Abstract
Coilia nasus is a rare anadromous fish species found in China’s Yangtze River and is a precious commercial commodity. The anti-stress mechanism of C. nasus has become the focus of research due to the animal’s strong stress responses. Salinity has been reported to weaken the C. nasus stress response. Nrf2 is an important anti-stress transcription factor that plays key regulatory roles in mediating salinity stress. In the present study, we cloned the full-length sequence of C. nasus Nrf2 for the first time and evaluated its differential expression in tissues. We also investigated the regulatory role of Nrf2 in osmoregulation, antioxidation and immunopotentiation in C. nasus under salinity stress. The results indicate that the C. nasus Nrf2 sequence is highly conserved. C. nasus Nrf2 is activated and plays a coordinated osmoregulatory role together with AQP1 under salinity stress and stimulates the activity of downstream antioxidant enzymes, including superoxide dismutase, glutathione peroxidase and so on. Nrf2 also triggers immunopotentiation via stimulation of lysozyme activity and an elevation of white blood cell counts. All of these C. nasus constructed adaptive Nrf2 regulatory networks act in response to salinity stress. This study lays a theoretical foundation for further research on the anti-stress mechanisms of C. nasus and will provide a reference for the further optimization of the C. nasus breeding mode.
Introduction
Coilia nasus is an important anadromous fish species that inhabits the Yangtze River of China. Moreover, it is valuable for artificial aquaculture. However, its strong response to stress has become a bottleneck for breeding research and is widely a cause of concern [Citation1–3].
Xu et al. [Citation4] reported that sodium chloride can weaken the C. nasus stress response and significantly increases its survival rate. Elevated salinity is also necessary for the entire course of the C. nasus breeding process. Therefore, it is beneficial to explore the C. nasus osmoregulatory mechanisms. Extensive studies have shown that antioxidant systems can regulate the anti-stress response [Citation5–7]. Nuclear factor erythroid 2-related factor (Nrf2) is a widely distributed transcription factor that plays a key role in antioxidant response [Citation8]. Under normal conditions, Nrf2 is maintained in an inhibited state by binding to Kelch-like ECH-associated protein (Keap1). In stressful conditions, Nrf2 is activated, detaches from Keap1, and translocates to the nucleus to activate transcription of Phase II enzymes including superoxide dismutase (SOD), catalase (CAT) and glutathione peroxidase (GPx) [Citation6,Citation9]. Abrupt salinity stress has been shown to induce an anti-oxidant response via the Nrf2-Keap1 pathway in the large yellow croaker (Pseudosciaena crocea) [Citation10]. Salinity can also enhance fish immunity by stimulating the non-specific immune system and influencing serum lysozyme activity, white blood cell counts, and other factors [Citation11,Citation12].
Extensive studies on the Nrf2 regulatory mechanisms have been performed in humans and other mammals [Citation13,Citation14], whereas very little research related to Nrf2 has been carried out on fish. Prior studies have focused on molecular cloning and differential expression of Nrf2 in grass carp (Ctenopharyngodon idellus) [Citation15] and zebrafish (Danio rerio) [Citation16], the effect of environmental factors on the antioxidant activity of yellow crocker (Pseudosciaena crocea) [Citation10] and the effect of feed nutrition on the antioxidant status of Jian carp (Cyprinus carpio var. Jian) [Citation17], and the detoxification activity of Nrf2 on fish [Citation18]. No Nrf2 research has been performed in C. nasus. A study on the C. nasus osmoregulatory mechanisms indicated that Aquaporin one adopts differential regulation modes in key osmoregulatory tissues to achieve homeostasis [Citation19]. We, for the first time, carried out molecular cloning and analyzed the differential expression of Nrf2 in C. nasus under salinity stress. We investigated the central regulatory role of Nrf2 in antioxidation, immunopotentiation and osmoregulation of C. nasus, in an effort to construct the regulatory network that manages salinity stress in C. nasus. This study will lay a theoretical foundation for further research on the C. nasus anti-stress mechanisms and provide a reference for further optimization of the breeding mode of C. nasus.
Materials and methods
Experimental fish and salinity stress
Experimental fish were five-month-old juvenile C. nasus whose average body length was (133.5 ± 0.9) cm and body weight was (8.78 ± 0.6) g. The fish were collected from Yixing, an experimental base of the Freshwater Fisheries Research Center of the Chinese Academy of Fishery Sciences. One month before the experiment, juvenile C. nasus were netted and cultured in six aquariums with circulating water systems whose volume was 1096 × 470 × 670 cm3. Each aquarium contained 15 fish. The aquariums were continuously aerated and water quality was monitored daily: the water temperature was (20 ± 0.8)°C, the pH was 7.3, and the concentration of dissolved oxygen was (8.3 ± 0.7) mg/L. The experimental fish were fed three times each day, at 7:00 A.M., 12:00 A.M. and 6:00 P.M. Feeding stopped one day before the stress experiment.
The control group and the salinity-stressed group (salinity level of 15‰ was achieved by adding sodium chloride) were established. Salinity was monitored by using a SALT6 salimeter (Eutech/Oakton, USA). Each group had three replicates, and each aquarium contained 15 fish. Fish were netted quickly and placed in 40 mg/L MS-222 for rapid infiltration anesthesia. Two fish were sampled from each aquarium at 0 h, 3 h, 6 h, 9 h and 12 h. Blood was collected from the caudal vein, then split into samples for centrifugation for serum preparation and measurement of other parameters. Gill, brain, heart, liver, spleen, intestine, kidney and muscle were collected and snap-frozen in liquid nitrogen, and then stored at −80 °C. The liver was also sampled for the measurement of enzyme activity.
Ethics statement
The study was approved by the Animal Care and Use Committee of Freshwater Fisheries Research Center at the Chinese Academy of Fishery Sciences. All the experiments were performed conforming to the Guide for the Care and Use of Laboratory Animals set by the Animal Care and Use Committee of the Freshwater Fisheries Research Center.
Cloning of Nrf2
Total liver RNA was extracted with the RNAiso reagent (Takara, Japan) following the manufacturer’s instructions. DNA was removed with recombinant DNase I (Takara, Japan). The concentration and quality of the extracted RNA was measured using a nucleic acid analyser (Eppendorf, Germany). An OD260/OD280 of 1.8–2.0 and 1% agarose gel electrophoresis was used to detect the RNA quality.
The degenerate primers P1 to P6 were designed according to the reported Nrf2 conserved sequence by Prime Premier 5 software (). cDNA was amplified with PrimeScript One Step reverse transcription polymerase chain reaction kit (RT-PCR Kit, Takara, Japan) and purified by Takara MiniBEST Agarose Gel DNA Extraction Kit (Takara, Japan). The fragments were ligated into the pMD18-T Vector (Takara, Japan), and transformed into Escherichia coli DH5α competent cells (Takara, Japan). Positive recombinants were selected and sequenced by the Shanghai Biosune Biotech Co. Ltd. (Shanghai, China). The obtained sequence was proved to be a homologous sequence of Nrf2 using NCBI blastx (https://blast.ncbi.nlm.nih.gov/Blast.cgi). Specific primers P7 to P8 for 5’- and 3’-end amplification were designed based on the obtained Nrf2 sequence of C. nasus (), and 5’- and 3’-end sequences were cloned by the method of rapid amplification of cDNA ends (RACE). The targeted fragment was purified and ligated into a vector and then transformed into E. coli DH5α competent cells and sequenced. The obtained intermediate fragment, 3’- end and 5’-end sequences were spliced by the ContigExpress software.
Table 1. Primers used in this study.
Analysis of nucleotide and deduced amino acids sequences
Nrf2 cDNA was translated into an amino acid sequence by the SMS online package(http://www.bio-soft.net/sms/index.html). The protein’s isoelectric point and molecular weight were analysed by the online program Isoelectric (http://isoelectric.org/). The secondary structure of the amino acid sequence was analysed by SOPMA (https://npsa-prabi.ibcp.fr/cgi-bin/npsa_automat.pl?page=/NPSA/npsa_sopma.html). The transmembrane structure domain was determined by the TMPRED program (https://web.expasy.org/protscale/), and functional domains were identified by PROSITE (https://prosite.expasy.org/), the amino acid sequence was aligned by MegAlign software.
Differential expression of Nrf2
The total RNA was extracted from tissues including the gill, brain, liver, heart, spleen, kidney, intestine and muscle under both normal conditions and salinity stress. β-Actin was used as the internal reference. P9 and P10 were used for β-actin amplification; specific primers P11 and P12 were designed to amplify the 185-bp Nrf2 fragment. The primers are shown in . Real-time PCR of Nrf2 was performed using the ABI 7500 Real-Time PCR System (ABI, USA) with SYBR Premix Ex Taq II (Takara, Japan). The procedure was as follows: 95 °C for 30 s for pre-denaturation, then 40 cycles of 95 °C for 5 s, 60 °C for 34 s and 72 °C for 50 s. Each sample was measured in triplicate, and the relative expression of Nrf2 was calculated by the 2-ΔΔCT method [Citation20].
Differential expression ofAQP1 under stress
Relative AQP1 expression levels in the gill, kidney, intestine and brain in the control and salinity-stressed groups were determined as described above for Nrf2.
Measurement of antioxidant enzymes and immune parameters under stress
Serum lysozyme activity was measured according to the method described by Subbotkina and Subbotkin [Citation21], and the test kit was purchased from Nanjing Jiancheng Bioengineering Institute of China. White blood cell (WBC) counts and red blood cell (RBC) counts were measured in a Mindray BC-5300 Hematology Analyzer (Mindray, China). The liver was weighed and ground at a ratio of 1 part liver to 9 parts pre-cooled saline solution, and then centrifuged for 15 min at 1789 g. The supernatant was used to determine the activities of SOD, CAT and GPx. Statistical analysis was done using the SPSS 21 software. Data are shown as mean values with (Standard error of the mean) (± SEM).
p < 0.05 was considered to be a significant difference.
Statistical analysis
One-way analysis of variance (ANOVA) followed by Duncan’s multiple range test was used for analyzing differences between all treatments (p < 0.05). Analysis was performed using the SPSS 21 software for Windows. The results are shown as (Mean ± SE), p < 0.05 was considered to be a significant difference.
Results and discussion
Sequence analysis and the functional domain of Nrf2
One 2486-bp full-length cDNA of Nrf2 was cloned, containing a 1,827-bp open reading frame (ORF). According to the deduced amino acid sequence, the ORF encodes a 607 amino acid protein with a 67.3 kDa molecular weight and an isoelectric point of 4.44 (GenBank No. KY652086). The deduced amino acid sequence contained 61 positively charged amino acid residues (Arg + Lys + His) and 97 negatively charged residues (Asp + Glu). The amino acid sequence does not contain a predicted transmembrane domain. As shown in , the C. nasus Nrf2 protein contains six conserved Neh domains (Neh1-Neh6) and a typical leucine zipper domain (bZIP) of the Cap-n-Collar family. Furthermore, the Nrf2 sequence also contained four N-glycosylation sites which were NETP, NNSP, NMSS and NSSG. The secondary structure was predicted to be 36.24% α-helical, 11.53% extended strand, 4.45% β-turn and 47.78% random coil.
Figure 1. Multiple protein sequence alignment of C. nasus Nrf2 in comparison with sequences from other relevant species. Amino acid alignment (A) and basic structure (B) of Nrf2.
Note: Completely identical amino acid residues are shown in shades. Four glycosylation sites are underlined; six conserved Neh domains are marked with horizontal lines above them; conserved sequences of DLG and ETGE are marked with arc; bZIP domain is marked in a box. The labels on the left are sequence names, the first two letters stand for species names, as follows, Cn, C. nasus; Cl, Clupea harengus; Ci, Ctenopharyngodon idella; Ss, Salmo salar; Cc, Cyprinus. carpio 'Jian'; Ip, Ictalurus punctatus; On, Oreochromis niloticus; Lc, Larimichthy crocea; Dr, Danio rerio; Hs, Homo sapiens; Mm, Mus musculus.
The six conserved Neh domains (Neh1-Neh6) predicted in C. nasus Nrf2 () are similar to other reported Nrf2 sequences. The Neh1 domain has one typical bZIP domain of the Cap-n-Collar family that can recognize and bind to the DNA of the anti-oxidant responsive element (ARE), and then activate the transcription of the downstream Phase II antioxidant genes [Citation22]. Neh2 has two basic sequences, DLG and ETGE, that are located at the N-terminus and bind to Keap1 and negatively regulate it [Citation9]. Neh4 and Neh5 activate the transcription of Nrf2 via binding to a co-activator. The Neh6 domain is serine-rich and regulates Keap1-independent degradation of Nrf2 [Citation22,Citation23]. All of these structural domains indicate a conserved function of C. nasus Nrf2 in regulating anti-oxidant pathways.
Osmoregulation of Nrf2 and AQP1 in C. nasus under salinity stress
As shown in , C. nasus Nrf2 was expressed in all detected tissues under normal conditions. It was expressed at the highest levels in the liver, followed by the brain and kidney; furthermore, the expression in these three tissues was significantly higher than in the other analysed tissues. The lowest expression was in the muscle. The expression levels in the various tissues were as follows: liver > brain > kidney > intestine > gill > spleen > heart > muscle. As shown in , the expression of Nrf2 in the gill and brain were significantly upregulated under salinity stress at 3 h (p < 0.05), whereas the expression in the kidney and intestine also increased but not significantly (p > 0.05). The Nrf2 expression in the four tissues was significantly upregulated at 6 h (p < 0.05). The expression levels of Nrf2 mRNA fluctuated in the four tissues at 9 h and 12 h, but they were all significantly upregulated compared with the control group (p < 0.05).
Figure 2. Relative Expression of Nrf2 in different C. nasus tissues.
Note: The uppercase letters indicate the tissue type: G, gill; B, brain; S, spleen; L, liver; H, heart; K, kidney; I, intestine; M, muscle. Different lowercase letters indicate significant differences (P<0.05).
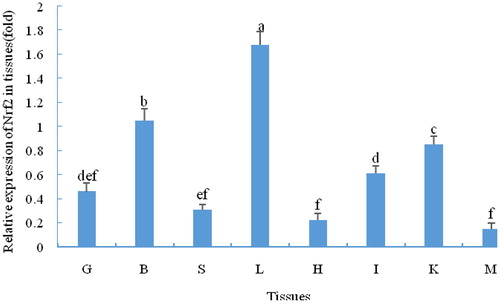
Figure 3. Differential expression of Nrf2 in four osmoregulatory tissues of C.nasus under salinity stress.
Note: BC, BE, GC, GE, KC, KE, IC, IE indicate control group (C) and the experimental groups (E) of the brain (B), gill (G), kidney (K) and intestine (I), respectively. Asterisks refer to significant differences at the same time point between the control and the experimental group (P<0.05).
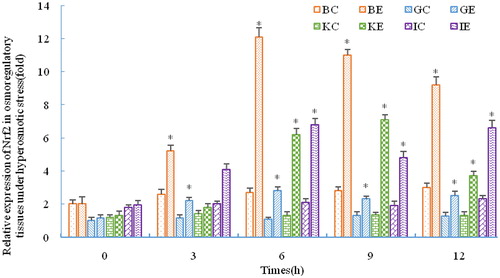
Nrf2 is a key transcription factor in the anti-oxidant system. In this study, Nrf2 was widely distributed in many tissues and was highly expressed in the brain, kidney, intestine and gill, which are in frequent contact with the external environment and metabolize vigorously [Citation24]. Similar results have been found for grass carp (Ctenopharyngodon idellus) [Citation15]. For euryhaline fish, the gill, kidney and intestine are recognized as major osmoregulatory tissues responsible for maintaining osmotic equilibrium [Citation25–27]. Furthermore, the brain has also been reported to play an important role in osmoregulation [Citation28–30]. In the present study, upregulation of Nrf2 in four C. nasus tissues also indicated an important osmoregulatory role under salinity stress. Furthermore, Nrf2 was upregulated earlier in the brain and gill than in the other two tissues. The reason for that may be that the gill is directly exposed to the environment and because of the central regulatory role of the brain under stress [Citation12].
As shown in , the expression of AQP1 mRNA in the gill significantly decreased, while it increased in the brain, kidney and intestine under stress (p < 0.05). The expression level in the gill was still significantly decreased at 9 h compared with the control group (p < 0.05), and then increased at 12 h but not significantly (p > 0.05). During salinity stress, the expression of AQP1 mRNA in the brain, kidney and intestine were all significantly upregulated compared with the pre-stress levels (p < 0.05).
Figure 4. Differential expression of AQP1 in four osmoregulatory tissues of C. nasustissues under salinity stress.
Note: BC, BE, GC, GE, KC, KE, IC, IE indicate control group (C) and the experimental groups (E) of the brain (B), gill (G), kidney (K) and intestine (I), respectively. Asterisks refer to significant differences at the same time point between the control and the experimental group (P<0.05).
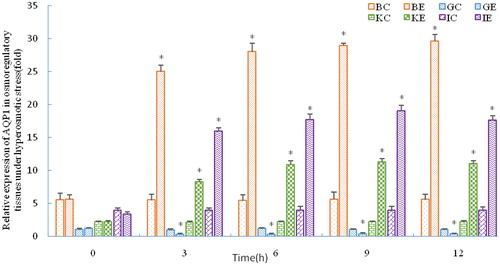
AQP1 is an integral membrane protein that facilitates transmembrane transport of water and other small molecules. Many relevant studies that have been carried out on Atlantic salmon (Salmo salar), sea bream (Dicentrarchus labrax), and other animals have revealed the key function of AQP1 in osmoregulation and maintaining homeostasis [Citation31–34]. There are no relevant studies regarding the synergetic effect of Nrf2 and AQP1 under salinity stress. Based on the results from the present study, we speculate that AQP1 executed differential regulation on four osmoregulatory tissues. Expression in the gill was downregulated, while the other tissues had similar AQP1 regulatory patterns. The branchial epithelium is at risk of high-flux water flow caused by the large osmotic gradient due to direct contact with the surrounding medium. Osmotic regulation is an adaptation pattern for organisms to choose to maintain osmotic balance by keeping the water permeability of the branchial epithelium at a lower limit. This may be the reason for the decreased AQP1 expression observed in the gill of many fish species under salinity stress [Citation31,Citation32,Citation35,Citation36]. Considering the significant upregulation of C. nasus Nrf2, these data suggest a potent protective function against salinity stress driven by synergistic activity of Nrf2 and AQP1.
Antioxidant regulation and immunopotentiation of C. nasus Nrf2 under salinity stress
As shown in , SOD activity in the liver was significantly increased at 3 h under stress (p < 0.05), and it was still increased at 12 h (p < 0.05). GPx activity kept increasing significantly during the period of stress (p < 0.05), and its expression pattern was similar to SOD. However, CAT expression was different from SOD and GPx: it increased and fluctuated during stress but not significantly (p > 0.05). As shown in , the serum lysozyme activity was increased at 3 h but not significantly (p > 0.05), upregulated at 6 h (p < 0.05), and continuously increased during stress. The RBC counts decreased at 12 h and were significantly decreased at 6 h (p < 0.05). However, WBC counts significantly increased during stress (p < 0.05).
Figure 5. Activity of antioxidant enzymes in the C. nasus liver under salinity stress.
Note: C, E indicate control group and experimental group, respectively. Asterisks refer to significant differences at the same time point between the control and the experimental group (P<0.05).
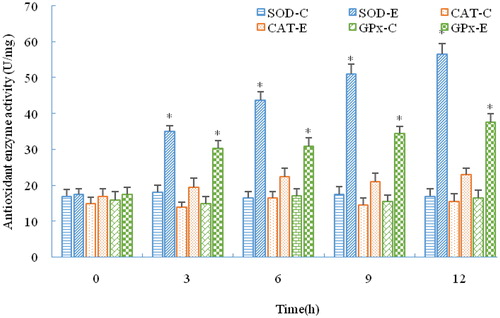
Figure 6. Immune parameters of C. nasus under salinity stress. RBC counts (A); WBC counts (B); Serum lysozyme activity (C).
Note: Asterisks refer to significant differences at the same time point between the control and the experimental group (P < 0.05).
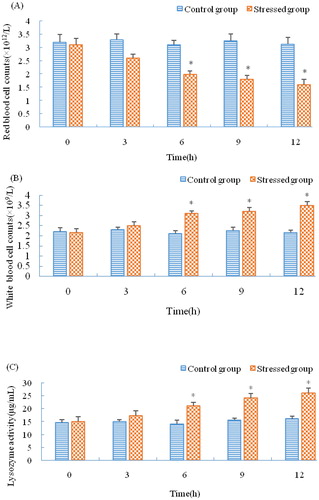
Salinity is an important environmental factor for aquatic organisms. Fish will produce more reactive oxygen species (ROS) under changing salinity. These ROS oxidize protein and unsaturated fatty acids, thereby causing damage. Organisms have evolved a unique antioxidant defence system to protect cells by limiting ROS concentrations to a relatively low level [Citation37–39]. This antioxidant defence system consists of both enzymes and non-enzymatic antioxidant molecules. The former include SOD, CAT and GPx [Citation40]. The latter include ascorbic acid, carotenoids and others. GPx and CAT are often induced concomitantly with the activation of SOD to increase antioxidant activity [Citation41]. The activity of CAT was not significantly upregulated in this study. The reason for that may be because GPx and CAT have similar functions, thus the antioxidant capacity of CAT is compensated for by an elevation in the activity of GPx [Citation42]. In this study, the regulation pattern of C. nasus Nrf2 was similar to that of SOD; this reflects the positive regulation of Nrf2 as an upstream transcription factor in the antioxidant pathway [Citation43,Citation44].
Salinity can also activate the fish non-specific immune system and trigger coordinated responses of the immune and osmoregulatory systems to variations in environmental factors [Citation45]. Lysozyme is widely distributed in fish mucus, serum and some lymphoid tissues. It participates in immune regulation and functions as one of the most effective agents against microbial invasion. Research regarding salinity stress of Takifugu obscurus indicated that salinity caused a change in serum lysozyme activity. In this study, the lysozyme activity in C. nasus was significantly upregulated, which is consistent with research on Takifugu obscurus [Citation46]. It was reported that fish with a faster swimming speed had more RBC than slower ones [Citation4,Citation47]. Xu et al. [Citation4] reported that sodium chloride will weaken the strong stress response of C. nasus. In this study, it was also observed that C. nasus swam gently and had decreasing RBC counts under salinity stress. The reason for these phenomena may be due to more of the animal’s energy supply being used for osmoregulation and energy consumption being reduced by decreasing RBC. The WBC is an important part of the non-specific immune system, and a change in WBC counts can influence fish immunity [Citation48]. In the present study, significant upregulation of WBC counts implicated the immunopotentiation of C. nasus in response to salinity stress. Research studies of Nrf2 on mice and human beings indicated that Nrf2 also played a vital role in protection against inflammation [Citation49–51]. Likewise, in this study, the C. nasus Nrf2-mediated antioxidant pathway was activated and further stimulated the upregulation of immune enzymes and molecules, indicating the regulatory role that Nrf2 plays in immunopotentiation in response to salinity stress ().
Figure 7. Nrf2-mediated adaptive regulatory network of C. nasus under salinity stress. Nrf2 was activated and detached from Keap1 under salinity stress, then Phase II antioxidant enzymes including SOD, GPx and CAT were activated by Nrf2. Together with the immune system (RBC, WBC, lysozyme) and the osmoregulatory factor AQP1. Nrf2 and these enzymes had a synergistic effect on the adaptive response of C. nasus under salinity stress.
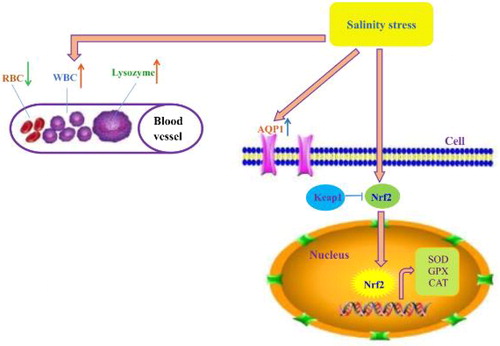
Conclusion
In summary, we, for the first time, cloned the full-length sequence of C. nasus Nrf2, analyzed its differential expression in tissues, and showed evidence of a regulatory role for Nrf2 in osmoregulation, antioxidation and immunopotentiation of C. nasus under salinity stress. This study revealed that the C. nasus Nrf2 sequence was highly conserved. Nrf2 was activated and executed a coordinated osmoregulatory role under salinity stress together with AQP1, and elevated the activity of downstream antioxidant enzymes including SOD and GPx. Meanwhile, Nrf2 also stimulated immunopotentiation via causing an elevation of lysozyme activity and WBC counts. All of these constructed adaptive Nrf2 regulatory networks act in response to salinity stress in C. nasus.
Abbreviations | ||
Nrf2 | = | Nuclear factor erythroid 2(NF-E2)-related factor |
Keap1 | = | Kelch-like ECH-associated protein |
SOD | = | superoxide dismutase |
CAT | = | catalase |
GPx | = | glutathione peroxidase |
bZIP | = | basic leucine zipper domain |
RBC | = | red blood cells |
WBC | = | white blood cells |
ORF | = | open reading frame |
ARE | = | anti-oxidant responsive element |
AQP1 | = | Aquaporin1 |
ROS | = | reactive oxygen species |
Disclosure statement
The authors declare that there is no conflict of interest.
Additional information
Funding
References
- Xu JB, Deng PP, Shi YH, et al. Effect of salinity on the survival and growth of the larvae and juveniles of Japanese grenadier anchovy Coilia nasus. N Am J Aquacult. 2016;78(1):1–7.
- Du FK, Xu GC, Gao JW, et al. Transport-induced changes in hypothalamic-pituitary-interrenal axis gene expression and oxidative stress responses in Coilia nasus. Aquac Res. 2016;4:3599–3607.
- Wang MY, Xu DP, Liu K, et al. Molecular cloning and expression analysis on LPL of Coilia nasus. Gene. 2016;39:66–72.
- Xu GC, Du FK, Nie ZJ, et al. Effects of 10‰ salinity to the plasma osmotic pressure, cortisol, glucose and liver glycogen in Colilia nasus stressed during loading and transportation. Acta Hydrobiol Sin. 2015;39:66–72.
- Giuliani ME, Benedetti M, Nigro M, et al. Nrf2 and regulation of the antioxidant system in the Antarctic silverfish, Pleuragramma antarctica: adaptation to environmental changes of pro-oxidant pressure. Mar Environ Res. 2017;129:1–13.
- Baysoy E, Atli G, Gurler CO, et al. The effects of increased freshwater salinity in the biodisponibility of metals (Cr, Pb) and effects on antioxidant systems of Oreochromis niloticus. Ecotox Environ Safe. 2012;84:249–253.
- Yin F, Peng SM, Sun P, et al. Effects of low salinity on antioxidant enzymes activities in kidney and muscle of juvenile silver pomfret Pampus argenteus. Acta Ecol Sin. 2011;31(1):55–60.
- Richardson BG, Jain AD, Speltz TE, et al. Non-electrophilic modulators of the canonical Keap1/Nrf2 pathway. Bioorg Med Chem Lett. 2015;25(11):2261–2268.
- Niture SK, Khatri R, Jaiswal AK. Regulation of Nrf2-an update. Free Radical Bio Med. 2014;66:36–44.
- Zeng L, Ai CX, Wang YH, et al. Abrupt salinity stress induces oxidative stress via the Nrf2-Keap1 signaling pathway in large yellow croaker Pseudosciaena crocea. Fish Physiol Biochem. 2017;43(4):955–910.
- Schmitz M, Ziv T, Admon A, et al. Salinity stress, enhancing basal and induced immune responses in striped catfish Pangasianodon hypophthalmus, (Sauvage). J Proteomics. 2017;167:12–24.
- Gu J, Dai S, Liu HT, et al. Identification of immune-related genes in gill cells of Japanese eels (Anguilla japonica) in adaptation to water salinity changes. Fish Shellfish Immunol. 2018;73:288–296.
- Ding H, Wang XL, Wang HD, et al. Nrf2-ARE signaling provides neuroprotection in traumatic brain injury via modulation of the ubiquitin proteasome system. Neurochem Int. 2017;111:32–34.
- Izumi Y, Kataoka H, Inose Y, et al. Neuroprotective effect of an Nrf2-ARE activator identified from a chemical library on dopaminergic neurons. Eur J Pharmacol. 2018;818:470–479.
- Feng Y, Qin ZD, Dai YJ, et al. Cloning and analysis of nuclear factor E2-related factor 2 (Nrf2) and its function in the regulation of respiratory burst in grass carp (Ctenopharyngodon idella). J Fish China. 2017;42:161–177.
- Timme-Laragy AR, Karchner SI, Franks DG, et al. Nrf2b, novel zebrafish paralog of oxidant-responsive transcription factor NF-E2-related factor 2 (NRF2). J Biol Chem. 2012;287(7):4609–4627.
- Zha J, Feng L, Liu Y, et al. Effect of dietary isoleucine on the immunity, antioxidant status, tight junctions and microflora in the intestine of juvenile Jian carp (Cyprinus carpio var. Jian). Fish Shellfish Immunol. 2014;41:663–673.
- Mukhopadhyay D, Priya P, Chattopadhyay A. Sodium fluoride affects zebrafish behaviour and alters mRNA expressions of biomarker genes in the brain: role of Nrf2/Keap1. Environ Toxicol Pharmacol. 2015;40(2):352–359.
- Wang MY, Yang J, Xu P, et al. Molecular cloning and expression analysis of aquaporin-1 from the Coilia nasus under high-salinity conditions(in Chinese). J Fishery Sci China. 2017;24(3):449–458.
- Livak KJ, Schmittgen TD. Analysis of relative gene expression data using real-time quantitative PCR and the 2-ΔΔCT method. Methods. 2001;25(4):402–408.
- Subbotkina TA, Subbotkin MF. Lysozyme content in organs and blood serum in various species in the Volga River. J Evol Biochem Physiol. 2003;39(5):537–546.
- Keum YS. Regulation of Nrf2-mediated phase II detoxification and anti-oxidant genes. Biomol Ther. 2012;20(2):144–151.
- Stefanson AL, Bakovic M. Dietary regulation of Keap1/Nrf2/ARE pathway: focus on plant-derived compounds and trace minerals. Nutrients. 2014;6(9):3777–3801.
- Furné M, García-Gallego M, Hidalgo MC, et al. Effect of starvation and refeeding on digestive enzyme activities in sturgeon (Acipenser naccarii) and trout (Oncorhynchus mykiss). Comp Biochem Phys A. 2008;149(4):420–425.
- Evans DH, Piermarini PM, Choe KP. The multifunctional fish gill: dominant site of gas exchange, osmoregulation, acid-base regulation, and excretion of nitrogenous waste. Physio Rev. 2005;85(1):97–177.
- Zou HF, He F, Lan ZH, et al. The personality of Japanese flounder (Paralichthys olivaceus) and gene expression related with osmoregulatory capacity in the gills. Aquaculture. 2019;500:221–227.
- Laverty G, Skadhauge E. Adaptation of teleosts to very high salinity. Comp Biochem Physiol, Part A Mol Integr Physiol. 2012;163(1):1–6.
- Weng CF, Chiang CC, Gong HY, et al. Bioenergetics of adaptation to a salinity transition in euryhaline teleost (Oreochromis mossambicus) brain. Exp Biol Med (Maywood). 2002;227(1):45–50.
- Delorenzi A, Dimant B, Frenkel L, et al. High environmental salinity induces memory enhancement and increases levels of brain angiotensin-like peptides in the crab Chasmagnathus granulatus. J Exp Biol. 2000;203(Pt 22):3369–3379.
- Sun DF, Lv JJ, Gao BQ, et al. Crustacean hyperglycemic hormone of Portunus trituberculatus: evidence of alternative splicing and potential roles in osmoregulation. Cell Stress Chaperons. 2019;24:517–525.
- Tipsmark CK, Sørensen KJ, Madsen SS. Aquaporin expression dynamics in osmoregulatory tissues of Atlantic salmon during smoltification and seawater acclimation. J Exp Bio. 2010;213(3):368–379.
- Giffard I, Boulo V, Aujoulat F, et al. Aquaporin molecular characterization in the sea-bass (Dicentrarchus labrax): the effect of salinity on AQP1 and AQP3 expression. Comp Biochem Physiol A Mol Integr Physiol. 2007;148:430–444.
- Aoki M, Kaneko T, Katoh F, et al. Intestinal water absorption through aquaporin 1 expressed in the apical membrane of mucosal epithelial cells in seawater-adapted Japanese eel. J Exp Biol. 2003;206(19):3495–3505.
- Sebastian W, Sukumaran S, Zacharia PU, et al. Isolation and characterization of aquaporin 1 (AQP1), sodium/potassium-transporting ATPase subunit alpha-1 (Na/K-ATPase α1), heat shock protein 90 (HSP90), heat shock cognate 71 (HSC71), osmotic stress transcription factor 1 (OSTF1) and transcription factor II B (TFIIB) genes from a euryhaline fish, Etroplus suratensis. Mol Biol Rep. 2018;45:2783–2789.
- Hill WG, Mathai JC, Gensure RH, et al. Permeabilities of teleost and elasmobranch gill apical membranes: evidence that lipid bilayers alone do not account for barrier function. Am J Physiol Cell Physiol. 2004;287(1):C235–C242.
- Choe KP, Evans DH. Compensation for hypercapnia by a euryhaline elasmobranch: effect of salinity and roles of gills and kidneys in fresh water. J Exp Zool. 2003;297A(1):52–63.
- Paital B, Chainy GB. Antioxidant defenses and oxidative stress parameters in tissues of mud crab (Scylla serrata) with reference to changing salinity. Comp Biochem Phys C. 2010;151:142–151.
- Choi CY, An KW, An MI. Molecular characterization and mRNA expression of glutathione peroxidase and glutathione S-transferase during osmotic stress in olive flounder (Paralichthys olivaceus). Comp Biochem Phys A. 2008;149(3):330–337.
- Aliko V, Qirjo M, Sula E, et al. Antioxidant defense system, immune response and erythron profile modulation in gold fish, Carassius auratus, after acute manganese treatment. Fish Shellfish Immun. 2018;76:101–109.
- Adeyemi JA. Oxidative stress and antioxidant enzymes activities in the African catfish, Clarias gariepinus, experimentally challenged with Escherichia coli and Vibrio fischeri. Fish Physiol Biochem. 2014;40(2):347–354.
- Giulio RT, Washburn PC, Wenning RJ, et al. Biochemical responses in aquatic animals: a review of determinants of oxidative stress. Environ Toxicol Chem. 2010;8:1103–1123.
- Kang SW, Rhee SG, Chang TS, et al. 2-Cys peroxiredoxin function in intracellular signal transduction: therapeutic implications. Trends Mol Med. 2005;11(12):571–578.
- Lee JM, Johnson JA. An important role of Nrf2-ARE pathway in the cellular defense mechanism. J. Biochem. Mol. Biol. 2004;37(2):139–143.
- Xu J, Feng L, Jiang WD, et al. Different dietary protein levels affect flesh quality, fatty acids and alter gene expression of Nrf2-mediated antioxidant enzymes in the muscle of grass carp (Ctenopharyngodon idella). Aquaculture. 2018;493:272–282.
- Burg MB, Ferraris JD, Dmitrieva NI. Cellular response to hyperosmotic stresses. Physiol Rev. 2007;87(4):1441–1474.
- Bian PJ, Qiu CG, Xu S, et al. Effects of salinity on growth, activity of non-specific immune and antioxidant enzymes in obscure puffer Takifugu obscures. Acta Hydrobiol Sin. 2014;38:108–114.
- Danilo WF, Gunther JE, Gilson K. Comparative hematology in marine fish. Comp Biochem Physiol. 1992;102:311–321.
- Ibrahem MD, Fathi M, Mesalhy S, et al. Effect of dietary supplementation of inulin and vitamin C on the growth, hematology, innate immunity, and resistance of Nile tilapia (Oreochromis niloticus). Fish Shellfish Immunol. 2010;29(2):241–246.
- Maruyama A, Itoh K. The role of Nrf2 in the protection against inflammation and innate immunity. Hirosaki Med J. 2007;59:S167–S171.
- Li H, Shi YY, Wang XL, et al. Piceatannol alleviates inflammation and oxidative stress via modulation of the Nrf2/HO-1 and NF-kappaB pathways in diabetic cardiomyopathy. Chem-Biol Interact. 2019;310:108754.
- Zhang X, Lai W, Ying X, et al. Salidroside reduces inflammation and brain injury after permanent middle cerebral artery occlusion in rats by regulating PI3K/PKB/Nrf2/NF kappaB signaling rather than complement C3 activity. Inflammation. 2019;42(5):1830–1842.