Abstract
An enclosed air-lift photobioreactor (ALPBR) is considered an efficient lab-scale bioreactor for microalgae cell growth and lipid biosynthesis. However, fluid-induced shear stress and mixing are two main factors that affect physiological metabolism in microalgal cell cultures. Herein, a 50-L ALPBR after being designed and manufactured was evaluated for microalgal suspension culture. Moreover, computational fluid dynamics (CFD) simulation was used to characterize the hydrodynamics of ALPBR. Specifically, two model microalgae species Chlorella vulgaris and Chlorella protothecoides were cultured with aeration rates at 0.1, 0.2, 0.3, 0.4 volume per volume per minute (vvm). The hydrodynamics of ALPBR while considering shear rate, volume average velocity, turbulent energy dissipation rate (EDR), air volume fraction, and mean air bubble diameter, etc. showed that aeration generated a very low shear stress. When C. protothecoides and C. vulgaris were cultured with various aeration rates, the maximum dry cell weights (DCW) of two microalgal species tested were 0.3 vvm and 0.2 vvm, while the maximum lipid contents of both species were about 15.0% of the DCW, which were achieved at an air flow rate of 0.2 vvm. Therefore, we concluded that the shear rate generated by aeration could play a vital role in the cell growth of microalgae grown in an air-lift photobioreactor.
Introduction
In recent years, microalgae have received considerable research attention as a renewable source of bioenergy and valuable additives like carotenoids [Citation1]. Compared with other biomass materials, microalgae have more efficient photosynthesis along with higher lipid content and shorter growth cycle [Citation2]. Currently, two types of photo-bioreactors are generally used to cultivate microalgae: open photo-bioreactors and enclosed photo-bioreactors [Citation3]. The raceway pond photo-bioreactor, the oldest type of photo-bioreactor (in continuous use), is a typical open photo-bioreactor, which has several advantages such as simple components, low cost, and easy operation [Citation4]. In addition, as the microalgae can absorb CO2 from the air, the cost required to supply CO2 (the most important raw material for microalgae growth) is greatly lowered [Citation5].
On the other hand, the enclosed photo-bioreactor (PBR) is considered the most efficient and superior type of photo-bioreactor due to certain advantages and useful applications. For example, the enclosed PBR is more suitable for laboratory scale studies of the cell physiologies and metabolisms of microalgae and photosynthetic bacteria [Citation6, Citation7]. Moreover, enclosed photo-bioreactors generate very little pollution, and algal cells can be aseptically cultured, which reduces the risk of contamination. In addition, the enclosed PBR can ensure a stable, continuous production rate unaffected by the external environment [Citation8, Citation9] as well as a high efficiency for microalgal cell growth [Citation10–14].
Generally, the microalgae growth in bioreactor culture is largely influenced by many factors such as culture environmental conditions (e.g. light intensity, temperature and pH), nutritional conditions (e.g. the concentrations of the carbon source, total nitrogen source and total phosphorus source) [Citation15–17], and the geometric configuration of the photobioreactor itself (e.g. mixing performance and shear stress) [Citation12, Citation18–20]. For example, the nutrient supply must be sufficient for cell growth, but must not interfere with product biosynthesis [Citation21]. Moreover, it is often necessary to limit the sources of nitrogen or phosphorus to support lipid accumulation [Citation12, Citation22]. Therefore, certain specific environmental conditions are required for optimal microalgal growth and lipid biosynthesis. In enclosed bioreactors, changes in aeration rates affect mixing, mass transfer, and shear environment so as to influence microalgal growth. Generally, microalgae are sensitive to shear stress [Citation23]. Among them, shear tolerance is greatest for green algae, followed by cyanobacteria, haptophytes, red algae and diatoms [Citation24]. A high shear stress may reduce microalgal growth rate, or even cause microalgal death [Citation23–25]. Due to the collective effect of lack of measurements, including local measurements in airlift reactors and measurements in real microalgae cultures, it poses serious limitations to understand the entire culturing system, which leaves a big space of error in the designing and scale-up process. Thus, the commercialization of large-scale microalgae production is still far from reality.
Due to its lower shear stress generated by aeration instead of stirring device or pumping systems [Citation26], the enclosed PBR, including bubble column PBR (BCPBR) and tubular air-lift PBR (ALPBR), is considered a useful bioreactor for cultures of shear sensitive microalgae in investigations of physiological properties and lipid biosynthesis by microalgal cells grown under different environmental conditions. Thus, a high performance in hydrodynamics is an essential prerequisite for PBR such as a low shear stress, a high mixing intensity and a sufficient light distribution etc. Noticeably, there is a negative correlation between the flow regime-induced shear stress and mixing intensity.
Although the effects of the hydrodynamic properties of fluid on microalgae grown in PBR have been documented, the relationship of the shear stress imposed by aeration to cell viability or cell damage in PRB remains unclear. However, in order to avoid stressful cultivation conditions, there is still a huge gap for improvement in the design of air-agitated PBRs [Citation24]. In the present study, we adopted the computational fluid dynamics (CFD) simulation to calculate different hydrodynamic properties in fluids in a 50-L enclosed ALPBR, which was designed and manufactured by our group, under different aeration conditions. Furthermore, the impact of the hydrodynamics on cell viability and lipid biosynthesis in two model microalgae species, Chlorella vulgaris and Chlorella protothecoides, were evaluated. The key factors affecting microalgae cell growth and lipid biosynthesis were also explored.
Materials and methods
Microalgae
The algal species used in this study are C. vulgaris and C. protothecoides, which were gifted by the Institute of Bioenergy and Bioprocess Technology, Chinese Academy of Sciences.
Microalgae culture preparation
C. vulgaris and C. protothecoides were grown as described previously [Citation27]. The cultures were grown 250-mL Erlenmeyer shake flasks containing 100 mL BG-11 medium under continuous light radiation (approximately 50 μmol photons/(m2 · s)) in a rotary shaker (125 rpm, 25 °C) for 10 d. Then, the seed culture with an inoculum of 5.0% (v/v) was introduced into a 50-L air-lift photobioreactor (ALPBR) containing 38 L of BG-11 inorganic medium. The culture conditions were as follows: The temperature was 28 °C and the aeration rate was controlled at 0.1, 0.2, 0.3, 0.4 air volume per unit medium volume per minute (vvm) with 5.0% CO2, respectively, corresponding to air flow rates of 4.0, 8.0, 12.0 and 16.0 L/min.
Design of an enclosed ALPBR
The air-lift photobioreactor (ALPBR) was designed based on a 50-L cylindrical fermenter (). The illumination was provided by three internal light tubes inserted evenly in the reactor lid. Each tube was nearly as long as the bioreactor and the intensity of the light could be altered by adjusting the power and number of the LED lamps. Therefore, a concentric-tube was installed in the middle of the reactor to increase fluid flow and mixing within the bioreactor. In this way, the ALPBR was well mixed, either throughout or in the direction of illumination.
CFD simulation method for a 50-L ALPBR
The hydrodynamic parameters under four aeration rates (0.1, 0.2, 0.3 and 0.4 vvm) were calculated. The engineering parameters of the bioreactor that were determined through the simulation were the velocity, turbulence energy dissipation rate (EDR), shear rate, air volume fraction and mean air bubble diameter. The Euler-Euler two-phase flow model was used to simulate the two phase flow of gas and liquid in the bioreactor; the standard k-ε model was applied to simulate turbulence; and the population balance model (PBM) was used to simulate air bubble size distribution [Citation28]. Meshing and model building were performed in ANASYS ICEM CFD v15.0 and ANSYS CFX v15.0 (ANSYS, USA). The grids near the light tube, the draft tube, and the sparger were refined. There were approximately 1.5 million grids in the entire reactor. The model was run on a 96-core Sugon sever (Sugon Co., Ltd., China). During the simulation, the calculated residual and the air volume fraction in the reactor were monitored. The calculation was considered convergent when the residual was <10−4 and the air volume fraction was stable.
Analytical methods
For dry cell weight (DCW), the algal cells were harvested by centrifugation at 500×g for 10 min, washed twice with distilled water, then the precipitate was dried at 105 °C till a constant cell weight. Total nitrogen (TN) and total phosphorus (TP) were measured using a spectrophotometer (UV2450, Shimadzu, Japan) after the samples were filtered through 0.45-μm Millipore membrane. The total amount of microalgal cells and the viable cells were counted in a cell chamber by microscope (Model: Countstar® Altair, Shanghai Ruiyu, China) using methylene blue staining. Briefly, microalgal cells were stained by 0.4% methylene blue for 2 min, then transfered to a cell chamber installed in a microscope. The viability of the microalgae was taken as the percentage of living to dead cells in cultures. It was calculated based on the uptake of methylene blue in solution by dead cells, which was described in a previous study [Citation29].
Cellular lipid content and their fatty acids profile in C. vulgaris and C. protothecoides were determined by gas chromatography–mass spectrometry (GC-MS) (Agilent Technologies 7890 C GC System and 5975 C inert MSD with Triple-Axis Detecter, Santa Clara, CA, USA) with a fused silica capillary column (Length 30 m, I.D. 0.25 mm, Thickness 0.25 μm, Agilent Technologies, USA) after direct transesterification. Samples were prepared by following the procedure described previously [Citation30]. After preparing samples, 1 μL sample of injection volume was used at a split ratio of 10:1. The initial oven temperature of 160 °C was set for two minutes and then increased by 5 °C/min to 230 °C and held for two minutes. The temperatures of the FID-detector and the injector port were set at 280 °C and 250 °C. Helium gas at a flow rate of 1.0 mL/min was used as the carrier. The known amounts of internal standards were used for quantitation of individual FAME. At last, peaks were identified by comparing the retention time with ion fragments information to those of reference standards. The cellular lipid content was expressed as total fatty acid methyl esters (FAMEs percentage of dry cell weight).
Statistical analysis
For statistical analysis, SPSS 19 software was used. The obtained statistical results were shown as mean values with standard deviation (±SD). Moreover, Student's test (t) was employed to analyze the mean values. Differences were considered statistically significant at p < 0.05 level.
Results and discussion
Hydrodynamic characterization of a 50 L enclosed air-lift photobioreactor
Computational fluid dynamics (CFD) is an effective and efficient modeling tool for studying the fluid flow properties of cell culture in air-lift photo-bioreactors [Citation31, Citation32]. In this work, the hydrodynamic properties of fluid in a 50-L enclosed ALPBR operated under different aeration rate conditions were calculated using CFD simulation, including velocity distribution, turbulence energy dissipation rate, shear rate, air volume fraction and mean air bubble diameter.
The velocity field under different aeration conditions
The fluid was subjected to the rising force of the air passing through the bottom of the bioreactor, and rising along the concentric tube (). The fluid dropped after reaching the top of the draft tube, descended along the space between the draft tube and the inner wall of the bioreactor, and eventually returned to the bottom of the bioreactor. At 0.1 vvm, the average flow rates inside and outside the draft tube were low (less than 0.35 and 0.175 m/s, respectively). The smallest superficial velocity at the bottom of the hypotenuse (downcomer) was nearly close to zero m/s (), which was lower than the critical superficial aeration velocity of up to 0.05 m/s that was experimented at any aeration rate in a 60-L air-lift bioreactor to achieve the shorter mixing time [Citation33, Citation34]. As the aeration rate increased, the flow rates inside and outside the draft tube also gradually increased. When the aeration rate was 0.4 vvm, the flow rates inside and outside the draft tube were less than 0.7 and 0.35 m/s, respectively. As the aeration rate increased, the flow rate inside the draft tube increased faster than the flow rate outside the draft tube (). The internal flow pattern appeared linear, while the external flow pattern appeared more chaotic. In addition, as the aeration rate increased, the degree of vortex formed by the fluid outside the draft tube also increased. At 0.1 vvm, no vortex was observed. However, from 0.2 to 0.4 vvm, vortices gradually formed outside the draft tube, and increased in number with aeration rate. Therefore, the flow rate outside the draft tube might have increased more slowly than that inside the draft tube because of the large cross-sectional area of the space outside the draft tube and the formation of vortices in this space. This was consistent with the velocity contour (). Clearly, with regard to the mixing performance in ALPBR, the minimum aeration is more than 0.1 vvm. Therefore, it is concluded that the flow hydrodynamics is an important factor, which affects the air-lift photobioreactor performance because fluid mixing governs the movement of microalgal cells and prevents their sedimentation [Citation35–37].
Turbulence energy dissipation rate (EDR) and shear rate under different aeration conditions
Generally, two critical parameters that have already been considered for microalgae culture are the average EDR and maximum shear stress [Citation38]. In order to quantitatively describe the distribution of turbulence EDR and shear stress generated by fluid flows in the bioreactor, which together were used to represent shear stress fields, were simulated with the CFD (). The turbulence EDR of the ALPBR was mainly concentrated in the interior (increasing along the draft tube) and at the position where the gas was about to leave the culture surface where gas liquid separation occurs (). When the aeration rate was 0.1 vvm, the turbulence EDR was less than 0.004 m2/s3 across most of the bioreactor. As the aeration rate increased, the turbulence EDR inside the draft tube increased continuously to an aeration rate of 0.3 vvm. Under this condition, the maximum EDR inside the draft tube was 0.04 m2/s3, and a clear T-shaped distribution of eddy dissipation was observed. The average EDR was lower at 0.4 vvm than that obtained at 0.3 vvm; however, the maximum EDR at the broth surface increased greatly. This might be because many air bubbles accumulated inside the draft tube, reducing the proportion of the liquid. Under this circumstance, there might not have been sufficient time for energy to disperse, and the bubbles rapidly burst after they accumulated at the culture surface, meanwhile, the bursting of bubbles results in detrimental forces such as the tension force to microalgal cells [Citation25].
With the increase of the air flow rate from 0.1 vvm to 0.4 vvm, the shear rates were estimated in the different zones of the ALPBR. The highest values of around 20 s−1 were found in the bottom zone of the ALPBR and in the gas-liquid separator when the air flow rate reached 0.4 vvm. As presented in , when the aeration rate was from 0.1 vvm to 0.4 vvm, the average shear rate increased from 4.26 s−1 to 7.21 s−1 (equivalent to 4.24 mPa- −7.21mPa in terms of shear stress), which nearly met the critical shear stress requirement of Dinoflagellates Protoceratium reticulatum, one of the most sensitive types of microalgae [Citation39]. Compared with the occurrence of T-shape distribution of turbulence EDR at aeration rate of 0.3 vvm, the distribution of shear rate was relatively uniform without an obvious T-line distribution (). As such, the ALPBR designed here possesses a very low shear stress generated by aeration. Thus, the results are in good agreement with the previous studies stated that the shear stress is an important factor for optimizing the microalgae cell production [Citation36, Citation40, Citation41].
Table 1. Detailed parameters of the CFD simulation in the 50 L enclosed air-lift photoreactor under different aeration rates.
Air volume fraction and mean air bubble size under different aeration conditions
Air bubbles were sparged from the bottom of the sparger with each pore size of 1.0 mm, rose along the inside of the draft tube to the culture surface, resulting in bubble coalescence and break-up (). The local gas holdup in the riser was higher than that in the downcomer, and the air volume fraction in the riser remarkably increased from the bottom to the top for all aeration rates. Moreover, the air volume fraction (gas holdup) increased gradually with the increase in aeration rate. The maximum air volume fractions under each of the four aeration rates, with the exception of a small part of the bioreactor, were less than 3.0% at 0.1 vvm, 4.2% at 0.2 vvm, 4.8% at 0.3 vvm, and 5.4% at 0.4 vvm. Under those gas holdup conditions, the calculated average liquid velocity was from 0.3 m/s to 0.5 m/s in ALPBR according to the method as described previously [Citation42], which agreed with the velocity field estimated by CFD simulation ().
The mean air bubble diameter distribution varied with the different aeration rates (). The bubble diameter was larger when the aeration rates were smaller, and gradually decreased as the aeration rate increased. This was because the degree of turbulence in the flow field increased as the aeration rate increased, causing bubbles to be broken and dispersed. Thus, the mean air bubble diameter was gradually reduced from 11.3 mm to 5.6 mm (). Bubbles generated by shear force in bioreactors exert two functions. One is to supply microalgae cells for growth with CO2 and nutrients when the bubble size is proper; another is the adverse effect of bubbles that damage cells largely due to micro-eddies caused by small bubbles. Yang et al. reported that the air bubble size ranged from 1.5 mm to 6.0 mm and algal growth declined with an increased bubble size [Citation43]. Accordingly, the average bubble size generated by flow in the air-lift photobioreactor is more than the critical size. These results can be correlated with the previously reported studies, showing that the average smaller bubble size is very important for better microalgae cell growth [Citation44, Citation45].
Growth of C. vulgaris and C. protothecoides in ALBBR with different air flow rates
Growth of C. protothecoides
The growth rate of C. protothecoides in the bioreactor varied with different aeration conditions (). At 0.1 vvm, C. protothecoides grew slowly with a small increase in dry cell weight (DCW) from 0.035 g/L to 0.075 g/L in 150 h, followed by a decline to 0.06 g/L with a stable pH value. Meanwhile, only small amounts of TN and TP in the medium were consumed (). However, the slower cell growth might be due to a low mixing intensity caused by a slow flow rate of the fluid in the bioreactor. Indeed, the CFD simulation indicated that the flow rate at the bottom of the bioreactor (except the aeration area) was less than 0.14 m/s (), which is less than the critical flow rate required for the shorter mixing time in the bioreactor [Citation46]. Under this condition, the microalgae settled at the bottom of the bioreactor due to gravity, leading to a slow growth rate.
Figure 2. Changes in dry cell weight (DCW), pH, nitrogen in nitrate and phosphorus in phosphate at aeration rates of 0.1–0.4 vvm in Chlorella protothecoides. (A) Changes in DCW and pH at aeration rate of 0.1 vvm, (B) Changes in TN and TP at aeration rate of 0.1 vvm, (C) Changes in DCW and pH at aeration rate of 0.2 vvm, (D) Changes in TN and TP at aeration rate of 0.2 vvm, (E) Changes in DCW and pH at aeration rate of 0.3–0.4 vvm and (F) Changes in TN and TP at aeration rate of 0.3–0.4 vvm.
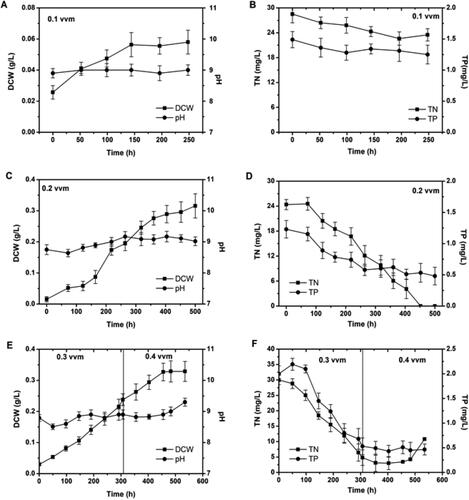
When the aeration rate was 0.2 vvm, the culture continued growing for up to 500 h, and the final DCW reached 0.32 g/L, which is 5.8 times of the peak cell density obtained at the aeration rate of 0.1 vvm. The CFD simulation indicated that the flow rate at the bottom of the reactor was 0.28 m/s. This flow rate was sufficient to re-suspend settled algal cells, greatly improving the growth performance.
In another set of experiments, the aeration rate was set to 0.3 vvm for the first 300 h (first stage), then increased to 0.4 vvm from 300 h onwards (second stage). In the first stage, the cells had a linear growth rate, and the DCW was 0.24 g/L at 300 h, which was higher than the growth rate at 0.2 vvm after 300 h. This indicated that the increased aeration rate also increased the cell growth, possibly because increased aeration increased the fluid circulation rate in the reactor. This reduced the cellular sedimentation rate, allowing more cells to be suspended in the medium. Moreover, the increase in the circulation rate also improved the reactor mixing. Microalgae cells also moved more quickly toward the light, leading to better light absorption and increased photosynthesis. The cell growth rate also increased. In the second stage, the aeration rate was increased to 0.4 vvm. The cell growth rate did not increase or decrease greatly after this change. This might be because the 0.3 vvm aeration rate provided adequate fluid mixing, fluid circulation and mass transfer for maximum photosynthesis and algal growth. Thus, greater aeration rates did not continue to accelerate cell growth. Furthermore, the viability of microalgae cells grown under different aeration conditions was presented in , demonstrating that the cell growth stopped under 0.4 vvm aeration rate due to cell damage, probably, caused by the bursting of bubbles. To gain a better understanding of the impact of aeration rate on biomass accumulation, the CFD calculation showed that higher shear rate was generated at the larger aeration rate. Microalgae have a certain tolerance for shear stress [Citation24, Citation47] and excessive shear adversely impaired cells growth [Citation23, Citation36]. Thus, 0.3 vvm is considered as a more suitable aeration rate for microalgal growth, which supports cell growth without damaging cells. The results are in line with a previous report that the increased aeration rate results in increased shear stress which may negatively affect the microalgae growth rate [Citation48].
Table 2. Cell viability (%) of C. vulgaris and C. protothecoides cultivated in ALBBR with different air flow rates.
Growth of C. vulgaris
The growth of C. vulgaris at 0.1 vvm was similar to that of C. protothecoides (). Similar to C. protothecoides, cell sedimentation could not be overcome at a low aeration rate. The biomass increased only slightly at the beginning of culture (from 0.025 g/L to 0.058 g/L), and did not change at all after 150 h.
Figure 3. Changes in dry cell weight (DCW), pH, nitrogen in nitrate and phosphorus in phosphate at aeration rates of 0.1–0.4 vvm in Chlorella vulgaris. (A) Changes in DCW and pH at aeration rate of 0.1 vvm, (B) Changes in TN and TP at aeration rate of 0.1 vvm, (C) Changes in DCW and pH at aeration rate of 0.2 and 0.4 vvm, (D) Changes in TN and TP at aeration rate of 0.2 and 0.4 vvm.
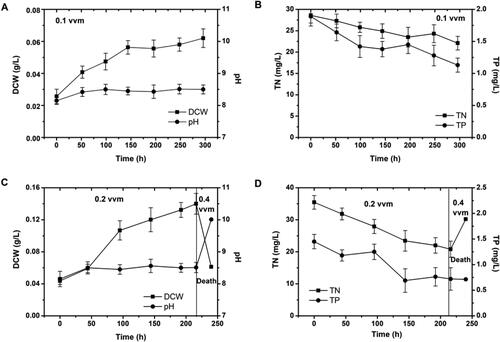
In another set of experiments, C. vulgaris was cultured in two stages. In the first stage, the aeration ratio was 0.2 vvm. During this stage, the cells grew slowly, and the concentrations of total nitrogen and total phosphorus in the substrate gradually decreased. However, the growth rate of C. vulgaris was slower than that of C. protothecoides at 0.2 vvm. After 100 h at 0.2 vvm, the biomass reached a maximum of 0.1 g/L. As it was possible that the sedimentation effect for C. vulgaris might still be strong at an aeration rate of 0.2 vvm, the aeration rate was increased to 0.4 vvm after 216 h. However, after 12 h at 0.4 vvm, all of the C. vulgaris cells in the reactor had died and the cell viability was very low (). It is possible that when the air flow rate increased from 0.2 vvm to 0.4 vvm, the corresponding increase in shear damaged and eventually killed the C. vulgaris cells. Thus, at higher aeration rate, the shear stress is also increased, which negatively affects the growth rate of microalgae [Citation48]. Interestingly, C. protothecoides cells were not dead under these aeration conditions. Therefore, C. protothecoides can withstand a greater degree of shear stress than C. vulgaris.
The average turbulence eddy dissipation at 0.1, 0.2, 0.3 and 0.4 vvm were 0.0019, 0.0067, 0.0156 and 0.026 m2/s3, respectively, while the average shear rate was 4.26, 5.63, 6.52 and 7.21 s−1, respectively (). Thus, in combination with the above culture experiments, it is indicated that the maximum shear rate tolerated by C. vulgaris was below 7.21 s−1, while the maximum shear tolerated by C. protothecoides was above 7.21 s−1. This suggested that the shear tolerance of microalgae is species- or strain-specific. For example, Pawar et al. [Citation46] found that the microalgae cells grown in an air-lift bioreactor in suspension culture experienced an average shear of 23.52–44.56 s−1 which is far below the critical limit of cell damage. The sensitivity of microalgae cells to shear stress should be carefully considered prior to microalgal culture. Although excessive shear stress increases the bioreactor mixing and mass transfer, it also may lead to cell damage or death, resulting in a failed culture [Citation48]. Therefore, there is a correlation between the flow regime-induced shear stress and mixing intensity.
Lipid biosynthesis of two microalgae under different culture conditions
Microalgal biomass is considered as an important source of useful bio-products such as vitamins and lipids (polyunsaturated fatty acids) [Citation49, Citation50]. In this study, the lipid contents of both species (C. vulgaris and C. protothecoides) were about 15.0% of dry cell weight as shown in . Based on these results, it seems obvious that there was no significant difference between these species. Both these species presented almost the same percentage of TGAs. Moreover, the composition and proportion of the fatty acids in the two algal species were similar, but with some important differences. The composition and proportion of the fatty acids of C. protothecoides and C. vulgaris are shown in and respectively. The differences in the composition and proportion of the fatty acids between the two microalgal species are largely due to the microalgal species’ tolerance to shear stress generated by different aeration rates tested in this work. Moreover, both these species produced a large amount of polyunsaturated fatty acids (PUFAs), especially C18:2 ω6 and C18:3 ω3. The results are in good agreement with some previous studies reporting that microalgae are the main primary producers of PUFAs [Citation51, Citation52].
Figure 4. Lipid contents in cells of Chlorella vulgaris and Chlorella protothecoides, grown under different aeration rates.
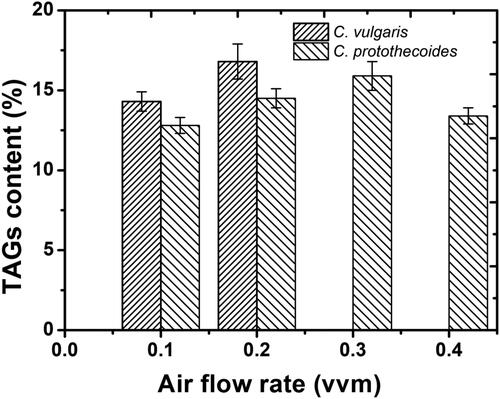
Conclusions
In this study, the developed ALPBR has low shear stress and high mixing performance. The fluid-induced shear stress influences the biomass and lipid biosynthesis of microalgal cells when C. vulgaris or C. protothecoides were grown in an enclosed ALPBR with different aeration rates. The optimum aeration rates for microalgal cell growth were different: 0.2 vvm for C. vulgaris or 0.3 vvm for C. protothecoides. However, the lipid content in both species accounted for ca. 15% of the dry cell weight with slightly different composition and proportion of the fatty acids. Excessive aeration leads to a high shear rate, which may cause cell death.
Authors’ contributions
ND, CL and MG conceived and designed the study. ND and TW performed the experiments. CL and TW contributed to data analysis and interpretation, and manuscript writing. ND, AM, MG and ZS revised the manuscript, and all authors have read and approved the final manuscript.
Consent for publication
Not applicable.
Ethics approval and concept to participate
Not applicable.
Abbreviations | ||
CFD | = | computational fluid dynamics |
DCW | = | dry cell weight |
DO | = | dissolved oxygen |
PBM | = | population balance model |
TAG | = | triacylglyceride |
Supplemental Material
Download PDF (845 KB)Availability of data and materials
The data that support the findings of this study are available from the corresponding author, [Meijin Guo], upon reasonable request.
Disclosure statement
No potential conflict of interest was reported by the authors.
Additional information
Funding
References
- Adeniyi OM, Azimov U, Burluka A. Algae biofuel: current status and future applications. Renewable Sustainable Energy Rev. 2018;90:316–335.
- Nascimento IA, Marques SSI, Cabanelas ITD, et al. Screening microalgae strains for biodiesel production: lipid productivity and estimation of fuel quality based on fatty acids profiles as selective criteria. Bioenerg Res. 2013;6(1):1–13.
- Carvalho A, Silva S, Baptista JM, et al. Light requirements in microalgal photobioreactors: an overview of biophotonic aspects. Appl Microbiol Biotechnol. 2011;89(5):1275–1288.
- Sawant S, Khadamkar H, Mathpati C, et al. Computational and experimental studies of high depth algal raceway pond photo-bioreactor. Renewable Energy. 2018;118:152–159.
- Patel B, Tamburic B, Zemichael FW, et al. Algal biofuels: a credible prospective? ISRN Renewable Energy. 2012;2012:1–14.
- Fan J, Huang J, Li Y, et al. Sequential heterotrophy-dilution-photoinduction cultivation for efficient microalgal biomass and lipid production. Bioresour Technol. 2012;112:206–211.
- Wang T, Tian X, Liu T, et al. A two-stage fed-batch heterotrophic culture of Chlorella protothecoides that combined nitrogen depletion with hyperosmotic stress strategy enhanced lipid yield and productivity. Process Biochem. 2017;60:74–83.
- Janssen M, Tramper J, Mur LR, et al. Enclosed photo bioreactors: light regime photosynthetic efficiency, scale-up and future prospect. Biotechnol Bioeng. 2003;81(2):193–210.
- Vargas S, Gómez Pérez CA, Espinosa J. A method for the design of a continuous microalgae culture photobioreactor in series with recirculation system, CT y F - Ciencia. CT&F Cienc Tecnol Futuro. 2017;7(1):101–116.
- Amaro H, Guedes A, Malcata F. Advances and perspectives in using microalgae to produce biodiesel. Appl Energy . 2011;88(10):3402–3410.
- González-Fernández C, Molinuevo-Salces B, García-González M. Open and enclosed photobioreactors comparison in terms of organic matter utilization, biomass chemical profile and photosynthetic efficiency. Ecol Eng. 2010;36(10):1497–1501.
- Hu Q, Sommerfeld M, Jarvis E, et al. Microalgal triacylglycerols as feedstocks for biofuel production: perspectives and advances. Plant J. 2008;54(4):621–639.
- Markov S. Hydrogen production in bioreactors: current trends. Energy Procedia. 2012;29:394–400.
- Schenk P, Thomas-Hall S, Stephens E, et al. Second generation biofuels: high-efficiency microalgae for biodiesel production. Bioenerg Res. 2008;1(1):20–43.
- Chen M, Tang H, Ma H, et al. Effect of nutrients on growth and lipid accumulation in the green algae Dunaliella tertiolecta. Bioresour Technol. 2011;102(2):1649–1655.
- Eixler S, Karsten U, Selig U. Phosphorus storage in Chlorella vulgaris (Trebouxiophyceae, Chlorophyta) cells and its dependence on phosphate supply. Phycologia. 2006;45(1):53–60.
- Pudaite JL. Effects of the absence of nitrogen and phosphorus on the growth rate and total chlorophyll content of a freshwater microalgae Scenedesmus sp. Science Vision. 2015;15(3):132–137.
- Guschina I, Harwood J. Lipids and lipid metabolism in eukaryotic algae. Prog Lipid Res. 2006;45(2):160–186.
- Xia L, Rong J, Yang H, et al. NaCl as an effective inducer for lipid accumulation in freshwater microalgae Desmodesmus abundans. Bioresour Technol. 2014;161:402–409.
- Xiong W, Gao C, Yan D, et al. Double CO(2) fixation in photosynthesis-fermentation model enhances algal lipid synthesis for biodiesel production. Bioresour Technol. 2010;101(7):2287–2293.
- Zheng Y, Li T, Yu X, et al. High-density fed-batch culture of a thermotolerant microalga Chlorella sorokiniana for biofuel production. Appl Energy. 2013;108:281–287.
- Courchesne N, Parisien A, Wang B, et al. Enhancement of lipid production using biochemical, genetic and transcription factor engineering approaches. J Biotechnol. 2009;141(1–2):31–41.
- Michels MHA, van der Goot AJ, Vermuë MH, et al. Cultivation of shear stress sensitive and tolerant microalgal species in a tubular photobioreactor equipped with a centrifugal pump. J Appl Phycol. 2016;28(1):53–62.
- Wang C, Lan C. Effects of shear stress on microalgae – a review. Biotechnol Adv. 2018;36(4):986–1002.
- Gallardo-Rodríguez JJ, López-Rosales L, Sánchez-Mirón A, et al. New insights into shear-sensitivity in dinoflagellate microalgae. Bioresour Technol. 2016;200:699–705.
- López-Rosales L, García-Camacho F, Sánchez-Mirón A, et al. Modeling shear-sensitive dinoflagellate microalgae growth in bubble column photobioreactors. Bioresour Technol. 2017;245(Pt A):250–257.
- Ge Y, Liu J, Tian G. Growth characteristics of Botryococcus braunii 765 under high CO2 concentration in photobioreactor. Bioresour Technol . 2011;102(1):130–134.
- Ranganathan P, Savithri S. Investigations on hydrodynamics and mass transfer in gas–liquid stirred reactor using computational fluid dynamics. Chem Eng Sci. 2011;66(14):3108–3124.
- Bicas JL, Kleinegris DMM, Barbosa MJ. Use of methylene blue uptake for assessing cell viability of colony-forming microalgae. Algal Research. 2015;8:174–180.
- Wang T, Ge H, Liu T, et al. Salt stress induced lipid accumulation in heterotrophic culture cells of Chlorella protothecoides: mechanisms based on the multi-level analysis of oxidative response, key enzyme activity and biochemical alteration. J Biotechnol. 2016;228:18–27.
- Aslanbay Guler B, Deniz I, Demirel Z, et al. Computational fluid dynamics simulation in scaling-up of airlift photobioreactor for astaxanthin production. J Biosci Bioeng. 2020;129(1):86–92.
- Sadeghizadeh A, Rahimi R, Dad FF. Computational fluid dynamics modeling of carbon dioxide capture from air using biocatalyst in an airlift reactor. Bioresour Technol. 2018;253:154–164.
- Sánchez Mirón A, Cerón García MC, García Camacho F, et al. Mixing in bubble column and airlift reactors. Chem Eng Res Des. 2004;82(10):1367–1374.
- Sánchez Mirón A, García Camacho F, Contreras Gómez A, et al. Bubble-column and airlift photobioreactors for algal culture. AIChE J. 2000;46(9):1872–1887.
- Aslanbay Guler B, Deniz I, Demirel Z, et al. Evaluation of scale-up methodologies and computational fluid dynamics simulation for fucoxanthin production in airlift photobioareactor. Asia-Pacific J Chem Eng. 2020; e2532.
- Gao X, Kong B, Vigil RD. Multiphysics simulation of algal growth in an airlift photobioreactor: effects of fluid mixing and shear stress. Bioresour Technol. 2018;251:75–83.
- Tong Z-X, Li M-J, Yan J-J, et al. A theoretical analysis of the hydrodynamic influence on the growth of microalgae in the photobioreactors with simple growth kinetics. Int J Heat Mass Transf. 2020;158:119986.
- Michels MHA, van der Goot AJ, Norsker N-H, et al. Effects of shear stress on the microalgae Chaetoceros muelleri. Bioprocess Biosyst Eng. 2010;33(8):921–927.
- García Camacho F, Gallardo Rodríguez JJ, Sánchez Mirón A, et al. Determination of shear stress thresholds in toxic dinoflagellates cultured in shaken flasks: implications in bioprocess engineering. Process Biochem. 2007;42(11):1506–1515.
- Gao X, Kong B, Vigil RD. CFD simulation of bubbly turbulent Tayor–Couette flow. Chin J Chem Eng. 2016;24(6):719–727.
- Gao X, Kong B, Vigil RD. Characteristic time scales of mixing, mass transfer and biomass growth in a Taylor vortex algal photobioreactor. Bioresour Technol. 2015;198:283–291.
- Luo H-P, Al-Dahhan MH. Local gas holdup in a draft tube airlift bioreactor. Chem Eng Sci. 2010;65(15):4503–4510.
- Yang Z, Pei H, Han F, et al. Effects of air bubble size on algal growth rate and lipid accumulation using fine-pore diffuser photobioreactors. Algal Research. 2018;32:293–299.
- Barahoei M, Hatamipour MS, Afsharzadeh S. CO2 capturing by chlorella vulgaris in a bubble column photo-bioreactor; effect of bubble size on CO2 removal and growth rate. J Co2 Util. 2020;37:9–19.
- El Shenawy EA, Elkelawy M, Bastawissi HA-E, et al. Effect of cultivation parameters and heat management on the algae species growth conditions and biomass production in a continuous feedstock photobioreactor. Renewable Energy. 2020;148:807–815.
- Pawar SB. Computational fluid dynamics (CFD) analysis of airlift bioreactor: effect of draft tube configurations on hydrodynamics, cell suspension, and shear rate. Bioprocess Biosyst Eng. 2018;41(1):31–45.
- Barbosa MJ, Hadiyanto H, Wijffels R. Overcoming shear stress of microalgae cultures in sparged photobioreactors. Biotechnology and Bioengineering. 2004;85:78–85.
- Coşgun A, Günay ME, Yıldırım R. Exploring the critical factors of algal biomass and lipid production for renewable fuel production by machine learning. Renewable Energy. 2021;163:1299–1317.
- Caporgno MP, Haberkorn I, Böcker L, et al. Cultivation of Chlorella protothecoides under different growth modes and its utilisation in oil/water emulsions. Bioresour Technol. 2019;288:121476
- Caporgno MP, Mathys A. Trends in microalgae incorporation into innovative food products with potential health benefits. Front Nutr. 2018;5:58(
- Canelli G, Neutsch L, Carpine R, et al. Chlorella vulgaris in a heterotrophic bioprocess: study of the lipid bioaccessibility and oxidative stability. Algal Research. 2020;45:101754.
- Morales-Sánchez D, Martinez-Rodriguez OA, Martinez A. Heterotrophic cultivation of microalgae: production of metabolites of commercial interest. J Chem Technol Biotechnol. 2017;92(5):925–936.