MTT-dye (3-(4,5-dimethylthiazol-2-yl)-2,5-diphenyltetrazolium bromide) reduction assay [Citation49]. After the treatment, the solution in each well was substituted with MTT solution (0.5 mg/mL in culture medium). The microplates were further incubated for 3 h at 37 °C and the obtained formazan crystals were dissolved by 100 µL/well of DMSO. The absorbance was measured in a multiplate reader Synergy 2 (BioTek Instruments) at 570 nm (690 nm for background absorbance).
H2O2-induced oxidative stress model in vitro
In the oxidative stress model, SH-SY5Y cells at a density of 3 × 104 cells/well were exposed to 1 mmol/L H2O2 for 15 min to obtain submaximal cytotoxicity. In order to estimate protection, cells were pre-treated with free or loaded pramipexole (concentrations, corresponding to 1, 10, 20, 100 and 200 µg/mL) and empty HMS particles (2.14, 21.75, 43.45, 217.35 and 434.65 µg/mL) for 1 h before treatment with H2O2. The cell viability was estimated by MTT-dye (3-(4,5-dimethylthiazol-2-yl)-2,5-diphenyltetrazolium bromide) reduction assay [Citation49].
Statistical analysis
The analyzed data points were normalized to the mean values of corresponding control groups, each of the respective 96-well plates. ANOVA (one-way analysis of variance) with Dunnett post-hoc test was used to calculate the differences between the means among the experimental groups treated with a corresponding concentration. Differences were accepted to be significant when p < 0.05; p < 0.01; p < 0.001. All statistical analysis was carried out on Graph Pad 5 software.
Results and discussion
Physicochemical characterization of the drug-delivery systems
The particle size measurements showed insignificant differences between uncoated and coated samples. The average size was between 550 nm (for initial empty particles) and 660 nm (double-coated samples). However, there were some differences related mainly to the polymer coating of the particles, which were most pronounced for double chitosan/alginate-coated particles (. These results were an indication for successful polymer coating of all the samples. The polydispersity index () changed after polymer coating, and this was more pronounced for chitosan and chitosan/alginate-coated particles.
Figure 1. Particle size (A) and polydispersity index (B) of uncoated (HMS/Prami), Na alginate-coated (HMS/Prami/Alg), chitosan-coated (HMS/Prami/Chit) and double chitosan-alginate-coated (HMS/Prami/Chit/Alg) particles.
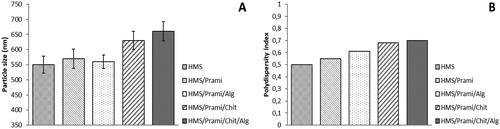
Zeta-potential measurements were used as indicator for successful polymer coating. The empty particles demonstrated a negative value typical of HMS (). The pramipexole incorporation in the empty HMS led to a significant change in the surface charge of the particles from strongly negative to weakly positive. These data can be explained with the post-loading influence of the amino-group in the structure of pramipexole expressed in the mesopores and probably on the outer surface of the HMS particles. The HMS/Prami particles coated with positively charged polymer chitosan showed a positive charge of the surface (+37.1 mV), which is typical of this type of polymer and indicates successful coating of the pramipexole-loaded particles. The zeta-potential measurements of HMS/Prami/Alg and HMS/Prami/Chit/Alg showed negative surface charge, which is typical of sodium alginate. No significant differences between HMS/Prami/Alg and HMS/Prami/Chit/Alg were observed, which was related with minimization of the influence of chitosan coating and respectively this indicated successful double coating.
Figure 2. Zeta-potential of uncoated (HMS/Prami), Na alginate-coated (HMS/Prami/Alg), chitosan-coated (HMS/Prami/Chit) and double chitosan-alginate-coated (HMS/Prami/Chit/Alg) particles.
Note: Data are means with standard deviation (± SD).
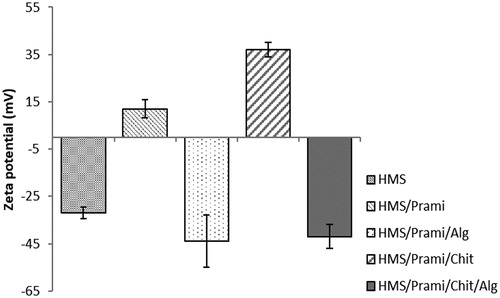
Further, the shape of the particles and the preservation of the porous structure were demonstrated by TEM technique. The TEM images () are in good accordance with the size measurements. The images of uncoated () and double chitosan-alginate-coated () samples show spherical shape of the particles and preserved porous structure of both samples.
Figure 3. TEM images of uncoated HMS (A) and chitosan/alginate-coated HMS (B), loaded with pramipexole.
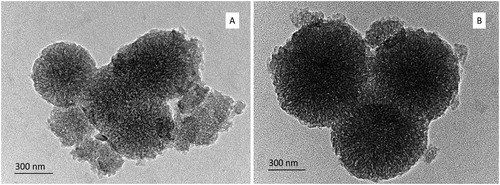
The powder X-ray diffraction patterns of pramipexole, HMS carrier and loaded and coated samples are presented in . The pattern of pramipexole shows that this compound crystallizes in a monoclinic SG P2 with unit cell parameters a = 7.19 Å, b = 27.42 Å, c = 7.63 Å and β = 90.13°. After the loading and coating processes, the intensities of peaks of the crystalline pramipexole almost disappear, indicating amorphization or fine dispersion of the drug on the surface and in the pores of the HMS substrate. For Alg coated samples some peaks attributed to crystalline NaCl and KCl were seen due to the preparation procedure.
N2 adsorption isotherms and pore-size distributions of the drug loaded and coated samples along with the parent HMS silica carrier are presented in and the texture characteristics are summarized in .
Figure 5. N2 adsorption-desorption isotherms (A) and pore-size distribution (B) of unloaded (HMS) as insets, and drug loaded and coated particles.
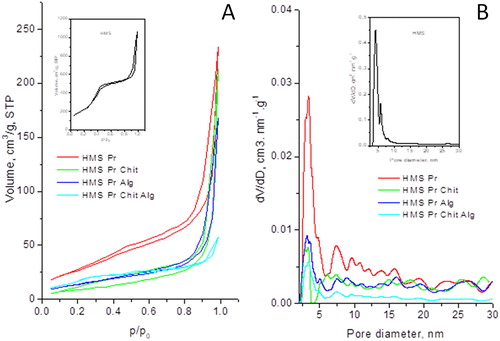
Table 1. Texture characteristics.
The isotherm of the carrier HMS is of type IV according to the IUPAC classification, which is characteristic of mesoporous materials () [Citation50]. The isotherm presents three well resolved regions due to monolayer–multilayer adsorption, increase in the adsorbed volume at p/p0=0.2–0.4 due to capillary condensation, followed by linear increase in the adsorbed volume at high pressure due to multilayer adsorption on the outer surface. The isotherm of HMS was characterized with a steep increase in nitrogen uptake at high relative pressure p/p0= 0.9–1.0 and a wide H4 hysteresis, typical of mesoporous solid materials. Large condensation of nitrogen manifested by a sharp increase of the adsorbed amount at relative pressure above 0.9 indicates the presence of large mesopores or inter-particle adsorption and condensation. The pore-size distribution indicated bimodal distribution as well. Neither parent nor the loaded and coated materials exhibited micro-porosity. For loaded and coated samples, H2-type hysteresis loops were present, and they were associated with less ordered mesoporous structures.
After pramipexole loading and subsequent coating with Chit and Alg, a dramatic change in the texture parameters was observed: a huge decrease in the specific surface areas and pore volumes (). This is expectable, as the drug and the modifiers could fill or block the pores or the pore entrances. However, the decrease in the average pore diameter was not so significant, indicating homogeneous distribution of the drug and the modifiers on the surface and in the pores of HMS. This is in good agreement with the XRD data, which revealed that the loaded and coated particles were well dispersed.
FTIR spectra of HMS, pramipexole, alginate, chitosan and pramipexole-loaded HMS and loaded nanoparticles coated with chitosan, alginate and chitosan/alginate are shown in . The characteristic absorption peaks of the pure drug were found at the wave numbers of 3409, 2945, 1585, 1308 and 759 cm−1, representing the functional groups of N-H stretching, C-H stretching, C = C stretching, C-N stretching and C-H bending, respectively [Citation51]. The FT-IR spectrum of HMS was characterized by an intensive band (not shown) at 1077 cm−1, which was due to the asymmetric stretching vibration of the silica structure (Si–O–Si) [Citation52, Citation53]. After loading of pramipexole on the particles, the vibration band of the drug shifted to 2972 cm−1 (NH3+) and the characteristic band of the silica structure (Si–O–Si) to 1054 cm−1, which can indicate some interaction between the NH2 group of pramipexole and the silica silanol groups. In the spectrum of chitosan, the absorption band at 1652 cm−1 corresponded to the carbonyl (C = O) stretching of the secondary amide. The band at 1596 cm−1 present in the IR spectrum of sodium alginate was assigned to symmetric stretching peaks of carboxylate salt groups [Citation54]. In the IR spectrum of pramipexole-loaded HMS coated with chitosan, the absorption band of chitosan shifts to 1632 cm−1, indicating possible interaction with silanol groups on the surface of HMS particles. In the IR spectrum of pramipexole-loaded HMS coated with alginate, a symmetrical stretching of –COO− groups of alginate shifted to 1585 cm−1, indicating possible interaction with pramipexole protonated amino groups. The new peak at 1632 cm−1 showed –COOH groups of alginate and the possiblе formation оf H-bonds with silanol groups on the surface of HMS particles. In the IR spectrum of pramipexole-loaded HMS coated with chitosan/alginate, the absorption band corresponding to the carbonyl stretching of the secondary amide shifted slightly to 1629 cm−1 the symmetrical stretching of the –COO− groups of alginate shifted slightly to 1590 cm−1 and no changes in the NH3+ peak at 2970 cm−1 occurred, which gives reason to believe that there is no electrostatic interaction between chitosan and alginate and pramipexole in the double-coated particles.
Drug loading
The loading capacity for all uncoated and coated samples is presented in . Due to the high free volume and core structure of HMS particles, the uncoated samples showed high drug loading of 55%. The process of polymer coating led to slight reduction in the loading rate, this change being more distinct for alginate and double-coated particles (46 and 44%, respectively). Chitosan coating led to an insignificant decrease in the amount of pramipexole into the particles.
Drug delivery
Buffers with different pH (1.2 and 6.8) were used for drug-delivery studies. These pH values are typically applied to mimic the in vivo conditions in the gastrointestinal tract. Different release profiles were established exploring coated and non-coated particles. Non-coated particles registered complete release of pramipexole within 15 minutes at both pH values. There was alteration of HMS particles permeability, which resulted in prolongation of drug release profile, following surface polymer modification. Modification of the particles with sodium alginate resulted in minimization of the initial burst-release effect. This was more visible at pH = 1.2 than at pH = 6.8 (). The chitosan-coated particles showed a different dissolution profile. The release of pramipexole at pH = 1.2 was more rapid compared to that at pH 6.8, where the drug release was far less rapid. Our results show that at lower pH the chitosan-coated particles would release the drug in the gastrointestinal fluid within 30 minutes following oral administration. Moreover, the presence of digestive enzymes in the stomach fluid could further accelerate chitosan degradation and drug release [Citation55]. The change of pH to 6.8 led to limited solubility of chitosan and a significant decrease in the drug release rate.
Figure 8. In vitro pramipexole release from uncoated (HMS/Prami), Na alginate-coated (HMS/Prami/Alg), chitosan-coated (HMS/Prami/Chit) and double chitosan-alginate-coated (HMS/Prami/Chit/Alg) particles at pH = 1.2 and pH = 6.8.
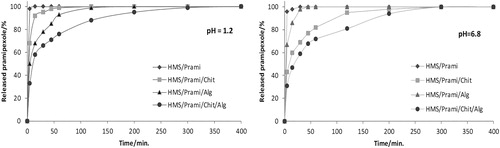
The combination of the two polymers led to a significant reduction in the initial burst-release effect at both pH values. The minimization of the released pramipexole at these different pH values was similar.
Haemolysis assay
The red blood cells (RBC) play a fundamental role in the circulatory system transporting oxygen, thus the assessment of the interaction of HMS with RBC is an important part of any in vitro toxicity evaluation. The haemolytic potential of various concentrations of HMS (0.025, 0.05, 0.1, 0.2 and 1.0 mg/mL) was tested in human erythrocytes (). We found that the haemolysis rate of HMS was lower than 5% and this result met the standard according to ISO/TR7405-1984(E). A slight increase in the haemolytic potential was observed in a concentration of 1 mg/mL, but this concentration is quite high and unlikely to be used in vivo. Most probably the observed effect is due to the electrostatic interactions between the negatively charged HMS and positively charged phosphotidylcholine on the outer membrane surface of erythrocytes.
Figure 9. Hemolysis assay of hollow silica nanoparticles (HMS).
Note: Mean values with standard deviation (± SD) of three independent experiments (n = 5). **p < 0.01; ***p < 0.001 vs. control value.
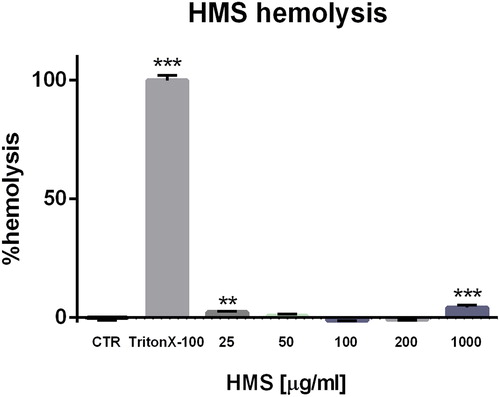
Our results confirmed that the HMS particles have good biocompatibility, indicating that they could be considered appropriate as a drug-delivery system. In fact, some studies on the effects of porous silica particles on erythrocytes reported low haemolytic activity [Citation56]. Their good hemocompatibility could be due to complex mechanisms, such as a decreased contact area of porous silica particles with the erythrocyte membranes and the fact that porous silica, in contrast to nonporous silica analogues, degrade to non-toxic orthosilicic acid [Citation57, Citation58].
In vitro cytotoxicity evaluation in SH-SY5Y cells
Cell viability is frequently used as a measurement of drugs/particle induced cellular injury. The effects of free pramipexol, empty HMS and pramipexol loaded HMS particles (single- or double-coated with chitosan/sodium alginate) on SH-SY5Y cell viability were evaluated by using MTT assay, as a marker of mitochondrial function (). The results showed lack of toxicity of free Prami, empty HMS, HMS/Prami, HMS/Prami/Chit (pramipexole concentration 1.0–200.0 µg/mL), since no significant changes in the cell viability were observed. Microphotographic observations revealed that SH-SY5Y morphology and the cell monolayer integrity were preserved (data not shown). HMS/Prami/Alg and HMS/Prami/Chit/Alg particles were not cytotoxic in low concentrations (1 and 10 µg/mL), whereas the cell viability detected at the higher concentrations (20, 100 and 200 µg/mL). Similar behavior of alginate containing particles was observed and already reported in our previous study [Citation59]. There, we reported that sodium alginate-based nanoparticles caused cytotoxicity, determined by increased LDH leakage in HepG2 cells, in contrast to chitosan-based particles, which were non-toxic. Our in vivo study showed that alginate-based particles caused slight changes in the levels of red blood count (RBC), haemoglobin (HGB) and haematocrit (HCT). The mechanisms underlying the effects of alginate on some haematology parameters are also discussed in the report of Xu et al. [Citation60]. The authors suggest that the higher degree of aggregation of RBCs caused by alginate particles is mostly dependent on the differences in alginate molecular weight.
Figure 10. Effects of free pramipexol (Prami), uncoated (HMS/Prami), sodium alginate-coated (HMS/Prami/Alg), chitosan-coated (HMS/Prami/Chit) and double-coated chitosan-sodium alginate (HMS/Prami/Chit/Alg) particles, on cell viability of SH-SY5Y neuroblastoma cells after 24 h treatment.
Note: Mean values ± SD from three independent experiments (p < 0.001).
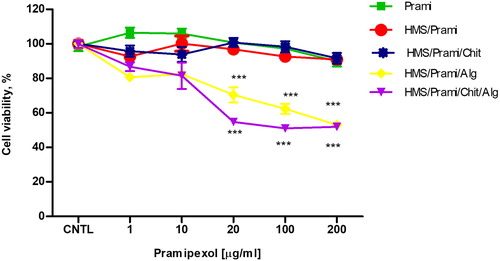
Protective effects of pramipexol loaded HMS formulations on oxidative stress model in human neuroblastoma SH-SY5Y cells in vitro
Cassarino et al. [Citation61] demonstrated neuroprotective effects of pramipexole mediated by mechanisms including inhibition of oxidative stress and free radical overproduction, and through maintenance of the cellular antioxidant status. We successfully developed chitosan/sodium alginate HMS particles as a novel drug-delivery system for pramipexole. Hence, it is important to prove the preservation of the antioxidant activity of pramipexole following its incorporation in HMS particles.
The antioxidant effects of newly formulated pramipexole-loaded HMS particles (both uncoated or chitosan and/or sodium alginate-coated) were further explored in a model of H2O2 induced oxidative stress damage in human neuroblastoma SH-SY5Y cells (). H2O2 induces cell damage by production of reactive hydroxyl radicals and byproducts (Fenton’s reaction), which further interact with intracellular components causing injury to the cell proteins, lipids and DNA. H2O2 treatment (1 mmol/L, 15 min) of SH-SY5Y cells caused significant toxicity and decreased the cell survival by 40% vs. the untreated control (p < 0.001) (). Free pramipexol and HMS/Prami particles showed high protection, especially in concentrations of 100 and 200 µg/mL (). Our results demonstrate that at the lower pramipexole concentrations (1–20 µg/mL), the protection of HMS/Prami and HMS/Prami/Chit particles was even enhanced compared to the free, unloaded drug. We suppose that the improved antioxidant effect of the encapsulated pramipexole was most probably related to the carrier’s properties. In fact, uniform mesoporous silica nanocarriers can facilitate the membrane permeability [Citation62]. The efficiency of the cellular uptake is also influenced by the particle’s adhesion and their interaction with the cells. An improved biological activity has also been established for other antioxidants loaded in mesoporous silica nanoparticles [Citation63, Citation64].
Figure 11. Protective antioxidant effects of free pramipexol (Prami), uncoated (HMS/Prami), Na alginate-coated (HMS/Prami/Alg), chitosan-coated (HMS/Prami/Chit) and double chitosan-alginate-coated (HMS/Prami/Chit/Alg) particles, in H2O2-induced oxidative stress in SH-SY5Y cells treated for 15 min with 1 mmol/L H2O2.
Note: Cell viability was determined after 24 h. Data are mean values ± SD. All groups were compared by one-way ANOVA with Dunnet’s post-test vs. H2O2–treatment. ***p < 0.001.
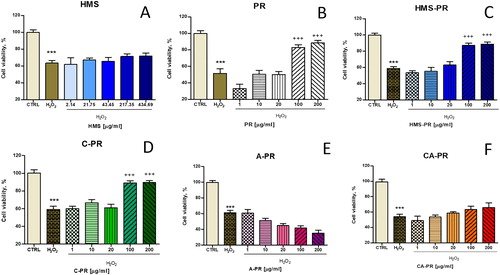
An interesting finding was that the antioxidant efficiency of pramipexole-loaded HMS nanoparticles was dependent on the type of coating agent (chitosan > chitosan/alginate > alginate containing particles). Thus, chitosan-coated HMS/Prami/Chit particles (100 and 200 µg/mL) also demonstrated efficient prevention of the cell viability decrease, whereas the HMS/Prami/Chit/Alg were less effective (). In contrast, HMS/Prami/Alg did not show any protection in H2O2 induced injury of neuronal SH-SY5Y cells (). Our observations on enhanced antioxidant activity of chitosan-containing particles loaded with pramipexole supported a recent report by Raj et al. [Citation65]. The authors reported a superior pharmacological antioxidant effect of pramipexole-loaded chitosan nanoparticles for intranasal administration, measured as increased superoxide dismutase and catalase activities in rat brain tissue homogenates [Citation65]. These examples demonstrate that nanotechnology continues to attract considerable attention in neurological pathology by providing successful approaches to facilitate drug delivery, to improve the sustained release efficacy and to reduce the intrinsic toxicity of drugs, thus offering numerous advantages over the conventional dosage forms.
Conclusions
In this study, we report a new formulation of dopamine-receptor agonist pramipexol in hollow mesoporous silica particles (HMS). HMS particles showed high drug loading capacity (55%) due to the reservoir structure and high free volume (1.28 cm3/g). Modified drug delivery was achieved following coating with two natural polymers, chitosan and sodium alginate. Significant reduction in the initial burst effect was visible at both pH values (1.2 and 6.8). Excellent biocompatibility of pramipexole-loaded HMS particles was confirmed by the low haemolytic potential of HMS. Additionally, a beneficial safety profile and preserved antioxidant properties of pramipexole-loaded HMS particles were proved in neuronal cells in vitro. Thus, HMS nanoparticles could be considered as a promising and safe carrier for pramipexole in the treatment of neurodegenerative diseases, such as Parkinson’s disease. The proposed formulation of pramipexole-loaded HMS particles provides a possibility for sustained release and therefore less frequent dosing, while keeping the antioxidant activity and neuroprotection. The simplification of the pramipexole administration scheme by reducing the drug’s daily intakes would improve the patients’ compliance and attenuate some frequent dose-dependent adverse events. In turn, a better symptom control is expected in Parkinson’s disease treatment.
Acknowledgements
This work was supported by the Bulgarian Ministry of Education and Science under the National Program for Research ‘Young Scientists and Postdoctoral Students’.
Disclosure statement
The authors report no conflicts of interest. The authors alone are responsible for the content and writing of the article.
References
- Weiner WJ, Factor SA, Jankovic J, et al. The long-term safety and efficacy of pramipexole in advanced Parkinson’s disease. Parkinsonism Relat Disord. 2001;7:115–120.
- Moller JC, Oertel WH, Koster J, et al. Long-term efficacy and safety of pramipexole in advanced Parkinson’s disease: results from a European multicenter trial. Mov Disord. 2005;20:602–610.
- Kreiss DS, Bergstrom DA, Gonzalez AM, et al. Dopamine receptor agonist potencies for inhibition of cell firing correlate with dopamine D3 receptor binding affinities. Eur J Pharmacol. 1995;277:209–214.
- Danzeisen R, Schwalenstoecker B, Gillardon F, et al. Targeted antioxidative and neuroprotective properties of the dopamine agonist pramipexole and its nondopaminergic enantiomer SND919CL2x [(+)2-amino-4,5,6,7-tetrahydro-6-Lpropylamino-benzathiazole dihydrochloride]. J Pharmacol Exp Ther. 2006;316:189–199.
- Kitamura Y, Kosaka T, Kakimura JI, et al. Protective effects of the antiparkinsonian drugs talipexole and pramipexole against 1-methyl-4-phenylpyridinium-induced apoptotic death in human neuroblastoma SH-SY5Y cells. Mol Pharmacol. 1998;54:1046–1054.
- Gu M, Iravani MM, Cooper JM, et al. Pramipexole protects against apoptotic cell death by non-dopaminergic mechanisms. J Neurochem. 2004;91:1075–1081.
- Abramova NA, Cassarino DS, Khan SM, et al. Inhibition by R(+) or S(-) pramipexole of caspase activation and cell death induced by methylpyridinium ion or beta amyloid peptide in SH-SY5Y neuroblastoma. J Neurosci Res. 2002;67:494–500.
- Ferrari-Toninelli G, Maccarinelli G, Uberti D, et al. Mitochondria-targeted antioxidant effects of S (-) and R (+) pramipexole. BMC Pharmacol. 2010;10:2.
- Pellicano C, Benincasa D, Fanciulli A, et al. The impact of extended release dopamine agonists on prescribing patterns for therapy of early Parkinson’s disease: an observational study. Eur J Med Res. 2013;18:60.
- Schapira AH, Barone P, Hauser R, et al. Efficacy and safety of pramipexole extended-release for advanced Parkinson’s disease [Abstract]. Mov Disord. 2009;24:277.
- Linazasoro G, Nanotechnologies for Neurodegenerative Diseases Study Group of the Basque Country (NANEDIS). Potential applications of nanotechnologies to Parkinson’s disease therapy. Parkinsonism Relat Disord. 2008;14:383–392.
- Beck JS, Vartuli JC, Roth WJ, et al. A new family of mesoporous molecular sieves prepared with liquid crystal templates. J Am Chem Soc.. 1992;114:10834–10843.
- Yang XY, Zhang SB, Qiu ZM, et al. Stable ordered mesoporous silica materials templated by high-temperature stable surfactant micelle in alkaline media. J Phys Chem B. 2004;108:4696–4700.
- Jiang T, Shen W, Tang Y, et al. Stability and characterization of mesoporous molecular sieve using natural clay as a raw material obtained by microwave irradiation. Appl Surf Sci. 2008;254:4797–4805.
- Yoncheva K, Tzankov B, Popova M, et al. Evaluation of stability of mesoporous silica nanoparticles and their further formulation in tablet form. J Dispers Sci Technol. 2016;37:113–118.
- Song K, Guan J, Wang Z, et al. Post-treatment of mesoporous material with high temperature for synthesis super-microporous materials with enhanced hydrothermal stability. Appl Surf Sci. 2009;255:5843–5846.
- Wang Y, Zhao Q, Han N, et al. Mesoporous silica nanoparticles in drug delivery and biomedical applications. Nanomedicine. 2015;11:313–327.
- Niedermayer S, Weiss V, Herrmann A, et al. Multifunctional polymer-capped mesoporous silica nanoparticles for pH-responsive targeted drug delivery. Nanoscale. 2015;7:7953–7964.
- Yao XM, Chen XF, He CL, et al. Dual pH-responsive mesoporous silica nanoparticles for efficient combination of chemotherapy and photodynamic therapy. J Mater Chem B. 2015;3:4707–4714.
- Li ZY, Hu JJ, Xu Q, et al. A redox-responsive drug delivery system based on RGD containing peptide-capped mesoporous silica nanoparticles. J Mater Chem B. 2015;3:39–44.
- Liu J, Zhang B, Luo Z, et al. Enzyme responsive mesoporous silica nanoparticles for targeted tumor therapy in vitro and in vivo. Nanoscale. 2015;7:3614–3626.
- Baek S, Singh RK, Khanal D, et al. Smart multifunctional drug delivery towards anticancer therapy harmonized in mesoporous nanoparticles. Nanoscale. 2015;7:14191–14216.
- Tang S, Huang X, Chen X, et al. Hollow mesoporous zirconia nanocapsules for drug delivery. Adv Funct Mater. 2010;20:2442–2447.
- Yeo KM, Choi S, Anisur RM, et al. Surfactant‐free platinum‐on‐gold nanodendrites with enhanced catalytic performance for oxygen reduction. Angew Chem Int Ed. 2011;50:745–748.
- Liu S, Yu J, Jaroniec M. Tunable photocatalytic selectivity of hollow TiO2 microspheres composed of anatase polyhedra with exposed {001} facets. J Am Chem Soc. 2010;132:11914–11916.
- Caruso F, Caruso RA, Möhwald H. Nanoengineering of inorganic and hybrid hollow spheres by colloidal templating. Science. 1998;282:1111–1114.
- Sun Y, Mayers B, Xia Y. Metal nanostructures with hollow interiors. Adv Mater. 2003;15:641–646.
- An K, Hyeon T. Synthesis and biomedical applications of hollow nanostructures. Nano Today. 2009;4:359–373.
- Hu J, Chen M, Fang X, et al. Fabrication and application of inorganic hollow spheres. Chem Soc Rev. 2011;40:5472–5491.
- Li L, Tang F, Liu H, et al. In vivo delivery of silica nanorattle encapsulated docetaxel for liver cancer therapy with low toxicity and high efficacy. ACS nano. 2010;4:6874–6882.
- Nejabat M, Mohammadi M, Abnous K, et al. Fabrication of acetylated carboxymethylcellulose coated hollow mesoporous silica hybrid nanoparticles for nucleolin targeted delivery to colon adenocarcinoma. Carbohydr Polym. 2018;197:157–166.
- Fang J, Liu Y, Chen Y, et al. Graphene quantum dots-gated hollow mesoporous carbon nanoplatform for targeting drug delivery and synergistic chemo-photothermal therapy. IJN. 2018;Volume 13:5991. [cited 2019 May 28]
- Moghaddam SPH, Yazdimamaghani M, Ghandehari H. Glutathione-sensitive hollow mesoporous silica nanoparticles for controlled drug delivery. J Control Release. 2018;282:62–75.
- Jia X, Yang Z, Wang Y, et al. Hollow mesoporous silica metal-organic framework and applications for pH‐responsive drug delivery. Chem Med Chem. 2018;13:400–405.
- Huang L, Liu J, Gao F, et al. A dual-responsive, hyaluronic acid targeted drug delivery system based on hollow mesoporous silica nanoparticles for cancer therapy. J Mater Chem B. 2018;6:4618–4629.
- Park SS, Ha CS. Hollow mesoporous functional hybrid materials: fascinating platforms for advanced applications. Adv Funct Mater. 2018;28:1703814.
- Yang Q, Li L, Zhao F, et al. Hollow silica–polyelectrolyte composite nanoparticles for controlled drug delivery. J Mater Sci. 2019;54:2552–2565.
- Teng Z, Li W, Tang Y, et al. Mesoporous organosilica hollow nanoparticles: synthesis and applications. Adv Mater. 2018;2018:1707612.
- Chen Y, Chu C, Zhou Y, et al. Reversible pore‐structure evolution in hollow silica nanocapsules: large pores for siRNA delivery and nanoparticle collecting. Small. 2011;7:2935–2944.
- Gao F, Li L, Liu T, et al. Doxorubicin loaded silica nanorattles actively seek tumors with improved anti-tumor effects. Nanoscale. 2012;4:3365–3372.
- Zhu YF, Shi JL, Li YS, et al. Storage and release of ibuprofen drug molecules in hollow mesoporous silica spheres with modified pore surface. Microporous Mesoporous Mater. 2005;85:75–81.
- Zhu Y, Shi J, Shen W, et al. Preparation of novel hollow mesoporous silica spheres and their sustained-release property. Nanotechnology 2005;16:2633.
- Gombotz WR, Wee S. Protein release from alginate matrices. Adv Drug Deliv Rev. 1998;31:267–285.
- McNeely WH, Kang KS. Xanthan and some other biosynthetic gums. Indus Gums. 1973;2:473–497.
- Lee DW, Lim C, Israelachvili JN, et al. Strong adhesion and cohesion of chitosan in aqueous solutions. Langmuir 2013;29:14222–14229.
- Lim C, Lee DW, Israelachvili JN, et al. Contact time- and pH-dependent adhesion and cohesion of low molecular weight chitosan coated surfaces. Carbohydr Polym. 2015;117:887–894.
- Suddhasattya D, Prasanna KP, Upadhayay UM, et al. Method development and validation of pramipexole by UV spectrophotometric method. J Pharm Res. 2012;5:5052–5054.
- Evans BC, Nelson CE, Shann S, et al. Ex vivo red blood cell hemolysis assay for the evaluation of pH-responsive endosomolytic agents for cytosolic delivery of biomacromolecular drugs. J Vis Exp. 2013;2013:e50166.
- Mosmann T. Rapid colorimetric assay for cellular growth and survival: application to proliferation and cytotoxicity assays. J Immunol Method. 1983;65:55–63.
- Sing KSW, Everett DH, Haul RHW, et al. International union of pure and applied chemistry physical chemistry division reporting physisorption data for gas/soils systems with special reference to the determination of surface area and porosity. Pure Appl Chem. 1985;57:603–619.
- Venkateswarlu K, Thakur H, Babu TNB. Fabrication of extended release tablets of pramipexole: in-vitro studies. Pharm Methods. 2017;8:115–120.
- Romero AA, Alba MD, Zhou W, et al. Synthesis and characterization of the mesoporous silicate molecular sieve MCM-48. J Phys Chem B. 1997;101:5294–5300.
- Takahashi R, Sato S, Sodesawa T, et al. High surface-area silica with controlled pore size prepared from nanocomposite of silica and citric acid. J Phys Chem B. 2000;104:12184–12191.
- Sartori C, Finch DS, Ralph B, et al. Determination of the cation content of alginate thin films by FTIR spectroscopy. Polymer 1997;38:43–51.
- Omwancha WS, Mallipeddi R, Valle BL, et al. Chitosan as a pore former in coated beads for colon specific drug delivery of 5-ASA. Int J Pharm. 2013;441:343–351.
- Lee JE, Lee N, Kim T, et al. Multifunctional mesoporous silica nanocomposite nanoparticles for theranostic applications. Acc Chem Res. 2011;44:893–902.
- Godin B, Gu J, Serda RE, et al. Tailoring the degradation kinetics of mesoporous silicon structures through PEGylation. J Biomed Mater Res. 2010;94:1236–1243.
- Jaganathan H, Godin B. Biocompatibility assessment of Si-based nano-and micro-particles. Adv Drug Deliv Rev. 2012;64:1800–1819.
- Aluani D, Tzankova V, Kondeva-Burdina M, et al. еvaluation of biocompatibility and antioxidant efficiency of chitosan-alginate nanoparticles loaded with quercetin. Int J Biol Macromol. 2017;103:771–782.
- Xu M, Feng C, Wang J, et al. In vitro heterogeneous degradation of alginate and its validation of different molecular weight on blood bio-compatibility. J Biomater Sci Polym Ed. 2017;28:380–393.
- Cassarino DS, Fall CP, Smith TS, et al. Pramipexole reduces reactive oxygen species production in vivo and in vitro and inhibits the mitochondrial permeability transition produced by the parkinsonian neurotoxin methylpyridinium ion. J Neurochem. 2002;71:295–301.
- Lu F, Wu SH, Hung Y, et al. Size effect on cell uptake in well-suspended, uniform mesoporous silica nanoparticles. Small. 2009;5:1408–1413.
- Mai Z, Chen J, Hu Y, et al. Novel functional mesoporous silica nanoparticles loaded with Vitamin E acetate as smart platforms for pH responsive delivery with high bioactivity. J Colloid Interface Sci. 2017;508:184–195.
- Ambati J, Lopez AM, Cochran D, et al. Engineered silica nanocarriers as a high-payload delivery vehicle for antioxidant enzymes. Acta Biomater. 2012;8:2096–2103.
- Raj R, Wairkar S, Sridhar V, et al. Pramipexole dihydrochloride loaded chitosan nanoparticles for nose to brain delivery: development, characterization and in vivo anti-Parkinson activity. Int J Biol Macromol. 2018;109:27–35.