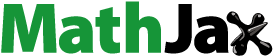
Abstract
The potential applications of β-mannanase in bioenergy, biorefinery, detergent, fodder, food processing, oil and gas, pharmaceutical, pulp and paper and textile industries, and the high cost of commercially available enzyme have attracted considerable research efforts to identify potent microbial strains for cost-effective production of this enzyme. The advantage of microbial biocatalyst is that the crude enzyme can be used directly in bioprocessing instead of the purified enzyme. In this study, β-mannanase was extracted from a novel Streptomyces sp. Alg-S25 (GenBank accession no. MK494177) and immobilized onto chitosan nanoparticles using glutaraldehyde as a crosslinker. The covalent binding of β-mannanase onto chitosan nanoparticles was confirmed by Fourier-transform infrared spectroscopy. The functionalized chitosan nanoparticles retained 83.6% ± 3.46% of β-mannanase activity. Free and immobilized β-mannanase of this novel Streptomyces sp. Alg-S25 displayed maximal activities at pH 7.5 and at temperature 50 °C and 55 °C, respectively. Immobilized β-mannanase exhibited higher values of activation energy (47.4 kJ/mol), enthalpy (44.9 kJ/mol) and entropy (–73.5 J/mol/K) of catalysis compared to the free enzyme. The substrate-specific kinetic analysis suggested that the immobilized β-mannanase had a strong affinity toward locust bean galactomannan by showing a low Km (4.94 mg/mL) and a high Vmax (93.5 U/mg-protein) values. Furthermore, the immobilized β-mannanase was not affected by 2% NaCl and retained 81.2% of the maximal activity after 10 cycles of successive reuse. These results indicate the potential of chitosan nanoparticle-immobilized β-mannanase for sustainable and cost-effective bioprocessing of mannan-containing agricultural, forest, seaweed and industrial wastes.
Introduction
In recent years, there has been a growing interest to develop biorefinery processes for the deconstruction of lignocellulosic wastes into second-generation biofuels and value-added products with potential industrial applications [Citation1–3]. Lignocellulose is the primary structural component of woody and nonwoody (e.g. grass) plants, and is composed of cellulose, hemicellulose and lignin [Citation4,Citation5]. Lignocellulosic wastes have been generated via the forest and agricultural residues and during the processing of plant biomass in various industries, including pulp and papers, food and agriculture (extracts of coffee bean, copra meal, palm kernel, konjac gum) and timber (sawmills, furniture, plywood) [Citation6,Citation7]. Among the lignocellulosic wastes, agro-industrial residues have received special attention as the sustainable feedstocks for the biorefinery because of their abundance and cost-effectiveness [Citation8–10]. Reports indicate that cellulose and hemicellulose constitute greater than 50% of the dry weight of agro-industrial wastes [Citation10,Citation11].
Hemicellulose, which is the second most abundant carbohydrate biopolymer on earth, is a group of various types of structural polysaccharides present in plant cell walls [Citation12,Citation13]. These structural polysaccharides are generally composed of the monomer sugar compounds: d-xylose, d-mannose, d-glucose, d-galactose, l-arabinose, 4-O-methyl-d-glucuronic acid [Citation12,Citation14]. Xylan and mannan are the most abundant structural polysaccharides of the heteropolymeric hemicellulose content of hardwood and softwood, respectively [Citation6,Citation15,Citation16]. Mannan is also present as a non-starch reserve component in the endosperm wall of seeds (coconut, coffee and locust beans), and in vacuoles (konjac, ivory nut and guar) [Citation14,Citation17,Citation18]. Mannan is reported as a cell wall component of several seaweed species belonging to the phyla Chlorophyta and Rhodophyta [Citation19].
Mannan is chemically composed of β-1,4-linked D-mannose (and/or glucose) and α-1,6-linked galactose as the backbone and side chain, respectively [reviewed in Citation16,Citation20]. Due to the complexity of the structure of mannan, the complete hydrolysis of this biopolymer is achieved via the synergistic action of four enzymes: β-mannanase (EC3.2.1.78), β-mannosidase (EC3.2.1.25), β-glucosidase (EC 3.2.1.21) and α-galactosidase (EC3.2.1.22) [reviewed in Citation14,Citation15]. Among these enzymes, β-mannanase has received substantial industrial attention because it catalyzes the cleavage of β-1,4 linkages in mannan into biologically active mannooligosaccharides. These mannooligosaccharides are subsequently hydrolyzed by the other three mannan-deconstructing enzymes. The potential industrial applications of β-mannanase are listed in .
Table 1. Biotechnological applications of β-mannanase.
These potential applications of β-mannanase in various industrial sectors have attracted research efforts for the screening of potent mannan-degrading microorganisms from diverse environments to be used as a biocatalyst for cost-effective production of this enzyme [Citation14,Citation20]. Additionally, efforts have been devoted for making the microbial biocatalyst operational under the harsh processes commonly used in the industries [Citation30,Citation31]. The advantage of microbial biocatalysts is that the crude enzyme can be used directly instead of the purified enzyme, which leads to a reduction in the cost of bioprocesses [Citation32–34].
Immobilization of the enzyme on the surface of polymeric matrices, including natural (alginate, chitosan, collagen and carrageenan) and synthetic (polyacrylamide, amberlite and polyvinyl alcohol) compounds, makes the biocatalyst more resistant to the harsh industrial processes [Citation35,Citation36]. In recent years, there has been a growing interest to utilize chitosan nanoparticles as the immobilized matrix because of its abundance, biocompatibility, biodegradability and nontoxicity [Citation37,Citation38]. Chitosan is produced industrially from chitin via N-deacetylation reaction. The advantage of immobilization on nano-sized particles is the high surface-to-area ratio, which helps in higher loading of enzyme, higher reaction rate and resistance to fouling and diffusion [Citation39,Citation40]. The surface covalent bonding of an enzyme onto chitosan nanoparticles occurs by treating the nanoparticles with a crosslinker (e.g. glutaraldehyde). Thereby, the bifunctional –CHO groups of glutaraldehyde react with the –NH2 groups present in chitosan and in the enzyme [Citation41,Citation42].
This article describes the characteristics of free and chitosan-nanoparticle-immobilized β-mannanase of a novel Streptomyces sp. Alg-S25 isolated from the decomposed invasive Sargassum seaweed wastes accumulated in huge quantities of the coast off Barbados.
Materials and methods
Isolation and screening of actinobacteria for β-mannanase activity
Thirty-one actinobacteria were isolated from decomposed Sargassum seaweeds piled up at the Harrismith beach (Latitude 13.12 °N and Longitude 59.42 °W), Barbados. The decomposed Sargassum seaweed biomass was composed of two invasive floating species, Sargassum fluitans and Sargassum natans. After a gentle rinse of 1 g of Sargassum biomass with sterile seawater, the sample was homogenized with 9 mL of sterile seawater. Actinobacteria were isolated from this homogenized resuspension by serial dilutions and spreading of 0.1 mL of diluents onto mineral medium (0.02% MgSO4, 0.002% CaCl2, 0.1% KH2PO4, 0.1% K2HPO4, 2% NaCl, 0.1% NH4NO3; pH 7.5) containing 0.5% (w/v) locust bean galactomannan (LBG) (Sigma-Aldrich, Saint Louis, MO) and 1.5% (w/v) agar. The Petri plates were incubated at 30 °C for 7 d. The purification of the resulting actinobacterial colonies was performed by streaking three times on actinomycetes isolation agar (Himedia Laboratories, Mumbai) amended with 2% NaCl. For the screening of β-mannanase activity, 10 µL of each actinobacterial culture [pre-grown in actinomycetes isolation broth (Himedia Laboratories, Mumbai) amended with 2% NaCl] was spot inoculated onto the aforementioned mineral medium containing 0.5% (w/v) LBG and 1.5% (w/v) agar. The Petri plates were incubated at 30 °C for 24 h, followed by flooding the Petri plates with Gram’s iodine to visualize the β-mannanase activity as a halo around the positive isolates [Citation43]. One isolate (Alg-S25), which showed the highest clearing zone (ca. 20 mm), was selected to assess the biocatalytic characteristics of its extracted β-mannanase activity immobilized on chitosan nanoparticles.
Molecular identification of Alg-S25
The phylogenetic affiliation of the Alg-S25 isolate was determined by sequencing its 16S rRNA gene. After extraction of the genomic DNA of Alg-S25 using the InstaGene DNA extraction kit (Bio-Rad Laboratories, Canada), the 16S rRNA gene was amplified with the primers set 27 F (5′-AGAGTTTGATCCTGGCTCAG-3′) and 1492 R (5′-GGTTACCTTGTTACGACTT-3′) with Thermo ScientificTM 2X PCR Master Mix (Thermo Fisher Scientific, USA) and an Edvotek (Bethesda, MD, USA) thermal cycler. The polymerase chain reaction (PCR) conditions used for 16S rRNA gene amplification were: initial denaturation at 95 °C for 5 min (1 cycle); denaturation at 94 °C for 30 s, annealing at 56 °C for 1 min and extension at 72 °C for 90 s (30cycles); and a final extension at 72 °C for 10 min (1 cycle). The PCR product underwent electrophoresis in 1% agarose gel supplemented with 1X SYBR™ safe DNA stain (Life Science Technologies, USA). After recording a single band (ca. 1500 bp) in electrophoresis, the PCR product was purified via IBI PCR/DNA fragment gel extraction kit (IBI Scientific, Peosta, IA, USA). Then the purified PCR product was sequenced in both the directions using 27F and 1492R primers and ABI 3730XL DNA analyser (Applied Biosystems, Foster City, CA, USA). The consensus sequence of 16S rRNA gene of Alg-S25 was generated with BioEdit software [Citation44]. The BLAST search of the consensus 16S rRNA gene sequence was performed in GenBank using the 16S rRNA sequences (Bacteria and Archaea) database. A neighbor-joining bootstrap phylogenetic tree of the closely related species of Alg-S25 was drawn with MEGA 7.0 using Jukes-Cantor algorithm and 1000 iterations [Citation45].
β-mannanase extraction
Batch cultivation of Alg-S25 isolate was conducted in 50 mL of mineral medium amended with 0.5% of LBG in Erlenmeyer’s flasks (24 × 250 mL) at 35 °C and 150 rpm shaking for 5 d. Bacterial cells were removed from the cultures by centrifugation (10,000g for 30 min at 4 °C). The supernatant was filtered under gentle vacuum through a 0.22 µm nominal pore size nitrocellulose membrane (Millipore, France), followed by precipitation of the enzyme in the resulting filtrate by the addition of 80% (w/v) (NH4)2SO4. The precipitated proteins were harvested via centrifugation (12,000g for 30 min at 4 °C), followed by addition of 20 mL of 50 mmol/L Trizma™ buffer (pH 7.5) to dissolve the protein, filtration under gentle vacuum through a 0.22 µm nominal pore size nitrocellulose membrane and ultrafiltration of the filtrate via the Vivaspin20™ 10 kDa cut-off ultrafiltration units (Vivaproducts Inc., Littleton, MA, USA). The concentrated fraction was diluted with 50 mL of 50 mmol/L Trizma™ buffer (pH 7.5) and used for immobilization as well as enzymatic characterization.
Chitosan nanoparticles preparation
The nanoparticles preparation was made via ionotropic gelation technique as described previously [Citation42]. Briefly, the resuspension obtained after the addition of 1 mL of 1.4 mol/L sodium sulfate solution to 19 mL of 0.25% (w/v) chitosan solution in 0.35 mol/L acetic acid, was agitated (500 rpm) at 25 °C on a magnetic stirrer for 4 h. The resulting nanoparticles were harvested by centrifugation (12,000g for 30 min at 4 °C), then washed with 50 mmol/L Trizma™ buffer (pH 7.5) three times. The washed nanoparticles were activated with the addition of 2% glutaraldehyde solution in the same buffer as a crosslinker and then shaking at 50 rpm and 30 °C for 2 h. The activated nanoparticles were harvested via centrifugation (12,000g for 30 min at 4 °C), followed by washing six times with 50 mmol/L Trizma™ buffer (pH 7.5) to remove the residual glutaraldehyde.
β-mannanase immobilization
Twenty milliliters of extracted β-mannanase were added to the activated chitosan nanoparticles, and then, gently stirred at 4 °C for 36 h. The chitosan nanoparticles containing the immobilized β-mannanase were collected by centrifugation (12,000g for 30 min at 4 °C), followed by repeated washing with 50 mmol/L Trizma™ buffer (pH 7.5) to remove the unbound β-mannanase. After the non-detection of β-mannanase activity in the washing fractions, 30 mL of 50 mmol/L Trizma™ buffer (pH 7.5) were added to the enzyme-immobilized nanoparticles. This resuspension of chitosan nanoparticles containing immobilized β-mannanase was mixed gently prior to performing the assays. The β-mannanase activity bound (MAB) onto activated chitosan nanoparticles was estimated from EquationEquation (1)(1)
(1) .
(1)
(1)
Where MAi is the β-mannanase activity added, MAs is the β-mannanase activity measured in the supernatant after collection of nanoparticles, MAw is the β-mannanase activity measured in the pooled washing fractions.
Fourier-transform infrared spectroscopy of immobilized β-mannanase
The immobilization of β-mannanase activity onto glutaraldehyde-activated chitosan was verified by comparing the Fourier-transform infrared (FTIR) spectra of activated chitosan nanoparticles before and after enzyme immobilization. The freeze-dried samples of activated chitosan nanoparticles before and after enzyme immobilization were mixed with 1% (w/w) KBr, and the FTIR spectra of the pellets were acquired with a Shimadzu FTIR spectrometer at 4 cm−1 resolutions.
Assay of β-mannanase activity
The activities of free and chitosan-nanoparticle-immobilized β-mannanase were determined in a standard assay mixture consisting of 0.1 mL of free or immobilized enzyme preparation, 0.4 mL of 50 mmol/L Trizma™ buffer (pH 7.5) and 0.5 mL of 1% of LBG resuspended in the same buffer. The assay mixture of free and immobilized enzyme was incubated for 30 min at 50 °C and 55 °C, respectively. Then, the enzyme-catalyzed reaction was stopped with the addition of DNS reagents, followed by heating of the assay mixture at 100 °C for 10 min. The reducing-sugar released in the assay mixture was quantified colorimetrially at 540 nm with d-mannose as the standard [Citation46]. One unit of β-mannanase activity was defined as the release of 1 µmol of d-mannose per min under the aforementioned assay conditions.
Estimation of protein
Bradford method was used to quantify the protein concentration in free and immobilized β-mannanase preparation using bovine serum albumin as standard. EquationEquation (2)(2)
(2) was used to calculate the protein content in chitosan-nanoparticle immobilized enzyme preparation (Pimmobilized).
(2)
(2)
Where Pi is the concentration of protein added, Ps is the concentration of protein detected in the supernatant, Pw is the concentration of protein detected in the pooled washing fractions.
pH influence on β-mannanase activity and stability
Buffers of different pH (50 mmol/L phosphate-citrate for pH 5 and 6, 50 mmol/L Trizma™ for pH 7, 7.5 and 8 and 50 mmol/L borate for pH 9 and 10) were used to assess the influence of pH on free and nanoparticle-immobilized β-mannanase activity and stability. The influence of pH on enzyme activity was examined by adding these buffers individually to the standard assay mixture, followed by estimating the enzyme activity at a particular pH as specified in the assay of β-mannanase activity section. The effect of pH on enzyme stability was evaluated by pre-incubating the free and immobilized β-mannanase with buffers of varying pH (5, 6, 7, 7.5, 8, 9 and 10) at 30 °C for 30 min, and then, assaying the residual β-mannanase activity.
Temperature influence on β-mannanase activity and stability
The standard assay mixture containing the free or immobilized β-mannanase was incubated at different temperatures (10–75 °C with 5 °C increments), followed by assaying the enzyme activity at a particular temperature. The thermodynamic parameters for β-mannanase catalysis, such as activation energy (Ea), enthalpy (ΔHa) and entropy (ΔSa) were calculated from the equations of Arrhenius (EquationEquation (3)(3)
(3) ) and Eyring–Polanyi (EquationEquation (4)
(4)
(4) ), respectively.
(3)
(3)
where V is the β-mannanase specific activity, T is the absolute temperature (K), R is the universal gas constant (8.3145 J/mol/K) and A is the pre-exponential factor.
(4)
(4)
where h is Planck’s constant (6.226 × 10−34 J s) and kb is Boltzmann’s constant (1.381 × 10−23 J/K).
For assessing the influence of temperature on β-mannanase stability, the free and nanoparticle-immobilized enzyme was incubated at 5–70 °C (in steps of 5 °C) for 30 min and then rapidly cooled to 4 °C. The residual β-mannanase activity was estimated as specified above.
Effect of sodium chloride
To evaluate the effect of NaCl, the assay mixture was incorporated with different concentrations of NaCl (1–5% in steps of 1%), followed by quantification of β-mannanase activities as described above.
Kinetic parameters determination
The Km (Michaelis–Menten constant) and Vmax (maximum reaction velocity) values of free and immobilized β-mannanase activities were determined via Lineweaver–Burk plot. The substrate-specific kinetics were studied by adding varying amounts of LBG (0.5, 1, 2, 5, 10 and 15 mg/mL) to the assay mixture, and then, assaying the β-mannanase activities at each substrate concentration as aforementioned.
Influence of metal ions and inhibitors
Two different concentrations (0.1 mmol/L and 1 mmol/L) of metal ions (Ca2+, Cu2+, Mg2+, Mn2+ and Ni2+) and inhibitors [EDTA, DTT (dithiothreitol), PMSF (phenylmethanesulfonyl fluoride) and iodoacetic acid] were added to the standard assay mixture. β-mannanase activity was assayed as described above.
Recycling efficacy of chitosan nanoparticle-immobilized β-mannanase
The effectiveness of sequential applications of immobilized β-mannanase was assessed by harvesting the immobilized enzyme from the standard assay mixture after the end of each reaction, followed by washing three times with 50 mmol/L Trizma™ buffer (pH 7.5) and reuse in the next reaction. The retention of β-mannanase activity in the first reactive cycle was assigned as 100%.
Results and discussion
Identification of Streptomyces sp. Alg-S25
The phylogenetic affiliation of Alg-S25 isolate was determined as Streptomyces species Alg-S25 (GenBank accession no. MK494177) after conducting a BLAST search of its 16S rRNA gene sequence against the 16S rRNA sequences database of GenBank. illustrates the phylogenetic divergence of Alg-S25 from other closely related Streptomyces species.
Figure 1. Phylogenetic affiliation of Streptomyces sp. Alg-S25 based on 16S rRNA gene sequences. The numbers at the nodes indicate the bootstrap percentage values of 1000 iterations. GenBank accession numbers are specified in the parentheses. Scale indicates 0.002 substitutions per nucleotide position.
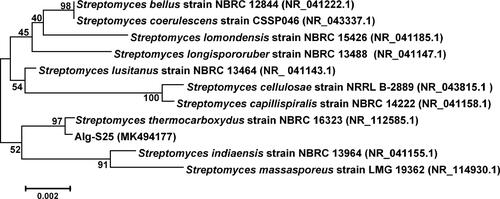
FTIR analysis
The retention of β-mannanase activity (MAB value calculated via EquationEquation (1)(1)
(1) ) on glutaraldehyde-activated chitosan nanoparticles was 83.6% ± 3.46%. ,b) depict the FTIR spectra of glutaraldehyde-activated chitosan nanoparticles and β-mannanase immobilized chitosan nanoparticles, respectively. The recordings of bands at 1412 cm−1,1564 cm−1 and 1676 cm−1 might be attributed to the vibrations of C–O–H stretching of the primary alcohol group, N–H bending and imine bond (N = C) of chitosan, respectively. The occurrence of imine bond was due to the interactions between –CHO of glutaraldehyde and –NH2 of chitosan. Additionally, the occurrence of a weak absorption band at 1724 cm−1 might be ascribed to the free –CHO groups of glutaraldehyde. The vibrations of C–H and the interactive vibrations of O–H and N–H stretching could be responsible for the observance of the broad bands at 2832–2972 cm−1 and at 3064–3500 cm−1, respectively. As depicted in , immobilization of β-mannanase onto chitosan nanoparticles led to the occurrence of a strong absorption band at 3028–3594 cm−1, which could be ascribed to the interactions of stretching vibrations of O–H and N–H groups of β-mannanase. The stretching vibrations of C–O–C and C–H of β-mannanase could result in the bands at 920 cm−1 and 1464 cm−1, respectively. After immobilization, the occurrence of bands at 1584 cm−1 and 1640 cm−1 could be assigned to the N–H bending vibrations of amide II and the C = O stretching vibrations of amide I, respectively. The overall FTIR spectral results suggest that the β-mannanase binds to the glutaraldehyde-activated chitosan nanoparticles via covalent bonding. The FTIR spectra of immobilized β-mannanase agree with the results of immobilized β-galactosidase from Kluyveromyces lactis [Citation42], cellulases from Trichoderma reesei [Citation47], alginate lyase from Arthrobacter species AD-10 [Citation48] and lipase from Rhizomucor meihei [Citation49].
Optimal pH range
The pH-enzyme activity profile of free and nanoparticle-immobilized β-mannanase revealed a common optimum at pH 7.5 (). At pH 7 and 8, the free and immobilized β-mannanase displayed the relative specific activity as 69.8% and 78.2%, and 79.4% and 89.4%, respectively. A substantial reduction in relative specific activities of free and immobilized β-mannanase were observed above pH 8, and the activities were 57.3% and 23.6%, and 65.2% and 39.8% at pH 9 and 10, respectively. The optimal pH of β-mannanase of Streptomyces sp. Alg-S25 is within the range that was previously reported for the free β-mannanase activity from Streptomyces ipomoea (pH 7.5) [Citation50] and higher than Streptomyces galbus (pH 6.5) [Citation51] and Streptomyces lividans (pH 6.8) [Citation52]. Conversely, the optimal pH was documented as pH 5 and pH 6 for β-mannanase of Clitocybe geotropa [Citation53] and Bacillus sp. CFR1601 [Citation54], respectively, immobilized on chitosan magnetic nanoparticles.
Figure 3. Influence of pH on free and immobilized β-mannanase activity. Analyses were conducted three times and data are reported as mean values ± SD.
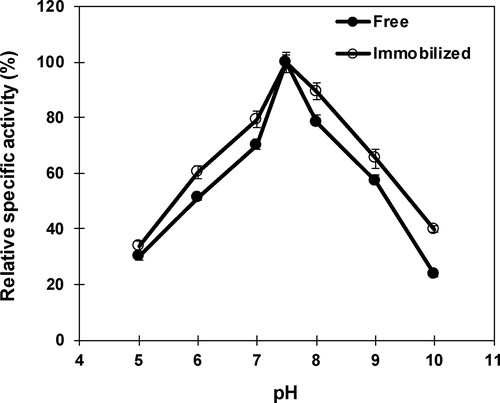
The results of the effect of pH on the stability of free and nanoparticle-immobilized β-mannanase exhibited that both free and immobilized enzyme were stable at the pH range 5–8 (). A rapid loss in relative specific activity of free and immobilized β-mannanase was recorded above pH 8 by retaining 61.7% and 66.4% of their maximal specific activities, respectively, at pH 9. The loss in β-mannanase activity at higher pH could be ascribed to the alteration of enzyme–substrate complex resulted from irreversible inactivation. The stability of free β-mannanase was reported within the range pH 5–7 and pH 7.5–8 for Paenibacillus cookie [Citation55] and Streptomyces ipomoea [Citation50], respectively. The immobilized β-mannanase of Bacillus sp. CFR1601 displayed stability between pH 6 and 10 [Citation54].
Optimal temperature range
The influence of temperatures on free and immobilized β-mannanase indicated the optimum at 50 °C and 55 °C, respectively (). Above the optimal temperature, a sharp decline in the activity was observed for both the free and immobilized enzyme. The free and immobilized β-mannanase lost 67.2% and 56.4%, respectively, of their maximal activities at 70 °C. Previous studies have reported the optimal temperature for free β-mannanase activity at 40 °C, 50 °C, 55 °C and 58 °C for Streptomyces galbus [Citation51], Bacillus sp. CFR1601 [Citation56], Streptomyces ipomoea [Citation50] and Streptomyces lividans [Citation52], respectively, and in the case of immobilized β-mannanase at 60 °C for Clitocybe geotropa [Citation53], and at 70 °C for Bacillus sp. CFR1601 [Citation54] and Penicillium occitanis [Citation57].
Thermodynamic parameters of catalysis
Free and immobilized β-mannanase exhibited the activation energy of catalysis (Ea: energy requires to form the enzyme–substrate complex) as 41.2 kJ/mol and 47.4 kJ/mol, respectively. The Ea value recorded for free β-mannanase was higher than the β-mannanase from Streptomyces sp. Alg-S23 (34.8 kJ/mol) [Citation43] and Sphingobacterium sp. GN25 (36 kJ/mol) [Citation58], and lower than Bacillus sp. CFR1601 (45.2 kJ/mol) [Citation59]. The enthalpy of catalysis (ΔHa: energy requires to transit from ground to transition state) values were estimated as 38.6 kJ/mol and 44.9 kJ/mol for free and immobilized β-mannanase, respectively, whereas the entropy of catalysis (ΔSa: energy requires per degree to shift from ground to the transition state) values were determined as −92.2 J/mol/K and −73.5 J/mol/K, respectively. Earlier studies have reported the ΔHa and ΔSa values of free β-mannanase as 28.6 kJ/mol and −41.4 J/mol/K for Bacillus subtilis subsp. inaquosorum CSB31 [Citation60] and 33.5 kJ/mol and −143.7 J/mol/K for Sphingobacterium sp. GN25 [Citation58]. Chitosan nanoparticle-immobilized β-mannanase of Alg-S25 displayed higher values of Ea, ΔHa and ΔSa, which could result from the prevention of penetration of the substrate into the immobilized matrix and/or the changes in β-mannanase conformation after immobilization [Citation61,Citation62]. This appears to be the first study on assessing the thermodynamic parameters of catalysis of immobilized β-mannanase. Therefore, a comparison of the Ea, ΔHa and ΔSa values of immobilized β-mannanase is not possible at this time with other studies.
Thermostability
The influence of temperature on the stability of free and nanoparticle-immobilized β-mannanase indicated that the immobilized enzyme was more thermostable than the free enzyme by retaining 91.1% and 76.7% of their maximal activities, respectively, at 50 °C (). A sharp decrease in the stability of free and immobilized β-mannanase was observed above 55 °C and the half-life time was recorded as 30 min at 65 °C for the immobilized enzyme, whereas the free enzyme had lost 80% of the activity at 65 °C and exhibited a half-life time of 30 min at 55 °C. Improved thremostability of immobilized β-mannanase could be attributed to the covalent bonding between free enzyme and nanoparticles, which prevents the protein unfolding and denaturation via increasing the conformational rigidity and decreasing the thermal vibrations [Citation62]. The free β-mannanase of Alg-S25 was noted to be more thermostable than the β-mannanase reported from Vibrio sp. MA-138 [Citation63] and Streptomyces galbus NR [Citation51].
Effect of NaCl
The NaCl-activity profile depicted the maximal β-mannanase activity at 2% NaCl both for the free and nanoparticle-immobilized enzyme (). Similarly, the β-mannanase activity of Vibrio sp. MA-138 isolated from seaweed was not affected by 1 mmol/L (0.006%) concentration of NaCl [Citation63]. It is worth noting that the stability toward the higher concentrations of NaCl improves the operational stability of an enzyme in industrial processes due to the presence of elevated concentrations of NaCl as impurities in bulk fermentation medium components.
Enzyme kinetics
At the optimal assay conditions, free and nanoparticle-immobilized β-mannanase of Alg-S25 exhibited Michaelis–Menten kinetics with the Km and Vmax values, determined via Lineweaver–Burk plot, as 4.14 mg/mL and 100 U/mg-protein, and 4.94 mg/mL and 93.5 U/mg-protein, respectively. The covalent bonding of β-mannanase to the glutaraldehyde-activated chitosan nanoparticles led to the decrease in the affinity of the immobilized enzyme toward the substrate because of steric hinderance of the active site, and/or diffusional limitations of the substrate and products [Citation62]. Km values of free β-mannanase were documented as 1.05 mg/mL, 1.9 mg/mL, 3.4 mg/mL and 7.6 mg/mL from Paenibacillus [Citation64], Rhodothermus marinus [Citation65], Streptomyces ipomea [Citation50] and Bacillus subtilis [Citation66], respectively, and that of immobilized β-mannanase as 0.122 mg/mL for Clitocybe geotropa [Citation53] and 6.7 mg/mL for Bacillus sp. CFR1601 [Citation54].
Effect of metal ions and inhibitors
displays the impact of different metal ions and inhibitors on free and nanoparticle-immobilized β-mannanase activities. Both the free and immobilized β-mannanase activities were not inhibited by the metal ions Ca2+ and Mg2+, but were strongly inhibited by Cu2+, Mn2+and Ni2+. Previous studies have documented non-inhibition of free β-mannanase activity of Bacillus sp. N16-5 [Citation67], Streptomyces tendae [Citation68] and Vibrio sp. MA-138 [Citation63] by Ca2+, Mg2+ and Mn2+ and immobilized β-mannanase activity of Bacillus sp. CFR1601 [Citation54] by Cu2+ and Ni2+. A strong inhibition in both free and immobilized enzyme activities was also recorded with EDTA and PMSF, suggesting that the β-mannanase of Alg-S25 is a metalloenzyme and contains a serine group at the active site. Free β-mannanase activity of Paenibacillus cookie [Citation55], Streptomyces tendae [Citation68] and Streptomyces sp. CS147 [Citation69] was also strongly inhibited by EDTA. Similarly, an inhibition in the activity of Paenibacillus cookie was noted with PMSF [Citation55]. Furthermore, the non-inhibition of the enzyme activities by the thiol-group activator DTT and strong inhibition by thiol-group inhibitor iodoacetic acid suggested the participation of a –SH (sulfhydryl) group at the active site of β-mannanase extracted from the novel Streptomyces sp. Alg-S25. Similarly, the β-mannanase extracted from Paenibacillus cookie [Citation55] and Streptomyces sp. Alg-S23 [Citation43] was documented to contain a –SH group at the active site.
Table 2. Influence of metal ions and inhibitors on free and nanoparticle-immobilized β-mannanase activity.
Reusability
illustrates the successive reusability of nanoparticle-immobilized β-mannanase of Streptomyces sp. Alg-S25. The immobilized enzyme was stable until the 8th cycle by retaining more than 93% of the β-mannanase activity. After the 9th cycle, there was some loss in activity and at the 15th cycle the immobilized enzyme lost 80% of the maximal activity. The successive reuse of nanoparticle-immobilized β-mannanase indicates the biocatalytic potential of immobilized enzyme on economical processing of polymeric mannan.
Conclusions
Exploring β-mannanase-producing microbial biocatalysts from diverse ecosystems is paramount because of the biotechnological applications of this enzyme in food, fodder, detergent, pharmaceutical, textile and biorefinery industries and high cost of commercial β-mannanase. Immobilization of enzymes on chitosan nanoparticles has been receiving significant attention as an economical approach to enhance the stability and reusability of biocatalysts in industrial bioprocesses. The β-mannanase extracted from a novel Streptomyces sp. Alg-S25 was immobilized onto functionalized chitosan nanoparticles. The immobilized β-mannanase displayed enhanced thermal stability and reusability, tolerance to elevated concentrations of salts and strong affinity toward the substrate. In view of these promising characteristics, the chitosan nanoparticle-immobilized β-mannanase has the potential for use in the environmentally friendly bioconversion of mannan-enriched agro-industrial and seaweed wastes into value-added products, including second/third-generation biofuels and health-promoting mannooligosaccharides.
Disclosure statement
No potential conflict of interest was reported by the author.
Additional information
Funding
References
- Menon V, Rao M. Trends in bioconversion of lignocellulose: biofuels, platform chemicals & biorefinery concept. Prog Energ Combust. 2012;38(4):522–550.
- De Bhowmick G, Sarmah AK, Sen R. Lignocellulosic biorefinery as a model for sustainable development of biofuels and value added products. Bioresour Technol. 2018;247:1144–1154.
- Paone E, Tabanelli T, Mauriello F. The rise of lignin biorefinery. Curr Opin Green Sustain Chem. 2020;24:1–6.
- Ragauskas AJ, Williams CK, Davison BH, et al. The path forward for biofuels and biomaterials. Science. 2006;311(5760):484–489.
- Sanderson K. Lignocellulose: a chewy problem. Nature. 2011;474(7352):S12–S14.
- Moreira LR, Filho EX. An overview of mannan structure and mannan-degrading enzyme systems. Appl Microbiol Biotechnol. 2008;79(2):165–178.
- Saini JK, Saini R, Tewari L. Lignocellulosic agriculture wastes as biomass feedstocks for second-generation bioethanol production: concepts and recent developments. 3 Biotech. 2015;5(4):337–353.
- Anwar Z, Gulfraz M, Irshad M. Agro-industrial lignocellulosic biomass a key to unlock the future bio-energy: a brief review. J Rad Res Appl Sci. 2014;7(2):163–173.
- Magalhães AI, Carvalho JC, Melo Pereira GV, et al. Lignocellulosic biomass from agro-industrial residues in South America: current developments and perspectives. Biofuels Bioprod Bioref. 2019;13(6):1505–1519.
- Rodrigues ISV, Barreto JT, Moutinho BL, et al. Production of xylanases by Bacillus sp. TC-DT13 in solid state fermentation using bran wheat. Prep Biochem Biotechnol. 2020;50(1):91–97.
- Sánchez C. Lignocellulosic residues: biodegradation and bioconversion by fungi. Biotechnol Adv. 2009;27(2):185–194.
- Scheller HV, Ulvskov P. Hemicelluloses. Annu Rev Plant Biol. 2010;61:263–289.
- Van Dyk JS, Pletschke BI. A review of lignocellulose bioconversion using enzymatic hydrolysis and synergistic cooperation between enzymes-factors affecting enzymes, conversion and synergy. Biotechnol Adv. 2012;30(6):1458–1480.
- Yamabhai M, Ubol SS, Srila W, et al. Mannan biotechnology: from biofuels to health. Crit Rev Biotechnol. 2016;36(1):32–42.
- Malgas S, van Dyk JS, Pletschke BI. A review of the enzymatic hydrolysis of mannans and synergistic interactions between β-mannanase, β-mannosidase and α-galactosidase. World J Microbiol Biotechnol. 2015;31(8):1167–1175.
- Chauhan PS, Gupta N. Insight into microbial mannosidases: a review. Crit Rev Biotechnol. 2017;37(2):190–201.
- Saittagaroon S, Kawakishi S, Namiki M. Characterisation of polysaccharides of copra meal. J Sci Food Agric. 1983;34(8):855–860.
- Rodríguez-Gacio Mdel C, Iglesias-Fernandez R, Carbonero P, et al. Softening-up mannan-rich cell walls. J Exp Bot. 2012;63(11):3976–3988.
- Stiger-Pouvreau V, Bourgougnon N, Deslandes E. Carbohydrates from seaweeds. In: Fleurence J, Levine I, editors. Health and disease prevention. San Diego: Academic Press; 2016. p. 223–274.
- Chauhan PS, Puri N, Sharma P, et al. Mannanases: microbial sources, production, properties and potential biotechnological applications. Appl Microbiol Biotechnol. 2012;93(5):1817–1830.
- Jackson ME, Anderson DM, Hsiao HY, et al. Beneficial effect of beta-mannanase feed enzyme on performance of chicks challenged with Eimerla sp. and Clostridium perfringens. Avian Dis. 2003;47(3):759–763.
- Wu G, Bryant MM, Voitle RA, et al. Effects of beta-mannanase in corn-soy diets on commercial leghorns in second-cycle hens. Poult Sci. 2005;84(6):894–907.
- Li Y, Yang P, Meng K, et al. Gene, cloning, expression and characterization of a novel β-mannanase from Bacillus circulans CGMCC 1416. J Microbiol Biotechnol. 2008;18(1):160–166.
- Saeed M, Ayaşan T, Alagawany M, et al. The role of β-Mannanase (Hemicell) in improving poultry productivity, health and environment. Braz J Poult Sci. 2019;21(3):1–8.
- Sachslehner A, Foidl G, Foidl N, et al. Hydrolysis of isolated coffee mannan and coffee extract by mannanases of Sclerotium rolfsii. J Biotechnol. 2000;80(2):127–134.
- Jørgensen H, Sanadi AR, Felby C, et al. Production of ethanol and feed by high dry matter hydrolysis and fermentation of palm kernel press cake. Appl Biochem Biotechnol. 2010;161(1–8):318–332.
- Gübitz GM, Sachslehner A, Haltrich D. Microbial mannanases: substrates, production, and applications. In: Himmel ME, Baker JO, Saddler JN, editors. Glycosyl hydrolases for biomass conversion. ACS Symposium Series, Vol. 769. Washington, (DC): American Chemical Society; 2000. p. 236–262.
- Dhawan S, Kaur J. Microbial mannanases: an overview of production and applications. Crit Rev Biotechnol. 2007;27(4):197–216.
- Ademark P, Varga A, Medve J, et al. Softwood hemicelluloses-degrading enzymes from Aspergillus niger: purification and properties of a β-mannanase. J Biotechnol. 1998;63(3):199–210.
- Heux S, Meynial-Salles I, O'Donohue MJ, et al. White biotechnology: state of the art strategies for the development of biocatalysts for biorefining. Biotechnol Adv. 2015;33(8):1653–1670.
- Escamilla-Alvarado C, Pérez-Pimienta JA, Ponce-Noyolac T, et al. An overview of the enzyme potential in bioenergy-producing biorefineries. J Chem Technol Biotechnol. 2017;92(5):906–924.
- Leite RSR, Alves-Prado HF, Cabral H, et al. Production and characteristics comparison of crude β-glucosidases produced by microorganisms Thermoascus aurantiacus e Aureobasidium pullulans in agricultural wastes. Enzyme Microb Technol. 2008;43(6):391–395.
- Wilson DB. Cellulases and biofuels. Curr Opin Biotechnol. 2009;20(3):295–299.
- Dutra TR, Guimarães VM, Varela EM, et al. A Chrysoporthe cubensis enzyme cocktail produced from a low-cost carbon source with high biomass hydrolysis efficiency. Sci Rep. 2017;7(1):3893.
- Sheldon RA, van Pelt S. Enzyme immobilisation in biocatalysis: why, what and how. Chem Soc Rev. 2013;42(15):6223–6235.
- Sirisha V, Jain A, Jain A. Enzyme immobilization: an overview on methods, support material, and applications of immobilized enzymes. Adv Food Nutr Res. 2016;79:179–211.
- Homaei AA, Sariri R, Vianello F, et al. Enzyme immobilization: an update. J Chem Biol. 2013;6(4):185–205.
- Verma ML, Kumar S, Das A, et al. Chitin and chitosan-based support materials for enzyme immobilization and biotechnological applications. Environ Chem Lett. 2020;18(2):315–323.
- Ansari SA, Husain Q. Potential applications of enzymes immobilized on/in nano materials: a review. Biotechnol Adv. 2012;30(3):512–523.
- Verma ML, Puri M, Barrow CJ. Recent trends in nanomaterials immobilised enzymes for biofuel production. Crit Rev Biotechnol. 2016;36(1):108–119.
- Biró E, Németh AS, Sisak C, et al. Preparation of chitosan particles suitable for enzyme immobilization. J Biochem Biophys Methods. 2008;70(6):1240–1246. (2008)
- Klein MP, Nunes MR, Rodrigues RC, et al. Effect of the support size on the properties of β-galactosidase immobilized on chitosan: advantages and disadvantages of macro and nanoparticles. Biomacromolecules. 2012;13(8):2456–2464.
- Mohapatra BR. Comparative kinetic analysis of alginate lyase and mannanase co-produced via solid-state fermentation of cow dung supplemented with seaweed wastes by a novel Streptomyces sp. Alg-S23. Biomass Conv Bioref. 2020. DOI:10.1007/s13399-020-00658-5
- Hall TA. BioEdit: a user-friendly biological sequence alignment editor and analysis program for Windows 95/98/NT. Nucleic Acids Symp Ser. 1999;41:95–98.
- Kumar S, Stecher G, Tamura K. MEGA7: molecular evolutionary genetics analysis Version 7.0 for bigger datasets. Mol Biol Evol. 2016;33(7):1870–1874.
- Miller GL. Use of dinitrosalicylic acid reagent for determination of reducing sugar. Anal Chem. 1959;31(3):426–428.
- Sánchez-Ramírez J, Martínez-Hernández JL, Segura-Ceniceros P, et al. Cellulases immobilization on chitosan-coated magnetic nanoparticles: application for Agave atrovirens lignocellulosic biomass hydrolysis. Bioprocess Biosyst Eng. 2017;40(1):9–22.
- Mohapatra BR. Biocatalytic characteristics of chitosan nanoparticle-immobilized alginate lyase extracted from a novel Arthrobacter species AD-10. Biocatal Agric Biotechnol. 2020;23:101458.
- Collins SE, Lassalle V, Ferreira ML. FTIR-ATR characterization of free Rhizomucor meihei lipase (RML), Lipozyme RM IM and chitosan-immobilized RML. J Mol Catal B Enzym. 2011;72(3–4):220–228.
- Montiel M, Hernandez M, Rodriguez J, et al. Evaluation of an endo-beta-mannanase produced by Streptomyces ipomoea CECT 3341 for the biobleaching of pine kraft pulps. Appl Microbiol Biotechnol. 2002;58(1):67–72.
- Kansoh AL, Nagieb ZA. Xylanase and mannanase enzymes from Streptomyces galbus NR and their use in biobleaching of softwood kraft pulp. Antonie Van Leeuwenhoek. 2004;85(2):103–114.
- Arcand N, Kluepfel D, Paradis FW, et al. β-mannanase of Streptomyces lividans 66: cloning and DNA sequence of the manA gene and characterization of the enzyme. Biochem J. 1993;290(3):857–863.
- Nadaroglu H, Sonmez Z. Purification of an endo-beta 1,4-mannanase from Clitocybe geotropa and immobilization on chitosan coated magnetite nanoparticles: application for fruit juices digest. Dig J Nanomater Bios. 2016;11(2):685–697.
- Panwar D, Kaira GS, Kapoor M. Cross-linked enzyme aggregates (CLEAs) and magnetic nanocomposite grafted CLEAs of GH26 endo-β-1,4-mannanase: improved activity, stability and reusability. Int J Biol Macromol. 2017;105(Pt 1):1289–1299.
- Yin LJ, Tai HM, Jiang ST. Characterization of mannanase from a novel mannanase-producing bacterium. J Agric Food Chem. 2012;60(25):6425–6433.
- Srivastava PK, Kapoor M. Recombinant GH-26 endo-mannanase from Bacillus sp. CFR1601: biochemical characterization and application of partially hydrolysed guar gum. LWT-Food Sci Technol. 2015;64(2):809–816.
- Blibech M, Chaari F, Bhiri F, et al. Production of manno-oligosaccharides from locust bean gum using immobilized Penicillium occitanis mannanase. J Mol Catal B Enzym. 2011;73:111–115.
- Zhang R, Zhou J, Gao Y, et al. Molecular and biochemical characterizations of a new low-temperature active mannanase. Folia Microbiol (Praha). 2015;60(6):483–492.
- Srivastava PK, Rao ARA, Kapoor M. Structural insights into the thermal stability of endo-mannanase belonging to family 26 from Bacillus sp. CFR1601 (580.2). FASEB J. 2014;28:580–582.
- Regmi S, Yoo HY, Choi YH, et al. Prospects for bio-industrial application of an extremely alkaline mannanase from Bacillus subtilis subsp. inaquosorum CSB31. Biotechnol J. 2017;12(11):1700113.
- Marangoni AG. Enzyme kinetics: a modern approach. Hoboken (NJ): Wiley; 2003.
- Hanefeld U, Gardossi L, Magner E. Understanding enzyme immobilisation. Chem Soc Rev. 2009;38(2):453–468.
- Tamaru Y, Araki T, Amagoi H, et al. Purification and characterization of an extracellular beta-1,4-mannanase from a marine bacterium, Vibrio sp. strain MA-138. Appl Environ Microbiol. 1995;61(12):4454–4458.
- Chandra MRS, Lee YS, Park IH, et al. Isolation, purification and characterization of a thermostable β-mannanase from Paenibacillus sp. DZ3. J Korean Soc Appl Biol Chem. 2011;54(3):325–331.
- Politz O, Krah M, Thomsen K, et al. A highly thermostable endo-(1,4)-beta-mannanase from the marine bacterium Rhodothermus marinus. Appl Microbiol Biotechnol. 2000;53(6):715–721.
- Jiang Z, Wei Y, Li D, et al. High-level production, purification and characterization of a thermostable β-mannanase from the newly isolated Bacillus subtilis WY34. Carbohydr Polym. 2006;66(1):88–96.
- Ma Y, Xue Y, Dou Y, et al. Characterization and gene cloning of a novel beta-mannanase from alkaliphilic Bacillus sp. N16-5. Extremophiles. 2004;8(6):447–454.
- Yoo HY, Pradeep GC, Kim SW, et al. A novel low-molecular weight alkaline mannanase from Streptomyces tendae. Biotechnol Bioprocess Eng. 2015;20(3):453–461.
- Yoo HY, Pradeep GC, Lee SK, et al. Understanding β-mannanase from Streptomyces sp. CS147 and its potential application in lignocellulose based biorefining. Biotechnol J. 2015;10(12):1894–1902.