Abstract
S-adenosylmethionine (SAM) is an important compound in living organisms and has a number of different roles, such as polyamine synthesis. It can also be used for the treatment of diseases. Gene targeting with homologous recombination in Komagataella phaffii (K. phaffii) created DAS1 and DAS2 gene knockout strains, which use the methanol pathway to generate more ATP and increase SAM production. These strains showed an increase in the flux of methanol oxidation and an increase in ATP production. The gene knockout retarded the cell growth in the late phase of induction, did not influence the total methanol consumption or the activity of SAM synthetase, but did significantly increase the yield of SAM by 43.7%. The metabolic flux of methanol oxidation is increased by DAS1 and DAS2 gene knockout, which leads to an increase in ATP and higher SAM production.
Introduction
S-Adenosylmethionine (SAM), also known as AdoMet and SAMe, is a nothing-goes-to-waste molecule in all living organisms as the principal biological methyl donor, the precursor of aminopropyl groups utilized in polyamine synthesis, the precursor of glutathione (GSH) via the trans-sulfuration pathway [Citation1]. Abnormal SAM metabolism has been associated with liver diseases and in various neurological disorders, whilst SAM addition has been used to treat depression, osteoarthritis, fibromyalgia and Alzheimer’s diseases. Several strategies have been used to enhance SAM production, such as catalysis by SAM synthetase, biotransformation by resting microbial cells and fermentation by microbial species. For example, it has been produced by SAM synthetase catalysis of L-methionine and ATP at a 50 mmol/L scale [Citation2], and produced through immobilized recombinant E. coli that expresses SAM synthetase [Citation3]. SAM production, through the optimization of cultivation or gene co-expression [Citation4], has been reported in many recombinant microbial species, such as Saccharomyces cerevisiae [Citation5, Citation6], Pichia pastoris (a.k.a. Komagataella phaffii) [Citation7], Candida utilis [Citation8], Scheffersomyces stipitis, Kluyveromyces lactis, Kluyveromyces marxianus, Corynebacterium glutamicum [Citation9] and Escherichia coli [Citation10]. In recent years, Komagataella phaffii (K. phaffii), formerly known as Pichia pastoris, has been reported to be a promising host for high levels of SAM production [Citation11]. The advantages of K. phaffii, which have attracted the attention of researchers and engineers, are high cell density, low cost of the cultivation, a strong and inducible promoter and a well-established genetic modification protocol [Citation12]. Over 600 proteins have been successfully expressed in cells or excreted in fermentation broth from K. phaffii. The genes associated with these proteins are from various organisms, such as viruses, prokaryotes, eukaryotes and humans. This shows that K. phaffii can be adapted to a wide range of uses [Citation13]. Several heterologous proteins from recombinant K. phaffii have been approved by the United States Food and Drug Administration (FDA) [Citation14]. K. phaffii is also considered to be a well-known host for metabolites, such as carotene, nootkatone, polyhydroxyalkanoates and SAM, through metabolic engineering [Citation15]. Currently, SAM production in K. phaffii has been described using a number of fermentation strategies, such as maintenance of the level of the nitrogen source [Citation16], enhancement of the oxygen supply [Citation17], decrease in the energy maintenance coefficient [Citation7], interval carbon source switch [Citation18] and the expression of an auxiliary enzyme [Citation19]. As SAM is produced from endogenous ATP and exogenous methionine, ATP that is generated by the microbial species is the key factor for SAM production. Many researchers have reported fermentation strategies to improve ATP generation [Citation20]. During the K. phaffii induction phase, ATP arises from the catabolism pathway of methanol.
shows the pathway of methanol oxidation in K. phaffii that expresses SAM synthetase under alcohol oxidase 1 promotor (PAOX1). Methanol is oxidized by alcohol oxidase (AOX) to formaldehyde which is further metabolized in either the assimilatory or dissimilatory pathway. In the assimilatory pathway, formaldehyde reacts with D-xylulose-5-phosphate and is subsequently converted into dihydroxyacetone and D-glyceraldehyde-3-phosphate by dihydroxyacetone synthase (DAS), and eventually into cell components and metabolites. In the dissimilatory pathway, formaldehyde is oxidized to carbon dioxide, yielding two equivalents of nicotinamide adenine dinucleotide (NADH). In our previous study using the recombinant K. phaffii strain that expressed SAM synthetase under alcohol oxidase 1 promotor (PAOX1) [Citation17], SAM was generated from ATP of NADH oxidation, and L-methionine by SAM synthetase. Here, we presented that the DAS1 and DAS2 genes that encode DAS were knocked out to disrupt the assimilatory pathway and redirect the flux through the dissimilatory pathway. Thus, methanol should be exclusively oxidized to carbon dioxide, which increased ATP and SAM production.
Figure 1. Schematic diagram of SAM production by recombinant K. phaffii. AOX, alcohol oxidase; DAS1/2-dihydroxyacetone synthase; DHA, dihydroxyacetone; GAP, glyceraldehyde 3-phosphate; Xu-5P, xylulose 5-phosphate; FBP, fructose 1,6, bisphosphate; F6P, fructose 6–phosphate; SAM, S-adenosylmethionine.
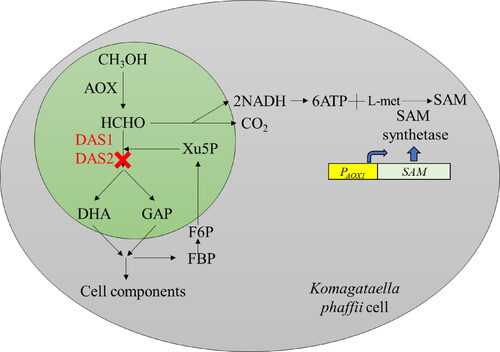
Materials and methods
A recombinant K. phaffii was constructed through a genetic protocol that had been reported in our previous work [Citation17]. We used a pPIC3.5K plasmid as a vector to express the SAM synthase gene in the K. phaffii GS115 strain (Invitrogen, Carlsbad, USA).
Yeast extract peptone dextrose medium (YPD) consisted of yeast extract (10 g/L), peptone (20 g/L), glucose (20 g/L) and agar powder (15 g/L) if necessary.
Basal salt medium (BSM) consisted of glycerol (40 g/L), calcium sulfate dihydrate (0.17 g/L), magnesium sulfate heptahydrate (2.32 g/L), potassium sulfate (2.86 g/L), phosphoric acid (aq. 85% 17 g/L), potassium hydroxide (2.56 g/L), pH adjusted to 5.0 with ammonia and addition of 4.35 mL Pichia trace metals (PTM1) after autoclaving.
PTM1 consisted of biotin (0.2 g/L), CuSO4 × 5 H2O (6 g/L), KI (93.33 mg/L), MnSO4 × H2O (3.067 g/L), Na2MoO4 × 2 H2O (0.2 g/L), H3BO3 (20 mg/L), COCl2 × 6 H2O (0.916 g/L), ZnSO4 × 7 H2O (42.2 g/L), FeSO4 × 7 H2O (65 g/L) and H2SO4 (3.75 mL/L).
Recombinant K. phaffii strain construction
Homologous recombination gene targeting technique [Citation21] was used to knockout the DAS gene to generate two recombinant strains K. phaffii SAMΔDAS1 and K. phaffii SAMΔDAS1/ΔDAS2. The knockout cassettes were constructed as described below. The primers used in this study are listed in . The 5′- (up) homologous arm of the DAS1 gene was amplified using primers DAS1-5UTR-F/DAS1-5UTR-R. And 3′- (down) homologous arm of the DAS1 gene was amplified using primers DAS1-3UTR-F/DAS1-3UTR-R. The two arms were amplified from the genomic DNA of K. phaffii GS115. These two homologous arms were then ligated by overlap extension PCR using primers DAS1-5UTR-F/DAS1-3UTR-R to obtain a fusion fragment. The plasmid pPICZ-DAS1 was generated by inserting a fusion fragment into the vector pPICZαA linearized with primers of Z-DAS1-F/Z-DAS1-R that harbor a ZeocinTM resistance marker by a ClonExpress II one step cloning kit (Vazyme). The pPICZ-DAS1 vector after Kpn I digestion was transferred by electroporation to K. phaffii competent cells [Citation17] to obtain the K. phaffii SAM ΔDAS1 strain. A similar process was performed to create a strain K. phaffii SAM ΔDAS1/ΔDAS2. The 5′- and 3′- fragments of the DAS1 and DAS2 genes were amplified by primers DAS1/2-5UTR-F/DAS1/2-5UTR-R, and DAS1/2-3UTR-F/DAS1/2-3UTR-R, respectively. Then ligated with the fragment of pPICZαA vector was prepared using the primers Z-DAS1/2-F/Z-DAS1/2-R, to obtain a recombinant pPICZ-DAS1/2 vector. The DAS1 gene knockout and DAS1/DAS2 gene knockout transformants were selected on YPD plates that contained 100 µg/mL ZeocinTM. The YPD plates were incubated at 30 °C for two days. The two gene knockout strains K. phaffii SAMΔDAS1 and K. phaffii SAMΔDAS1/ΔDAS2 were identified by PCR using the internal validation primers in-DAS1-F/in-DAS1-R. The molecular protocol was performed in accordance with Molecular Cloning [Citation22] and Pichia protocols [Citation21].
Table 1. Primers.
Recombinant K. phaffii cultivation
Recombinant K. phaffii strains were transferred from a 25% glycerol stock tube to 3 mL of YPD, and cultivated for three days at 30 °C and 200 r/min. The cultivation broth was then transferred into 50 mL of BSM medium in a 250 mL flask, with 5% inoculate ratio. The BSM medium was cultivated for two days at 30 °C and 200 r/min. Methanol was then added to a final concentration of 1% every 24 h. Samples were collected for analysis every 24 h, before methanol addition.
Determination of cell density
Cell density was measured using a spectrophotometer (U-1100, Hitachi Ltd., Tokyo, Japan), against distilled water, at 600 nm, after appropriate dilution. The dry cell weight (DCW) of a sample was obtained from the experimentally determined calibration curve between cell optical density and the DCW.
Determination of SAM yield
The yield of SAM was determined by mixing 0.5 mL of fermentation broth with 0.5 mL of 20% HClO4, The mixture was stored for 8 h and was then centrifuged at 15,777 g for 3 min. The supernatant (0.5 mL) was then harvested and ammonium hydroxide (0.5 mL of a 10% solution) was added for neutralization. The supernatant was filtrated using a Φ0.22 μm membrane. The SAM was analyzed by high performance liquid chromatography (Waters 2480, MA, USA), using a reverse phase C18 column (4.6 × 250 mm, 5 µm). The mobile phase was 0.01 mol/L ammonium formate (pH adjusted to 3.0 with formic acid).
Determination of SAM synthetase
Recombinant K. phaffii fermentation broth (2 mL) was collected by centrifugation at 10,956 g for 2 min and immediately washed twice with 0.1 mol/L PBS buffer (pH 7.5). The supernatant was discarded. The volume of the cell pellet was then estimated and three volumes of ice-cold lysis buffer (0.1 mol/L PBS pH 7.5, 1 mmol/L phenylmethanesulfonyl fluoride, 1 mmol/L EDTA, 5% [v/v] glycerol) were added. Chilled glass beads of the same total volume as the suspended K. phaffii cells were added. The suspension was vortexed for 30 s and this was repeated eight times. Finally, the cell debris was removed by centrifugation at 10,956 g for 5 min at 4 °C. The activity of SAM synthetase was measured in the supernatant, in accordance with the method of Shiozaki et al. [Citation23]. One unit of enzyme activity was defined as the amount of activity required to catalyze the transformation of 1 μmol of L-methionine into SAM, per minute, at 37 °C.
Determination of ATP concentration
After washing with deionized water, the K. phaffii cells (2 mL) were suspended in 0.5 mL of deionized water and 0.5 mL of 20% perchloric acid for 10 min. The pH of the suspension was adjusted to 3.0 using 1 mL of 1.3 mol/L KOH. Subsequently, the suspension was centrifuged (10,956 g for 1 min) and filtered through a Φ0.22 μm membrane to remove the potassium perchlorate, and the pH was further adjusted to 7.0 using 1 mL of 100 mmol/L PBS (pH 7.5). The obtained solution was stored at −20 °C until further analysis. High performance liquid chromatography (Waters 2480, MA, USA) was performed using a reverse phase C18 column (4.6 × 250 mm, 5 µm), with the stored solution and 100 mol/L PBS (pH 6.5), at a flow rate of 1.0 mL/min. The ATP concentration was calculated based on the peak areas at 254 nm.
Methanol analysis
Methanol is oxidized by potassium permanganate to generate formaldehyde, which forms a purple compound with chromotropic acid in sulfuric acid solution. This purple compound has an absorption peak at 574 nm. One milliliter of fermentation broth was centrifuged at 10,956 g for 5 min. And, 0.2 mL of 25% sulfuric acid and 0.1 mL of 1% potassium permanganate were added to 0.1 mL of the supernatant. This mixture was incubated for 5 min at room temperature and then mixed with 0.1 mL of 5% sodium sulfite solution and 1.0 mL of concentrated sulfuric acid, then added 0.1 mL of 0.5% chromotropic acid, mixed the solution by shaking and heated it in boiling water for 20 min. The volume was adjusted to 2 mL using water after cooling to room temperature. The methanol content was then calculated based on the standard curve of OD574 value.
Data statistical analysis
All experiments were carried out three times. The statistical analysis was performed using Microsoft Excel 2016 (Office, Microsoft, Seattle, USA). The standard deviation was calculated and was showed as the error bar.
Results and discussion
Construction of knockout strains
shows the results from the construction of two recombinant K. phaffii strains. The 3′- and 5′- untranslated regions (UTR) of the DAS1 gene were 1279 bp and 1537 bp, respectively. The 3′- and 5′- UTRs of DAS1/2 were 1124 bp and 1279 bp, respectively. The linearized vector was 1966 bp and 1965 bp. The recombinant vectors were transformed to create K. phaffii SAM ΔDAS1 and K. phaffii SAM ΔDAS1/ΔDAS2 strains (). shows the PCR results of the UTR fragments and overlapped fragments during K. phaffii SAM ΔDAS1 and K. phaffii SAM ΔDAS1/ΔDAS2 strain construction. shows the PCR results of the K. phaffii SAM △DAS1 and K. phaffii SAM ΔDAS1/ΔDAS2 strain. Using internally validated primers, the resulting product size was 482 bp (). The presence of non-DAS1 fragments in indicated that DAS1 and DAS1/DAS2 knockout was successful.
Figure 2. Schematic representation of experimental procedures for DAS gene knockout and identification. (A) Construction strategy of DAS1 gene disruption cassette. (B) Construction strategy of DAS1, DAS2 gene disruption cassette. M: DL5000 (Takara Biomedical Technology (Beijing) Co., Ltd), 1: DAS1 3 UTR, 2: DAS1 5 UTR, 3: DAS1/2 3 UTR, 4: DAS1/2 5 UTR, 5: DAS1 5 UTR- DAS1 3 UTR, 6: DAS1/2 5 UTR- DAS1/2 3 UTR, 7: Zeocin-ori fragment of pPICαA by PCR with Z-DAS1-F and z-DAS1-R, 8: Zeocin-ori fragment of pPICαA by PCR with Z-DAS1/2-F and Z-DAS1/2-R. (C) PCR results of K. phaffii transformants. M: DL2000 (Takara Biomedical Technology (Beijing) Co., Ltd), 1: positive control, 2: negative control, 3: K. phaffii SAM △DAS1, 4: K. phaffii SAM △DAS1/△DAS2.
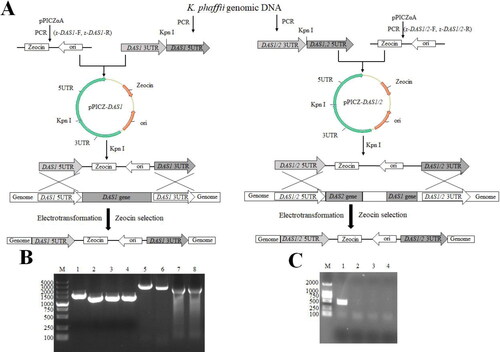
Growth curves and SAM production of recombinant K. phaffii strains
shows the recombinant K. phaffii growth curves, SAM yields, residual methanol and SAM synthetase activity. As shown in , the cell densities of K. phaffii SAM, K. phaffii SAM ΔDAS1 and K. phaffii SAM ΔDAS1/ΔDAS2 strains increased from approximately to about 6.4–6.5 g DCW/L after 72 h induction. The cell densities then remained stable in the K. phaffii SAM strain and decreased to 6.0 g DCW/L and 5.8 g DCW/L in the K. phaffii SAM ΔDAS1 and K. phaffii SAM ΔDAS1/ΔDAS2 strains, respectively. In this study, the K. phaffii SAM ΔDAS1 and K. phaffii SAM ΔDAS1/ΔDAS2 strains reduced the formaldehyde assimilatory pathway, which slowed cell component production from methanol. Therefore, a decrease in biomass was observed due to cell lysis during the late induction phase because of low building block supply through the formaldehyde assimilatory pathway. The phenomenon of cell density decreasing was reported by several researchers previously. Hohenblum et al. [Citation24] reported a decrease in the viability of K. phaffii significantly below 70% during the methanol fed batch phase. Liu et al. [Citation25] reported the cell lysis of K. phaffii, which was improved by methanol feed strategy modification and pure oxygen supply. Pure oxygen supply is not a safety method to be applied into industrial operation [Citation25].
Figure 3. SAM production by recombinant K. phaffii strains. (A) Curves of cell growth, (B) SAM production, (C) methanol concentration, (D) Activity of SAM synthetase.
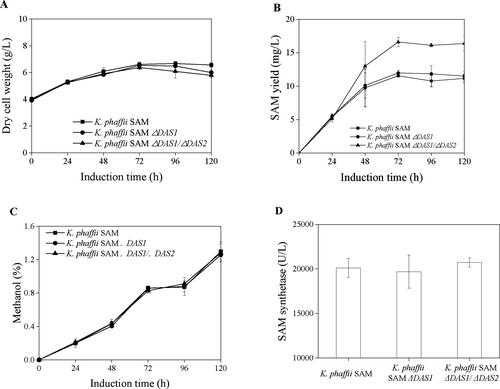
The overall profiles of SAM yield of K. phaffii SAM and K. phaffii SAM ΔDAS1 showed similar curves, in which SAM yields increased to 11.6 mg/L and 12.0 mg/L after 72 h induction, and decreased slightly at 120 h induction (). The SAM yield of K. phaffii SAM ΔDAS1/ΔDAS2 increased to 16.6 mg/L after 72 h induction, and remained stable until 120 h induction (). Therefore, no significant difference of SAM yield was observed between K. phaffii SAM and K. phaffii SAM ΔDAS1. An increase of 43.7% was observed at the condition of K. phaffii SAM ΔDAS1/ΔDAS2. The residual methanol concentrations of K. phaffii SAM, K. phaffii SAM ΔDAS1 and K. phaffii SAM ΔDAS1/ΔDAS2 showed the same trends (), which indicated non-significant difference of methanol consumption when the assimilatory pathway was reduced by DAS gene knockout. The SAM synthetase activities in these three K. phaffii strains did not show significant difference either (), which indicated that the increase in the SAM yield was attributed to the formaldehyde dissimilatory pathway. As ATP is a substrate for SAM production, the flux enhancement of the dissimilatory pathway is a direct and promising approach for higher SAM production [Citation11].
ATP analysis of recombinant K. phaffii strains
listed the parameters of recombinant K. phaffii strains after 72 h induction. The biomass of K. phaffii SAM ΔDAS1/ΔDAS2 was 6.4 g DCW/L and was not significantly different from that of the SAM strain. But the SAM yields in K. phaffii SAM ΔDAS1/ΔDAS2 increased to 16.6 mg/L, i.e. 43.7% increase compared to the SAM strain. The specific productivity of SAM increased from 0.082 mg/(DCW·g) to 0.086 mg/(DCW·g) and 0.12 mg/(DCW·g) for the K. phaffii SAM ΔDAS1 and K. phaffii SAM ΔDAS1/ΔDAS2 strains, respectively. This equates to a 1.46-fold increase caused by DAS1 and DAS2 gene knockout. shows that the ATP concentration increased from 0.36 mmol/L to 0.40 mmol/L and 0.46 mmol/L in the K. phaffii SAM ΔDAS1 and K. phaffii SAM ΔDAS1/ΔDAS2 strains, respectively. This suggests that DAS gene knockout redirected the methanol pathway toward ATP production and increased SAM production.
Table 2. Analysis of SAM production of recombinant K. phaffii strains.
Methanol is metabolized by K. phaffii in two ways: 1) methanol is oxidized to carbon dioxide and NADH is generated, which can enter the mitochondrial electron transport chain to produce ATP, 2) methanol is metabolized to produce dihydroxyacetone (DHA) and 3-phosphoglyceraldehyde (GAP) into the pentose phosphate pathway (PPP) and the tricarboxylic acid cycle (TCA) to produce amino acids and nucleotides that provide substances for cell growth and recombinant protein expression. S-adenosylmethionine is produced from ATP and l-methionine in recombinant K. phaffii strains. shows the ATP level in recombinant K. phaffii strains after 72 h induction. These results indicated an increased oxidization of methanol in these K. phaffii strains, to generate ATP for SAM production. Notably, cell growth was inhibited in DAS knockout strains during the later induction phase. In contrast, Geier et al. [Citation6] reported that the growth rate showed a slight decrease in the DAS1 knockout strain but the DAS1 and DAS2 double knockout strain showed a very low growth rate. However, this was determined under conditions where methanol was the sole carbon source and did not take into account the circumstance where glycerol is used for biomass enrichment. The reason for the inconsistencies may be that during the first 48 h of utilizing glycerol as a carbon source, a large amount of amino acids, nucleotides, lipids and other substrates for cell growth accumulate in the cell. When the carbon source is converted, methanol induces the AOX1 promoter but the accumulated substances in the cell would continue to work for cell growth and proliferation. Therefore, there is little difference in growth found between the strains but, after 96 h, the cell probably depletes these substances and the biomass decreases. Chen et al. [Citation26] over-expressed NADH kinase (Pos5p), NADH kinase-like enzymes (PntAB) and YfjB to increase NADH for SAM synthesis, with a resulting 70% increase. Chen et al. [Citation27] also repressed ATP-dependent by-products from related genes, proB, glnA and argB, to increase SAM production. A newly discovered ATP-sensing riboswitch ydaO motif was used to balance the requirement of ATP in SAM biosynthesis, with the result of a higher SAM titer (1.23 mg/L). The SAM titer was increased by approximately 55% when compared with the control [Citation28].
Conclusions
To enhance SAM production in recombinant K. phaffii, DAS1 and DAS2 genes were knocked out to block the catabolism pathway of methanol, increasing the flux of methanol oxidase to CO2. This approach benefited NADH (ATP) generation, and SAM production eventually in the recombinant K. phaffii harboring SAM synthetase at the condition of external L-methionine supply. The results showed a 43.7% enhancement of SAM yield, which indicated that more ATP was generated when the DAS gene was knocked out. This approach built a basis for SAM production further.
Author contributions
TL Carried out all the experiments, and collected all data. JZ and HZ provided the concept. JZ and BL wrote the manuscript. All authors polished the manuscript.
Acknowledgment
This work was supported by the National Natural Science Foundation of China (No. 31870045).
Disclosure statement
All authors declare they have no other competing interests.
References
- Fontecave M, Atta M, Mulliez E. S-adenosylmethionine: nothing goes to waste. Trends Biochem Sci. 2004;29(5):243–249.
- Niu W, Cao S, Yang M, et al. Enzymatic synthesis of S-adenosylmethionine using immobilized methionine adenosyltransferase variants on the 50-mM scale. Catalysts. 2017;7(8):238.
- Yin C, Zheng T, Chang X. Biosynthesis of S-adenosylmethionine by magnetically immobilized Escherichia coli cells highly expressing a methionine adenosyltransferase variant. Molecules. 2017;22(8):1365.
- Ravi Kant H, Balamurali M, Meenakshisundaram S. Enhancing precursors availability in Pichia pastoris for the overproduction of S-adenosyl-l-methionine employing molecular strategies with process tuning. J Biotechnol. 2014;188:112–121.
- Zhao W, Hang B, Zhu X, et al. Improving the productivity of S-adenosyl-l-methionine by metabolic engineering in an industrial Saccharomyces cerevisiae strain. J Biotechnol. 2016;236:64–70.
- Geier M, Brandner C, Strohmeier GA, et al. Engineering Pichia pastoris for improved NADH regeneration: a novel chassis strain for whole-cell catalysis. Beilstein J Org Chem. 2015;11:1741–1748.
- Zhang JG, Wang XD, Su EZ, et al. A new fermentation strategy for S-adenosylmethionine production in recombinant Pichia pastoris. Biochem Eng J. 2008;41(1):74–78.
- Wang D, Li D, Zhang G, et al. Disruption of por1 gene in Candida utilis improves co-production of S-adenosylmethionine and glutathione. J Biotechnol. 2019;290:16–23.
- Han G, Hu X, Wang X. Overexpression of methionine adenosyltransferase in Corynebacterium glutamicum for production of S-adenosyl-l-methionine. Biotechnol Appl Biochem. 2016;63(5):679–689.
- Chen H, Wang Z, Cai H, et al. Progress in the microbial production of S-adenosyl-l-methionine. World J Microbiol Biotechnol. 2016;32(9):153.
- Chu J, Qian J, Zhuang Y, et al. Progress in the research of S-adenosyl-l-methionine production. Appl Microbiol Biotechnol. 2013;97(1):41–49.
- Zhu T, Sun H, Wang M, et al. Pichia pastoris as a versatile cell factory for the production of industrial enzymes and chemicals: current status and future perspectives. Biotechnol J. 2019;14(6):13.
- Fischer J, Glieder A. Current advances in engineering tools for Pichia pastoris. Curr Opin Biotechnol. 2019;59:175–181.
- Vogl T, Hartner FS, Glieder A. New opportunities by synthetic biology for biopharmaceutical production in Pichia pastoris. Curr Opin Biotechnol. 2013;24(6):1094–1101.
- Pena D, Gasser B, Zanghellini J, et al. Metabolic engineering of Pichia pastoris. Metab Eng. 2018;50:2–15.
- Zhang JG, Wang XD, Zheng Y, et al. Enhancing yield of S-adenosylmethionine in Pichia pastoris by controlling NH4+ concentration. Bioprocess Biosyst Eng. 2008;31(2):63–67.
- Zhang JG, Wang XD, Zhang JN, et al. Oxygen vectors used for S-adenosylmethionine production in recombinant Pichia pastoris with sorbitol as supplemental carbon source. J Biosci Bioeng. 2008;105(4):335–340.
- Hu X, Chu J, Zhang S, et al. A novel feeding strategy during the production phase for enhancing the enzymatic synthesis of S-adenosyl-l-methionine by methylotrophic Pichia pastoris. Enzyme Microb Technol. 2007;40(4):669–674.
- Hu H, Qian J, Chu J, et al. Optimization of L-methionine feeding strategy for improving S-adenosyl-L-methionine production by methionine adenosyltransferase overexpressed Pichia pastoris. Appl Microbiol Biotechnol. 2009;83(6):1105–1114.
- Hara KY, Kondo A. ATP regulation in bioproduction. Microb Cell Fact. 2015;14(1):198
- Higgins DR, Cregg JM. Pichia protocols. Vol. 103. Totowa, New Jersey: Springer; 1998.
- Green MR, Sambrook J. Molecular cloning: A laboratory manual. 4th ed. New Jersey: Cold Spring Harbor Laboratory Press; 2012.
- Shiozaki S, Shimizu S, Yamada H. Unusual intracellular accumulation of S-Adenosyl-L-methionine by microorganisms. Agric Biol Chem. 1984;48(9):2293–2300.
- Hohenblum H, Borth N, Mattanovich D. Assessing viability and cell-associated product of recombinant protein producing Pichia pastoris with flow cytometry. J Biotechnol. 2003;102(3):281–290.
- Liu WC, Gong T, Wang QH, et al. Scaling-up Fermentation of Pichia pastoris to demonstration-scale using new methanol-feeding strategy and increased air pressure instead of pure oxygen supplement. Sci Rep. 2016;6(1):18439
- Chen Y, Xu D, Fan L, et al. Manipulating multi-system of NADPH regulation in Escherichia coli for enhanced S-adenosylmethionine production. RSC Adv. 2015;5(51):41103–41111.
- Chen Y, Lou S, Fan L, et al. Control of ATP concentration in Escherichia coli using synthetic small regulatory RNAs for enhanced S-adenosylmethionine production. FEMS Microbiol Lett. 2015;362(15):fnv115.
- Chen Y, Zhou H, Wang M, et al. Control of ATP concentration in Escherichia coli using an ATP-sensing riboswitch for enhanced S-adenosylmethionine production. RSC Adv. 2017;7(36):22409–22414.