Abstract
In this work, we reported production of glucansucrases with molecular weights of about 300 kDa by strains of fructophilic lactic acid bacteria (FLAB) Lactobacillus pentosus AG8, Lactobacillus kunkeei AG9, AG10 and AG11 isolated from the gut of honey bee Apis mellifera. From sucrose, these enzymes synthesize average to high-molecular-weight dextran-type glucans ranging from 8.5 × 105 to >4 × 106 Da and containing 16 to 22% α-(1→3)-linked glucose units, with 5 to 8% branching in their structures. Enzyme preparations from the strains were applied in the synthesis of glucooligosaccharides (GOSs) with degrees of polymerization (DP) from 3 to 7 using maltose/sucrose ratios (M/S) from 8 to 0.12. The yields and composition of the obtained oligosaccharide products were strongly influenced by the ratios of the acceptor and donor of glucose units in the reactions. The highest yields of GOSs (>50 g/L) were achieved at M/S = 1, and the lowest content of α-(1→6) linkages (71%) combined with increased proportion of the products with DP > 3 were determined at M/S = 0.5. GOSs preparations from these two ratios were able to maintain the growth and acids production of three probiotic strains of lactobacilli, suggesting potential application as prebiotics.
Introduction
In the recent years, the microbial communities associated with economically important insects such as the western honey bee Apis mellifera are showing significant scientific interest. This could be attributed to the better understanding of the importance of the symbiotic relationships between the honey bees and their gastrointestinal microflora which play a substantial role in the well-being of these natural pollinators. On the other hand, there is a growing demand for isolation of new niche-specific microorganisms having beneficial properties that could be applied as probiotics, source of enzymes and other bio-active substances benefiting both humans and bees [Citation1–5]. One important group of microorganisms associated with the gut and the ecological niche of honey bee Apis mellifera is this of lactic acid bacteria (LAB) [Citation6, Citation7]. The substrates rich in sugars and mainly in fructose, such as fruits and flowers, play a selective role for differentiation of a specific group of LAB called fructophilic lactic acid bacteria (FLAB) [Citation8].
Fructophilic LAB have heterofermentative metabolism and on the basis of specific differences in their fermentative and 16S rDNA profiles, they were divided into obligate and facultative fructophilic lactic acid bacteria. To the first subgroup were included Lactobacillus kunkeei and several Leuconostoc spp. which were reclassified into the new genus Fructobacillus, namely F. fructosus, F. durionis, F. ficulneus and F. pseudoficulneus [Citation9]. Lb. florum and Lb. brevis isolated from honey bees and flowers were classified into the facultative FLAB subgroup [Citation10, Citation11]. A characteristic property that distinguishes the facultative FLAB is their ability to grow anaerobically on glucose media in the absence of external electron acceptors, and to produce one order of magnitude more ethanol in comparison to obligate FLAB such as Lb. kunkeei [Citation10, Citation12].
Lactic acid bacteria belonging to genera Lactobacillus, Leuconostoc, Weissella and Streptococcus are well-known for their beneficial properties as probiotics and starter cultures in the production and preservation of foods. In addition, many species of these bacteria are able to produce exopolysaccharides (EPSs) which find application as prebiotics, immunomodulators, blood volume expanders, main compounds or additives to new materials [Citation13–17]. An especially important group of LAB-derived EPSs is the glucose homopolysaccharides synthesized by a special family of α-retaining non-Leloir-type sucrases called glucansucrases which transfer glucose residues from sucrose to the growing chain of the synthesizing α-glucan polysaccharide [Citation13, Citation18]. Currently, there are more than 60 glucansucrase enzymes which were characterized biochemically and classified in the glycoside hydrolase family 70 (http://www.cazy.org) on the basis of their structure and mechanism of action [Citation19, Citation20]. Depending on the linkages between the residues in the synthesized glucans, there are four well-studied types of glucansucrases, namely: i) dextransucrases (EC 2.4.1.5), producing dextran containing >95% α-(1→6) linkages; ii) mutansucrases (EC 2.4.1.5), synthesizing mutan polysaccharide composed of >50% α-(1→3)-linked glucose units; iii) reuteransucrases (EC 2.4.1.5), synthesizing reuteran containing >50% α-(1→4) linkages in the main chain; iv) alternansucrases (EC 2.4.1.140), synthesizing a polysaccharide composed of alternating α-(1→6) and α-(1→3)-linked glucose units called alternan [Citation13, Citation18, Citation20].
In addition to their ability to synthesize high-molecular-weight glucans, glucansucrases from LAB are able to transfer competitively the glucose moiety of sucrose to suitable low-molecular-weight acceptors, such as maltose, isomaltose, lactose, raffinose, glucose and fructose. Thus they generate a series of short-chain GOSs having a prebiotic potential, which depends on their structure and composition, and is tightly related to the specificity of the enzymes used in the synthesis reactions [Citation21–23]. Presently, the low-molecular-weight GOSs having branched structures and α-(1→2) or α-(1→3)-linked glucose residues are considered to have more pronounced prebiotic effects due to their ability to induce a broader spectrum of glycoside hydrolase enzymes in the probiotic bacteria inhabiting the host’s gut [Citation24]. Some of the well-studied glucansucrases from LAB which are able to synthesize GOSs containing specific glyosidic linkages and have a practical application in their production are dextransucrases DSR-S and DSR-E from L. mesenteroides NRRL B-512F and L. citreum NRRL B-1299, respectively; alternansucrase from L. citreum NRRL B-21297 and reuteransucrase GTF-A from Lb. reuteri 121 [Citation25–28].
In our previous study, we reported the isolation of two new strains of FLAB Lb. kunkeei which produce high-molecular-weight glucansucrases that are able to synthesize branched glucans form sucrose [Citation29]. This and the other research mentioned above underline the importance of the honey bee’s gut microbiota both as means by which this economically important insect adapts to its ecological niche, and as a new source of biologically active molecules such as the glucansucrase enzymes. In addition, there is a growing demand for new molecular tools by which to achieve a cost-effective production of polysaccharides, prebiotic GOSs and glycoconjugates with new properties. This makes the habitats of the honey bees, which are rich in sugars, as well as their gastrointestinal tract a tempting source of useful carbohydrate-acting biocatalysts.
In the present study, we report the production of glucansucrases by four FLAB strains isolated from the gut of honey bees Apis mellifera. In addition, partially purified enzyme preparations from the strains were applied in the synthesis of glucan polysaccharides, whose molecular weights and structures were determined. The ability of the enzymes to catalyze acceptor reactions synthesizing GOSs and their prebiotic potential was also studied.
Materials and methods
Bacterial strains and cultural conditions
Fructophilic LAB strains Lb. pentosus AG8, Lb. kunkeei AG9, AG10 and AG 11 were obtained from the culture collection of the Department of Biochemistry and Microbiology, Plovdiv University (Plovdiv, Bulgaria). The strains were identified prior to this study on the basis of their carbohydrate fermentation profiles (API CH50®) and 16S rDNA sequencing and comparison [Citation30]. The strains were maintained weekly on fructose yeast extract polypeptone (FYP10) broth, containing: 100 g/L D-fructose; 10 g/L yeast extract; 5 g/L polypeptone; 2 g/L anhydrous sodium acetate; 0.5 g/L Tween 80; 0.2 g/L MgSO4.7H2O; 0.01 g/L MnSO4.4H2O; 0.01 g/L FeSO4.7H2O; 0.01 g/L NaCl, pH 6.8 at 30 °C, and were also stored in 20% glycerol (v/v) at −20 °C [Citation8]. For production of glucansucrases, the studied FLAB strains and the reference strain of L. mesenteroides subsp. mesenteroides NCIMB 8023 (purchased from National Bank for Industrial Microorganisms and Cell Cultures, Sofia, Bulgaria, and known also as ATCC 8293) were cultivated aerobically on a rotary shaker (200 rpm) in Dols medium containing: 40 g/L sucrose; 20 g/L yeast extract; 20 g/L K2HPO4; 0.2 g/L MgSO4.7H2O; 0.01 g/L MnSO4.H2O; 0.01 g/L NaCl; 0.01 g/L CaCl2; 0.01 g/L FeSO4.7H2O; pH 6.9 at 30 °C for 10 to 12 h [Citation31]. Probiotic strains Lb. plantarum S26, Lb. brevis S27 and Lb. sakei S16 were obtained from the bacterial culture collection of the Department of General and Industrial Microbiology, Sofia University, Bulgaria, and were routinely grown in de Man, Rogosa and Sharpe (MRS) medium (Biokar Diagnostics, France) at 37 °C.
Quantitative determination of metabolites
The amounts of lactic acid, acetic acid and ethanol produced during the anaerobic cultivation of probiotic strains Lb. plantarum S26, Lb. brevis S27 and Lb. sakei S16 in media supplemented with GOSs/glucose were determined enzymatically with the following commercially available kits (Megazyme, International Ireland Ltd., Wicklow, Ireland): i) K-DLATE – for determination of lactate; ii) K-ACET – for determination of acetate; iii) K-ETOH – for determination of ethanol. All the analyses for determination of metabolites were performed in duplicate from different experiments with standard deviations (±SD).
Partial purification and concentration of glucansucrases
The bacterial cells were separated from culture media by centrifugation (10000 g, 4 °C, 20 min). The cell pellet was washed twice in 20 mmol/L acetate buffer (pH 5.4) and re-suspended in the initial volume of buffer for further measurement of the cell-wall-bound glucansucrase activity and in situ analysis. To ensure the total absence of cells, the cultural liquids were filtered through 0.2 µm membranes. Extracellular glucansucrases were then concentrated and partially purified by addition of polyethylene glycol with molecular mass 1500 (PEG 1500) to a final concentration of 200 g/L. This approach achieved a two-phase partitioning between the native polysaccharides and the PEG-rich phase containing glucansucrases [Citation32]. The enzyme fraction was then pelleted by centrifugation (8500 g, 4 °C, 20 min). The obtained enzyme fraction from each isolate was used in the in situ analysis, polysaccharide and GOSs synthesis.
Assay of glucansucrase activity
One unit of glucansucrase activity is defined as the amount of enzyme catalyzing the release of 1.0 µmol fructose per minute at 30 °C in 20 mmol/L acetate buffer, pH 5.4; 0.05 g/L CaCl2 and 100 g/L of sucrose. Glucansucrase activity was determined by measuring the amount of reducing sugars derived from sucrose using 3,5-dinitrosalicylic acid method [Citation31, Citation33]. Additionally, released fructose was determined enzymatically (Cat. K-FRUGL, Megazyme, International Ireland Ltd., Wicklow, Ireland). Protein concentration was measured by the method of Bradford [Citation34] against bovine serum album standard curve. All the analyses for determination of residual sugars and protein concentrations were performed in duplicate from different experiments with standard deviations (±SD).
Electrophoresis analysis (SDS-PAGE)
Sodium dodecyl sulfate polyacrylamide gel electrophoresis (SDS-PAGE) analysis using polyacrylamide 70 × 80 slab gels (3% concentrating; 5% separating gel) was performed according to the method of Laemmli [Citation35]. In situ activity detection of glucansucrases was performed by incubation of the gels in 100 g/L sucrose, 20 mmol/L acetate buffer, 0.05 g/L CaCl2 overnight, followed by staining the synthesized polysaccharides according to a periodic acid-Schiff’s protocol [Citation36]. The protein standards (High Molecular Weight Calibration Kit for SDS Electrophoresis, Amersham, UK) were stained with 1.0 g/L Coomassie Brilliant Blue R 250 in 5% (v/v) acetic acid.
Synthesis and analysis of glucan polysaccharides
Glucansucrase preparation from a FLAB strain was added to 100 g/L sucrose in 20 mmol/L acetate buffer (pH 5.4) containing 0.05 g/L CaCl2 to a final enzyme activity of 0.5 U/mL. To each reaction mixture was added 0.01% (w/v) sodium azide to prevent microbial growth. Reactions were incubated at 30 °C with shacking (100 rpm) for 48 h. The depletion of sucrose was monitored by high performance liquid chromatography (HPLC) as described previously [Citation37]. The reactions were stopped by addition of equal volume of 96% ethanol to each mixture which led to denaturation of the enzyme and precipitation of the synthesized glucan. The polysaccharides were pelleted (9000 g, 4 °C, 20 min), washed two more times with ethanol, dissolved in ultra-pure water and freeze-dried (FreeZone® PlusTM 4.5 Liter Cascade, Labconco Corporation, Kansas City, USA).
The molecular mass of the purified glucans was determined by size-exclusion chromatography (HPSEC) using column Shodex OHpak SB-805, 8 × 300 mm, equipped with OH SB-G 6B guard column. The mobile phase was ultra-pure water containing 0.45 mmol/L NaNO3; 0.6 mL/min flow rate; 30 °C; 20 µL injection volume. The detection was performed with a refractive index detector RID-560 (KONIK, Barcelona, Spain). The molecular mass of the glucans was calculated against a standard curve built with dextran standards of 12, 50, 70, 150, 500, 670 and 1360 kDa (Sigma). All the analyses for molecular mass determination of the glucans were performed in triplicate and average values are given.
The linkages in the purified polysaccharides and their distribution were determined by Nuclear magnetic resonance (NMR) spectroscopy on Avance II (Bruker, Billerirrica, Massachusetts, USA) as described before [Citation38]. Samples (10 mg) were dissolved in 0.5 mL D2O and specters were recorded at 500 MHz and 125 MHz for 1H and 13C NMR analyses, respectively using 5 mm z-gradient TBI (triple resonance broadband inverse) probe. 1H and 13C chemical shifts (δ) were expressed in ppm by reference to internal acetone (δ = 2.225 ppm) and the methyl-carbon of internal acetone (δ = 31.08 ppm), respectively. The temperature of the analysis was 298 K. The data acquisition and processing was performed using TopSpin 3 software. The percentages of the linkages in glucans were calculated on the basis of the relative intensities of the anomeric protons or carbons.
Synthesis and analysis of GOSs
The reactions for synthesis of GOSs were performed at 30 °C in 20 mmol/L acetate buffer (pH 5.4) supplemented with 0.05 g/L CaCl2 and 0.01% (w/v) sodium azide. Sucrose served as a donor of glucose units and maltose monohydrate (Merck, Bulgaria EAD) as a sugar acceptor. The following ratios of maltose/sucrose were used: 1, 2, 4, 6, 8, 0.12, 0.16, 0.25 and 0.5. The final concentration of sugar substrates was 100 g/L, and each reaction was started by addition of glucansucrase preparation to 1.0 U per milliliter of reaction mixture. The course of the reactions was monitored by HPLC, and they were stopped when all sucrose was depleted (∼48 h). The further steps of processing and analysis of the synthesized GOSs were described previously [Citation37]. Briefly, polysaccharide fractions were precipitated with 96% ethanol, and GOSs fractions were subjected to vacuum evaporation, and then evaluated by HPLC analysis using YMC-PackTM Polyamine II column (YMCTM, Co., Ltd., Japan), 60:40% acetonitrile:water mobile phase at 30 °C. The remaining monosaccharides and maltose were removed by gel-filtration (Bio-Gel P2, fine mesh, Bio-Rad Laboratories, Inc., USA) through 5 × 150 cm column [Citation39]. GOSs preparations were then subjected to freeze-drying and used in the bacterial utilization and hydrolysis experiments. Synthesis reactions, HPLC analyses and the purification were carried out in duplicate and the results from different experiments are presented with a standard deviation (±SD).
Enzymatic hydrolysis of GOSs
Hydrolysis reactions of purified GOSs were performed as described before [Citation37]. Briefly, 1% (w/v) GOSs solution in 20 mmol/L acetate buffer (pH 5.4) was incubated with 1.0 U/mL endodextranase (EC 3.2.1.11) (Sigma – Aldrich, Chemie, Germany) for 24 h at 30 °C. As a control was used 1% (w/v) isomaltose. One unit endodextranase activity is defined as the amount of enzyme catalyzing the hydrolysis of 1.0 µmol isomaltose per minute at the above-mentioned reaction conditions [Citation39]. The reaction products were analyzed by HPLC. The hydrolysis of GOSs was carried out in triplicate and HPLC analyses were performed in duplicate, and the results from different experiments with standard deviations (±SD) are presented.
Utilization of GOSs by probiotic lactobacilli
The overall experimental settings were described in full before [Citation37]. Briefly, washed Lb. plantarum S26, Lb. brevis S27 and Lb. sakei S16 cells were inoculated into modified MRS medium containing 10 g/L glucose or filter-sterilized GOSs. The fermentations were carried out in BBL® Gas Pack anaerobic system (Becton Dickinson, Sparks, USA) at 37 °C for 48 h, without pH control. GOSs utilization was estimated by measurement of bacterial growth, calculation of the specific growth rate (µ), changes in pH and production of lactate, acetate and ethanol by the strains. Each GOSs utilization experiment was performed in triplicate, and the results from different experiments with standard deviations (±SD) are presented.
Statistical analysis
Programmable scientific calculator “CASIO” fx-991ES Plus, statistical software package SigmaPlot v12.0 (Systat Software, Inc., Chicago, USA) and Microsoft Excel (version 2010, SP2) were used for data analysis and graphical representation. Data from the performed experiments were subjected to one-way analysis of variance (ANOVA) and the statistical significance was set at a level of confidence p < 0.05.
Results
Detection of glucansucrases by in situ SDS-PAGE
In order to detect and determine the molecular weights and distribution of glycansucrases produced by FLAB strains AG8, AG9, AG10 and AG11 on sucrose media, in situ SDS–PAGE of extracellular and cell-associated fractions was performed. As controls were used enzyme preparations from a reference strain L. mesenteroides ATCC 8293, which is a well-known producer of several types of glycansucrase enzymes () [Citation40].
Figure 1. In situ SDS-PAGE analysis of extracellular and cell-associated glycansucrases from FLAB strains AG8, AG9, AG10 and AG11. M, protein standard (Amersham High Molecular Weight Calibration Kit for SDS Electrophoresis, GE Healthcare. Cat. 17-0615-01); (A) Lane 1, extracellular fraction of AG8; Lane 2, extracellular fraction of AG9; Lane 3, extracellular fraction of AG10; Lane 4, extracellular fraction of AG11; Lane 5, extracellular fraction of L. mesenteroides ATCC 8293. (B) Lane 1, cell-associated fraction of AG8; Lane 2, cell-associated fraction of AG9; Lane 3, cell-associated fraction of AG10; Lane 4, cell-associated fraction of AG11; Lane 5, cell-associated fraction of L. mesenteroides ATCC 8293.
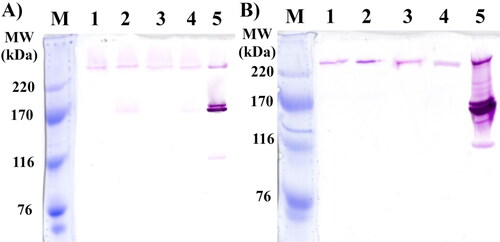
The in situ analysis of enzyme preparations from strains AG8, AG9, AG10 and AG11 showed that all of them produce extracellular and cell-associated glucansucrases with molecular weights of about 300 kDa (). In addition, activity bands of about 180 kDa were detected only in the extracellular fractions of strain AG9 and AG11 (, lanes 2 and 4). The referent strain L. mesenteroides ATCC 8293 also showed such bands in both extracellular and cell-associated enzyme fractions, and they correspond to dextransucrase. This strain is also known as a producer of fructansucrase with a molecular weight of about 120 kDa, but such activity was not detected in the extracellular and cell-associated enzyme profiles of the studied fructophilic strains ().
Analysis of glucans synthesized by FLAB
The size-exclusion chromatography analysis of the glucans revealed that strains Lb. pentosus AG8 and Lb. kunkeei AG10 produce polymers with molecular weights of about 9.4 × 105 Da and 1.1 × 106 Da, respectively. For the polysaccharide preparations produced by glucansucrases from strains Lb. kunkeei AG9 and AG11, fractions with molecular weights of about 8.5 × 105 and 9.8 × 105, respectively, were detected. Additionally, these two strains produced glucan fractions that exceeded the exclusion limit of the column used, so they have molecular weights greater than 4 × 106. These high-molecular polysaccharide fractions, which represent about 8% of the area of the signals, correlate well with the detection of additional 180 kDa activity bands in the extracellular enzyme preparations from strains Lb. kunkeei AG9 and AG11.
The analyses of the 1H part of NMR spectra showed chemical shifts at 5.31 ppm and 4.94 ppm corresponding to D-Glcp residues bound with α-(1→3) and α-(1→6) linkages, respectively. Anomeric protons with these chemical shifts were detected in the glucan samples from the four fructophilic strains (). These results were further confirmed by the obtained 13C spectra of the analyzed glucans (). The signal at 101.18 ppm was assigned to the anomeric carbon involved in the formation of α-(1→3) linkages, and the chemical shift at 98.98 ppm was assigned to the anomeric carbon involved in the formation of α-(1→6) linkages between D-Glcp residues. In addition, the presence of a signal at 84.47 ppm, corresponding to C3 involved directly in the formation of α-(1→3) linkages, further confirms the explanation of the results (). The presence of 1H NMR signal at 4.98 ppm and of 13C NMR signal at 98.73 ppm indicated the presence of 3,6-di-O-substituted α-D-Glcp residues and branched structure of the analyzed glucans, ranging from 5 to 8%. The obtained spectra of the studied glucans correlate well with the ones reported previously by Bounaix et al. [Citation41]. On the basis of the obtained NMR spectra, the highest content of α-(1→3) linkages was calculated in the glucan of Lb. pentosus AG8 (22%), followed by these of Lb. kunkeei AG10 (20%), Lb. kunkeei AG9 (18%) and Lb. kunkeei AG11 (16%), respectively.
Synthesis of GOSs by glucansucrases from FLAB
In order to evaluate the ability of glucansucrases from the studied strains to produce GOSs, we performed a series of synthesis reactions using various maltose/sucrose ratios. A summary of the obtained yields, their distribution by degree of polymerization (DP) and the overall profiles that differentiate the expressed glucansucrase inventory of strains Lb. pentosus AG8 and Lb. kunkeei AG9, AG10 and AG11 is presented in .
Figure 4. Synthesis of GOSs by extracellular glucansucrases from strains AG8 (A), AG9 (B), AG10 (C) and AG11 (D) in the presence of different maltose/sucrose ratios.
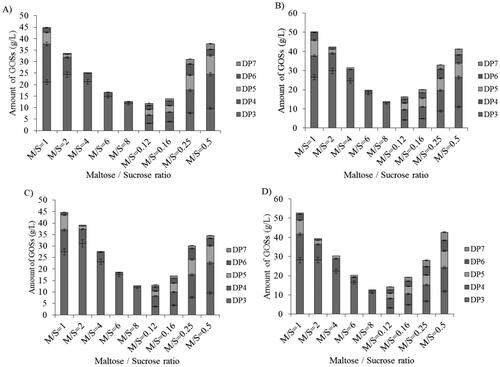
The synthesis reactions generated a series of GOSs with DP ranging from 3 to 7 and distribution of the yields that is strongly affected by the maltose/sucrose (M/S) ratios. In all the cases, the highest yields of GOSs were obtained at M/S = 1, and the products with DP3 were the predominant oligosaccharide fraction − 47.15, 52.9, 61.8 and 53.43% of the total amounts of the synthesized GOSs by the enzymes from strains AG8, AG9, AG10 and AG11, respectively (). The increase in the M/S ratios resulted in a progressive decrease in the proportion of GOSs having DP > 3 and also their total quantities, which at M/S = 8 were between 70 and 75% lower compared to those synthesized at M/S = 1. On the other hand, at lower M/S ratios where sucrose is the prevalent sugar at the start of the reactions, we recorded a more even distribution of the fractions according to their DP. Under these conditions, DP3 products served as efficient acceptors of glucose units from sucrose leading to a decrease in their proportion at the expense of the accumulation of DP4 GOSs, which are the largest fraction at M/S ratios from 0.12 to 0.5 (), and representing between 29% and 39% of the total amount of the synthesized oligosaccharides. In contrast to the reactions with increasing M/S ratios, where maltose competes more efficiently for the glucose residues in the less available sucrose, which leads to gradual elimination of GOSs with DP > 3, all the oligosaccharides synthesized at M/S ratios <1 contained products with DP from 3 to 7. Their amounts and the overall production of GOSs increased towards M/S = 0.5 where the highest production of GOSs was achieved with DP > 3 − 15.77, 21.84, 31.67 and 20.41%, respectively for the reactions driven by glucansucrases from strains AG8, AG9, AG10 and AG11, compared to the yields at M/S = 1.
An interesting feature that distinguished the oligosaccharide profiles of strains AG9 and AG11 is the production of DP7 GOSs at M/S = 1: respectively, 0.5 and 1.14% of the total amount of oligosaccharides produced by the strains. In addition, DP5 products at M/S = 6 were detected only in the profiles of these two strains: 1.54 and 4.68% of the total amount of GOSs, respectively. These differences, compared to the oligosaccharide profiles of strains AG8 and AG10 correlate well with the detection of two extracellular glucansucrases produced by strains AG9 and AG11 ().
Enzymatic hydrolysis of GOSs synthesized by glucansucrases from FLAB
The content of α-(1→6) linkages in the GOSs synthesized by glucansucrases from strains AG8, AG9, AG10 and AG11 was evaluated by subjecting the obtained preparations to endodextranase hydrolysis and determination of the amounts of the released glucose ().
Figure 5. Distribution profiles of α-(1→6) linkages in GOSs synthesized by glucansucrases from strains Lb. pentosus AG8, Lb. kunkeei AG9, AG10 and AG11.
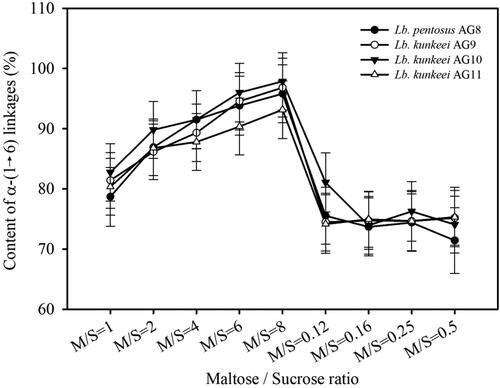
The highest content of α-(1→6)-linked glucose units in the synthesized acceptor products was determined at M/S = 8 and ranges from 93.16% (AG11) to 96.86% (AG10). This correlates well both with the decrease of sucrose concentration from M/S = 1 to M/S = 8 and the fractions of GOSs having DP > 3. In addition, this suggests that at increasing M/S ratios where maltose is the predominant acceptor sugar in the reaction mixtures, glucose residues are transferred to it forming mostly α-(1→6) linkages, instead to GOSs having DP > 3 which in turn are less efficient and less competitive acceptors of the of glucose units available in the scarce amounts of sucrose. At M/S = 0.12 where the amount of maltose was the lowest tested, there was a significant decrease in the content of α-(1→6) linkages in the synthesized GOSs (). For the enzyme preparations tested at this M/S ratio, the content of α-(1→6) linkages ranged from 74.15% to (AG11) to 81.60% (AG10). The further increase in the M/S ratios to 0.5 while keeping the concentration of sucrose prevalent, led only to an increase in the overall yield of GOSs with no significant change in the content of α-(1→6) linkages in the products ( and ). At M/S = 0.5, the lowest content of α-(1→6) linkages was detected in the GOSs preparations of isolate AG8 − 71.42% (). All in all, at decreasing M/S ratios where the maltose was less abundant, more of the available glucose units in the sucrose were transferred to acceptor products having DP > 3. This led to a decrease in the amount of α-(1→6)-transferred glucose units, suggesting formation of different linkages in the acceptor GOSs products. This trend correlated well with the observation that, at M/S ratios from 0.12 to 0.5, the amount of GOSs with DP3 was lower than that of DP4 products, suggesting a competitive transfer of glucose units to DP3 acceptors, which diminishes their amount in favor of the accumulation of DP4 GOSs ().
As seen, the profiles of the content of α-(1→6) linkages in GOSs synthesized by enzyme preparations from the four FLAB strains were very similar. This suggested more general effects of M/S ratios on the linkages specificity of the studied glucansucrases ().
Utilization and prebiotic potential of GOSs synthesized by glucansucrases from FLAB
Strains of Lb. plantarum S26, Lb. brevis S27 and Lb. sakei S16, which are known to exhibit probiotic properties [Citation42, Citation43], were grown anaerobically in media supplemented with GOSs preparations synthesized at M/S = 1 and 0.5 (). All the strains demonstrated nearly identical specific growth rates (µ) during their cultivation in media with GOSs to the ones calculated in glucose media. The observed growth of the probiotic lactobacilli in the GOSs supplemented media showed strain-dependent features. The specific growth rates of Lb. sakei S16 were on average 39% lower than the ones of the other two probiotic strains independently of the carbohydrates supplemented in the media ().
Table 1. Specific growth rates of Lb. plantarum S26, Lb. brevis S27 and Lb. sakei S16 cultivated in media supplemented with maltose derived GOSs.
The calculated values of the specific growth rates during cultivation in media with GOSs or glucose correlated well with the heterofermentative profiles of the strains associated with acidification of the media due to the production of lactic acid, acetic acid and very little amounts of ethanol (). At the end of the cultivation of the strains (48 h), strain specific rations of the amounts of lactic acid to acetic acid produced were observed. For Lb. plantarum S26, the ratio is 4.1, and for the other two strains they are 1.4 and 0.8, respectively. This decrease of the amounts of the produced lactic acid at the expense of acetic acid was associated with the specific metabolic properties of the strains and was not directly related to the composition of the oligosaccharide preparations tested in the current study.
Table 2. Values of pH, lactate, acetate and ethanol after cultivation of Lb. plantarum S26, Lb. brevis S27 and Lb. sakei S16 in media containing GOSs.
Discussion
Previously, we have reported isolation of two Lb. kunkeei strains from the gastrointestinal tract of honey bee A. mellifera that produce extracellular glucansucrases synthesizing branched glucans [Citation29]. Here, we report isolation of four more FLAB strains producing glucans in sucrose media. According to the description made by Endo et al. [Citation12], Lb. kunkeei does not produce dextran from sucrose suggesting the lack of dextransucrase activity. In a previous study, we isolated two Lb. kunkeei strains producing glucansucrases with molecular weights of about 300 kDa and synthesizing branched dextrans [Citation29]. Vasquez et al. [Citation44] described the formation of EPS-containing biofilms in the honey stomach of honey bees that maintain the presence of FLAB and Lb. kunkeei as a predominant species in this organ. The analysis of the genome of Lb. kunkeei MP2 also revealed the presence of a gene encoding glucosyltransferase GtfC which is expressed in the presence of carbohydrates such as sucrose and glucose, and is responsible for the synthesis of a mixture of soluble and insoluble glucans playing a role in the biofilm formation [Citation45]. A genome-wide screening of Lb. kunkeei EFB6 identified glucansucrase BRS-D which catalyzes α-(1→2)-branching of external dextran using sucrose. Such branching sucrases are also identified in L. citreum NRRL B-1299 (BRS-A), L. citreum NRRL B-742 (BRS-B) [Citation46] and Lb. fallax KCTC3537 (BRS-C) [Citation5]. Here, we reported the isolation of four glucan-synthesizing FLAB producing glucansucrases with molecular weights of about 300 kDa. Currently, production of enzymes with such a high molecular mass is reported only for members of genus Leuconostoc. The most well studied among them is a 312 kDa dextransucrase DSR-E produced by L. citreum NRRL B-1299 which was cloned and characterized. This enzyme contains two catalytic domains: one responsible for formation of α-(1→6) linkages, and a second one that introduces α-(1→2) branches in synthesized glucans [Citation26, Citation47]. Production of enzymes of a similar size has been reported also for L. mesenteroides ATCC 8293 [40] and L. mesenteroides URE 13 [Citation38]. The gene for glucansucrase URE13-300 from the second strain was cloned and employed in the synthesis of branched GOSs [Citation37].
Extracellular glucansucrases from Lb. pentosus AG8, Lb. kunkeei AG9, AG10 and AG11 synthesize branched medium to high-molecular-weight dextrans containing 16–22% α-(1→3) linkages and 5–8% 3,6-branches. Depending on their source and specificity, glucansucrases from LAB produce glucans with different molecular weights and content of α-(1→6) linkages. Dextrans containing >50% α-(1→6) linkages usually display molecular mass in the range 106–108 Da [Citation14]. Dextransucrase DSR-S from L. mesentroides NRRL B-512F, which is used for production of commercial dextrans, synthesizes up to 106 Da dextran containing 95% α-(1→6) linkages and about 5% α-(1→3) linkages. Dextransucrase Gtf180 from Lb. reuteri 180 synthesizes branched α-glucan with molecular weight of 3 × 107 Da, and about 30% α-(1→3) linkages [Citation20]. Alternansucrase ASR from L. citreum NRRL B-1355 synthesizes two populations of glucans containing about 40% α-(1→3) linkages: i) around 107 Da, and ii) medium size polymers of 103–104 Da [Citation48]. Dextrans with low and average molecular weights have found significant application as volume expanders, infusion fluids, anticoagulants, iron carriers and vaccine adjuvants, and because of that the discovery of new glucansucrases able to produce directly such glucan fractions are of interest [Citation14]. In this regard, two dextransucrases from the genome of L. citreum NRRL B-1299 were cloned: 145 kDa DSR-M and 229 kDa DSR-DP synthesizing dextrans with molecular weights ranging from 580 to 27 × 103 Da. Most strikingly, DSR-DP was reported as the first dextransucrase introduced in the genome of LAB by phage-mediated horizontal gene transfer [Citation49].
An additional catalytic feature of the non-Leloir glucansucrases from LAB is the so-called acceptor reaction leading to the synthesis of low-molecular-weight GOSs having prebiotic properties [Citation49, Citation50]. In the present work, we reported for the first time employment of glucansucrases from FLAB isolated from honey bee A. mellifera in acceptor reactions for synthesis of GOSs. The highest yields of GOSs were obtained at M/S = 1, and the observed variations in the composition and quantity of the oligosaccharide preparations could be explained with the specific features of the acceptor reactions, which are function of the used catalyst, concentration of the acceptor molecule and its strength. Maltose was reported as one of the most potent sugar acceptors that is able to redirect significant part of the glucose units in sucrose to synthesis of low-molecular-weight GOSs, and thus suppressing the production of glucan polysaccharides [Citation51–53]. Robyt and Eklund [Citation52] reported synthesis of a homologous series of GOSs with DP up to 7 using dextransucrase DSR-S from L. mesentroides NRRL B-512F and M/S = 1. They observed a gradual decrease in the amount of GOSs correlating with the increase in their DP values. Notable exceptions were the DP4 products, which showed the highest yields, suggesting that the DP3 product (panose) is a better glucose acceptor compared to the products with higher DP. At equimolar initial concentrations of maltose and sucrose in the reactions, the authors reported that >75% of the glucose available in sucrose is included in GOS products [Citation52]. In the present work, between 74% (AG10) and 92% (AG11) of the glucose units available in sucrose were included in GOS products at M/S = 1, and these results are comparable to those discussed above, suggesting similar efficiency of the acceptor reactions catalyzed by the enzymes studied here.
Maltose was the strongest acceptor for synthesis of GOSs by alternansucrase from L. citreum NRRL B-1355. Using M/S = 0.42, Cote and Robyt [Citation54] reported the synthesis of GOSs with DP up to 4. The authors found that the first added glucose residue is α-(1→6)-linked to maltose, and α-(1→3), and more α-(1→6) products appear at DP4. The authors also reported yields of 84% GOSs [Citation54]. In comparison, at M/S = 0.5, we achieved lower yields of GOSs ranging from 52% (AG10) to 66% (AG11), but with more even distribution of the products up to DP7. Later studies of the maltose acceptor reactions by alternansucrase from L. citreum NRRL B-21297 revealed that at M/S = 1 the enzyme produces non-homogenous series of alternan-type GOSs with DP up to 8 [Citation27, Citation55]. Using glucansucrase preparation from L. citreum NRRL B-742 and M/S ratios 0.5 and 0.14, Remaud et al. [Citation39] reported the synthesis of α-(1→3)-branched GOSs with DP up to 7. They observed an increase in the proportion of branched GOSs with the increase of sucrose concentration [Citation39]. This correlates with our observation of decreased content of α-(1→6)-linked glucose units in GOSs synthesized at M/S < 1, but the differences between M/S ratios 0.12 to 0.5 are in the range of only 2 to 4%. Knowing that the enzyme preparations from the studied FLAB strains introduce α-(1→3)-branches in the synthesizing polysaccharides, we could presume retaining of this specificity in the acceptor products too. At M/S = 0.5, using cloned dextransucrase DSR-E, Bozonnet et al. [Citation47] reported the synthesis of α-(1→2)-branched R5 fraction with DP5. In previous studies concerning the synthesis of GOSs with dextransucrase preparation from L. citreum NRRL B-1299, Remaud-Simeon et al. [Citation49] revealed that the sucrose concentration and its ratio to maltose are crucial factors affecting the yields of α-(1→2) branched GOSs. The authors reported the highest yields of α-(1→2)-branched GOSs at M/S = 0.25, where the concentration of sucrose is prevalent. This also led to an increase in the proportion of the longer GOSs products containing α-(1→2) linkages [Citation49]. These findings correlate well with the results obtained in the present study and also with the ones for glucansucrase URE 13-300 from L. mesenteroides URE 13. Using maltose as an acceptor, this enzyme incorporates between 55% (M/S = 0.5) and 76% (M/S = 1) of the available glucose units from sucrose into GOSs [Citation37].
The ability of lactobacilli to metabolize prebiotic GOSs differs significantly from this of Bifidobacterium because their enzyme machinery for carbohydrate utilization is mostly intracellular and depends strongly on the presence and efficiency of the available mechanisms for transport of sugars [Citation56, Citation57]. In addition, the ability to utilize and ferment a certain type of GOSs is not tightly bound to a given Lactobacillus species but also to specific strains, and is often a result of acquisition of plasmids or horizontal gene transfer [Citation58, Citation59]. Despite that the evolution of lactobacilli was accompanied by reduction in the sizes of their genomes and tight specialization to ecological niches, such as the upper parts of the intestinal tract of humans and animals, they demonstrate remarkable abilities to utilize a variable range of specific carbohydrates which allow them to compete with other genera in the given habitat [Citation57, Citation60]. An interesting example of the effectiveness of the habitat adaptation on a genetic level are the Lb. plantarum strains which are referred to as ‘natural metabolic engineers’ due to the cassette organization of their carbohydrate utilization genes. These genes can be acquired, shuffled, substituted or deleted depending on the conditions of the particular ecological niche [Citation61].
Practically all lactobacilli are able to utilize α-glucosides, and amylopullulanase and amylase were identified as the only extracellular glycoside hydrolases participating in the metabolism of these substrates by hydrolysis resulting in the release of maltodextrins and other α-glucooligosaccharides [Citation57]. A particularly important role in the utilization of α-GOSs substrates is attributed to the four-component ATP-binding cassette (ABC) transport system MalEFG/MsmK, which is well characterized in Lb. acidophilus, Lb. sanfranciscensis and other lactobacilli [Citation62]. This transport system seems to be responsible for the uptake not only of maltose but also of longer α-GOSs, because a phosphotransferase system that could facilitate the transport of these carbohydrates has not been identified in lactobacilli [Citation57]. In addition, in Lb. acidophilus and Lb. casei strains intracelular amylopullulanases and maltose phosphorylase were identified, which were expressed from the same operon as MalEFG/MsmK ABC transporter and β-phosphoglucomutase, which together ensure entering of maltose and maltodextrins in the corresponding metabolic pathways. The identification of intracellular dextranase DexB in lactobacilli further contributes to the knowledge about the ability of these LAB to utilize GOSs containing α-(1→6) linkages, both natural or obtained by planned acceptor reactions using glucansucrase enzymes [Citation57, Citation63]. In the present work, we reported a strain-specific utilization of GOSs containing between 71% and 80% α-(1→6) linkages suggesting the presence of additional intracellular glycoside hydrolase enzymes that contribute to the hydrolysis of oligosaccharides different from the dextran-derived isomaltooligosaccharides. As we have shown above, the enzyme preparations from the studied FLAB strains produce natively glucans containing >10% α-(1→3) linkages, suggesting their presence in the maltose acceptor products too. In a previous work, Cote et al. [Citation53], and Sanz et al. [Citation64] reported poor utilization of α-(1→3)-containing GOSs by pure cultures of lactobacilli. Later studies on utilization of alternansucrase oligosaccharide preparations by microbiota of fecal samples and estimation of their prebiotic indices revealed that DP7 products contribute significantly to the growth of lactobacilli population [Citation65]. According to the authors, this could be due to a cooperative degradation of GOSs by other bacterial groups of the gut community or to the intrinsic properties of the given population of lactobacilli and its overall inventory of carbohydrate-acting enzymes.
The presented results about utilization of oligosaccharides with prebiotic potential by Lb. plantarum S26, Lb. brevis S27 and Lb. sakei S16 correlate well with our recent studies concerning the utilization of maltose, raffinose, lactose and xylose-derived oligosaccharides by these strains [Citation37, Citation43].
Conclusions
Considering the increasing demand for obtaining of new materials, the discovery of new natural biocatalysts that are able to fit the desired purpose of transforming comparatively cheap substrates into valuable commodities is trending. In the presented work, we reported application of four FLAB strains from the gut of honey bee A. mellifera for production of extracellular high-molecular-weight glucansucrases. This suggested that these inherently important insects, responsible for production of honey, bee bread and other beneficial products, and most significantly – pollinating the flowering crops all over the world, could be viewed also as a source of proven biotechnologically important enzymes such as glucansucrases. These enzymes were able to synthesize glucans containing >10% α-(1→3) linkages from sucrose, and also were employed into the synthesis of GOSs with DP up to 7 using maltose as an acceptor of glucose units. To the best of our knowledge, this work reports for the first time application of glucansucrases from fructophilic lactobacilli in the production of glucooligosaccharides via maltose acceptor reactions, and also testing of their prebiotic potential. In addition, the yields and composition of the acceptor products containing >20% assumed α-(1→3) linkages are influenced by the acceptor/donor ratio which is a promising opportunity for modulation of the acceptor reactions. Still, further studies employing the studied enzymes and other acceptor molecules remain to be performed in order to characterize the synthetic potential of these glucansucrases in full.
Acknowledgements
This work was supported by Department for Scientific Research – PU, Bulgaria, grant МУ19-БФ-019/2019, and by operational program “Science and education for smart growth” 2014–2020, grant number BG05M2OP001-1.002-0005-C01, Personalized Innovative Medicine Competence Centre (PERIMED).
Data availability statement
All data that support the findings reported in this study are available from the corresponding author upon reasonable request.
Disclosure statement
The authors have no conflicts of interest to disclose.
Additional information
Funding
References
- Berrios P, Fuentes JA, Salas D, et al. Inhibitory effect of biofilm-forming Lactobacillus kunkeei strains against virulent Pseudomonas aeruginosa in vitro and in honeycomb moth (Galleria mellonella) infection model. Benef Microbes. 2018;9(2):257–268.
- Pachla A, Wicha M, Ptaszyńska AA, et al. The molecular and phenotypic characterization of fructophilic lactic acid bacteria isolated from the guts of Apis mellifera L. derived from a Polish apiary. J Appl Genet. 2018;59(4):503–514.
- Khan KA, Al-Ghamdi AA, Ghramh HA, et al. Structural diversity and functional variability of gut microbial communities associated with honey bees. Microb Pathog. 2020; 138:103793.
- Romero S, Nastasa A, Chapman A, et al. The honey bee gut microbiota: strategies for study and characterization. Insect Mol Biol. 2019;28(4):455–472.
- Vuillemin M, Claverie M, Brison Y, et al. Characterization of the first α-(1→3) branching sucrases of the GH70 family. J Biol Chem. 2016;291(14):7687–7702.
- Endo A. Fructophilic lactic acid bacteria inhabit fructose-rich niches in nature. Microb Ecol Health D. 2012; 23(18563):6–9.
- Olofsson TC, Vasquez A . Detection and identification of a novel lactic acid bacterial flora within the honey stomach of the honeybee Apis mellifera. Curr Microbiol. 2008;57(4):356–363.
- Endo A, Futagawa-Endo Y, Dicks LMT. Isolation and characterization of fructophilic lactic acid bacteria from fructose-rich niches. Syst Appl Microbiol. 2009;32(8):593–600.
- Endo A, Okada S . Reclassification of the genus Leuconostoc and proposals of Fructobacillus fructosus gen. nov., comb. nov., Fructobacillus durionis comb. nov., Fructobacillus ficulneus comb. nov. and Fructobacillus pseudoficulneus comb. nov. Int J Syst Evol Microbiol. 2008;58(Pt 9):2195–2205.
- Endo A, Futagawa-Endo Y, Sakamoto M, et al. Lactobacillus florum sp. nov., a fructophilic species isolated from flowers. Int J Syst Evol Microbiol. 2010;60(Pt 10):2478–2482.
- Neveling DP, Endo A, Dick LMT. Fructophilic Lactobacillus kunkeei and Lactobacillus brevis isolated from fresh flowers, bees and bee-hives. Curr Microbiol. 2012;65(5):507–515.
- Endo A, Irisawa T, Futagawa-Endo Y, et al. Characterization and emended description of Lactobacillus kunkeei as a fructophilic lactic acid bacterium. Int J Syst Evol Microbiol. 2012;62(Pt 3):500–504.
- Monsan P, Bozonnet S, Albenne C, et al. Homopolysaccharides from lactic acid bacteria. Int Dairy J. 2001;11(9):675–685.
- Naessens M, Cerdobbel A, Soetaert W, et al. Leuconostoc dextransucrase and dextran: production, properties and applications. J Chem Technol Biotechnol. 2005;80(8):845–860.
- Zannini E, Waters DM, Coffey A, et al. Production, properties, and industrial food application of lactic acid bacteria-derived exopolysaccharides. Appl Microbiol Biotechnol. 2016;100(3):1121–1135.
- Zeidan AA, Poulsen VK, Janzen T, et al. Polysaccharide production by lactic acid bacteria: from genes to industrial applications. FEMS Microbiol Rev. 2017;41(Supp_1):S168–S200.
- Zhou Y, Cui Y, Qu X. Exopolysaccharides of lactic acid bacteria: structure, bioactivity and associations: a review. Carbohydr Polym. 2019; 207:317–332.
- Korakli M, Vogel RF . Structure/function relationship of homopolysaccharide producing glycansucrases and therapeutic potential of their synthesised glycans. Appl Microbiol Biotechnol. 2006;71(6):790–803.
- van Hijum SAFT, Kralj S, Ozimek LK, et al. Structure-function relationships of glucansucrase and fructansucrase enzymes from lactic acid bacteria. Microbiol Mol Biol Rev. 2006;70(1):157–176.
- Gangoiti J, Pijning T, Dijkhuizen L. Biotechnological potential of novel glycoside hydrolase family 70 enzymes synthesizing α-glucans from starch and sucrose. Biotechnol Adv. 2018;36(1):196–207.
- Monsan P, Remaud-Siméon M, André I. Transglucosidases as efficient tools for oligosaccharide and glucoconjugate synthesis. Curr Opin Microbiol. 2010;13(3):293–300.
- Andre I, Potocki-Veronese G, Morel S. Sucrose-utilizing transglucosidases for biocatalysis. Top Curr Chem. 2010; 294:25–48.
- Patel S, Goyal A. Functional oligosaccharides: production, properties and applications. World J Microbiol Biotechnol. 2011;27(5):1119–1128.
- Monsan PF, Ouarne F. Oligosaccharides derived from sucrose. In: Charalampopoulos D, Rastall RA, editors. Prebiotics and Probiotics Science. New York: Springer Science + Media, 2009. p. 293–337.
- Monchois V, Remaud-Simeon M, Russell RR, et al. Characterization of Leuconostoc mesenteroides NRRL B-512F dextransucrase (DSRS) and identification of amino-acid residues playing a key role in enzyme activity. Appl Microbiol Biotechnol. 1997;48(4):465–472.
- Fabre E, Bozonnet S, Arcache A, et al. Role of the two catalytic domains of DSR-E dextransucrase and their involvement in the formation of highly alpha-1,2 branched dextran. J Bacteriol. 2005;187(1):296–303.
- Cote GL, Sheng S, Dunlap CA. Alternansucrase acceptor products. Biocatal Biotransfor. 2008;26(1-2):161–168.
- Kralj S, van Geel-Schutten GH, van der Maarel MJEC, et al. Biochemical and molecular characterization of Lactobacillus reuteri 121 reuteransucrase. Microbiology (Reading)). 2004;150(Pt 7):2099–2112.
- Vasileva T, Bivolarski V, Michailova G, et al. Glucansucrases produced by fructophilic lactic acid bacteria Lactobacillus kunkeei H3 and H25 isolated from honeybees. J Basic Microbiol. 2017;57(1):68–77.
- Rabadjiev YK. Study of the genus Lactobacillus and the genus Fructobacillus in the microbiota of honey bees PhD dissertation, Sofia (Bulgaria): Sofia University “St. Kliment Ohridski”, 2018.
- Dols M, Remaud-Simeon M, Monsan PF. Dextransucrase production by Leuconostoc mesenteroides NRRL B-1299. Comparioson with L. mesenteroides NRRL B-512F. Enzyme Microb Technol. 1997;20(7):523–530.
- Goyal A, Katiyar S. Fractionation of Leuconostoc mesenteroides NRRL B-512F dextransucrase by polyethylene glycol: a simple and effective method purification. J Microbiol Methods. 1994;20(3):225–231.
- Miller GL. Use of dinitrosalicylic acid reagent for determination of reducing sugar. Anal Chem. 1959;31(3):426–429.
- Bradford M. A rapid and sensitive method for the quantitation of microgram quantities of protein utilizing the principle of protein-dye binding. Anal Biochem. 1976; 72:248–254.
- Laemmli UK. Cleavage of structural proteins during the assembly of the head of bacteriophage T4. Nature. 1970;227(5259):680–685.
- Miller AW, Robyt J. Detection of dextransucrase and levansucrase on polyacrylamide gels by the periodic acid-Schiff stain: staining artifacts and their prevention. Anal Biochem. 1986;156(2):357–363.
- Bivolarski V, Vasileva T, Gabriel V, et al. Synthesis of glucooligosaccharides with prebiotic potential by glucansucrase URE 13-300 acceptor reactions with maltose, raffinose and lactose. Eng Life Sci. 2018;18(12):904–913.
- Vasileva T, Iliev I, Amari M, et al. Characterization of glycosyltransferase activity of wild-type Leuconostoc mesenteroides strains from Bulgarian fermented vegetables. Appl Biochem Biotechnol. 2012;168(3):718–730.
- Remaud M, Paul F, Monsan P, et al. Characterization of α-(1,3) branched oligosaccharides synthesized by acceptor reaction with the extracellular glucosyltransferase from L. mesenteroides NRRL B-742. J Carbohydr Chem. 1992;11(3):359–378.
- Olvera C, Centeno-Leija S, López-Munguía A. Structural and functional features of fructansucrases present in Leuconostoc mesenteroides ATCC 8293. Antonie Van Leeuwenhoek. 2007;92(1):11–20.
- Bounaix M-S, Gabriel V, Morel S, et al. Biodiversity of exopolysaccharides produced from sucrose by sourdough lactic acid bacteria. J Agric Food Chem. 2009;57(22):10889–10897.
- Ananieva M, Tzenova M, Iliev I, et al. Gene expression of enzymes involved in utilization of xylooligosaccharides by Lactobacillus strains. Biotechnol Biotechnol Equip. 2014; 28:941–948.
- Iliev I, Vasileva T, Bivolarski V, et al. Metabolic profiling of xylooligosaccharides by lactobacilli. MDPI Polymers. 2020;12(10):2387.
- Vasquez A, Forsgren E, Fries I, et al. Symbionts as major modulators of insect health: lactic acid bacteria and honeybees. PLoS One. 2012;7(3):e33188.
- Asenjo F, Olmos A, Henriquez-Piskulich P, et al. Genome sequencing and analysis of the first complete genome of Lactobacillus kunkeei strain MP2, an Apis mellifera gut isolate. Peer J. 2016; 4:e1950.
- Passerini D, Vuillemin M, Ufarte L, et al. Inventory of the GH70 enzymes encoded by Leuconostoc citreum NRRL B-1299 - identification of three novel α-transglucosylases. Febs J. 2015;282(11):2115–2130.
- Bozonnet S, Dols-Laffargue M, Fabre E, et al. Molecular characterization of DSR-E, an alpha-1,2 linkage-synthesizing dextransucrase with two catalytic domains. J Bacteriol. 2002;184(20):5753–5761.
- Claverie M, Cioci G, Vuillemin M, et al. Investigation of the determinants responsible for low molar mass dextran formation by DSR-M dextransucrase. ACS Catal. 2017;7(10):7106–7119.
- Remaud-Simeon M, Willemot R-M, Sarcabal P, et al. Glucansucrases: molecular engineering and oligosaccharide synthesis. J Mol Catal B Enzym. 2000;10(1-3):117–128.
- Manning TS, Gibson GR. Prebiotics. Best Pract Res Cl Ga. 2004;18(2):287–298.
- Koepsell HJ, Tsuchiya HM, Hellman NN, et al. Enzymatic synthesis of dextran; acceptor specificity and chain initiation. J Biol Chem. 1953;200(2):793–801.
- Robyt JF, Eklund SH. Relative quantitative effects of acceptors in the reaction of Leuconostoc mesenteroides B-512F dextransucrase. Carbohydr Res. 1983; 121:279–286.
- Cote GL, Holt SM, Miller-Formore C. Prebiotic oligosaccharides via alternansucrase acceptor reactions. In: Eggleston G, Cote GL, editors. Oligosaccharides in Food and Agriculture, ACS Symposium Series. Washington, DC: Oxford University Press, 2003. Vol 849, p. 76–89.
- Cote GL, Robyt JF. Acceptor reactions of alternansucrase from Leuconostoc mesenteroides NRRL B-1355. Carbohydr Res. 1982; 111:127–142.
- Leathers TD, Ahlgren JA, Cote GL. Alternansucrase mutants of L. mesenteroides strain NRRL B-21138. J Ind Microbiol Biot. 1997;18(4):278–283.
- van den Broek LAM, Hinz SWA, Beldman G, et al. Bifidobacterium carbohydrases-their role in breakdown and synthesis of (potential) prebiotics. Mol Nutr Food Res. 2008;52(1):146–163.
- Ganzle MG, Follador R. Metabolism of oligosaccharides and starch in lactobacilli: a review. Front Microbiol. 2012; 3:1–15.
- de Vos WM, Vaughan EE. Genetics of lactose utilization in lactic acid bacteria. FEMS Microbiol Rev. 1994;15(2-3):217–237.
- Barrangou R, Altermann E, Hutkins R, et al. Functional and comparative genomic analyses of an operon involved in fructooligosaccharide utilization by Lactobacillus acidophilus. Proc Natl Acad Sci U S A. 2003;100(15):8957–8962.
- Makarova K, Slesarev A, Wolf Y, et al. Comparative genomics of the lactic acid bacteria. Proc Natl Acad Sci U S A. 2006;103(42):15611–15616.
- Siezen RJ, van Hylckama Vlieg JE. Genomic diversity and versatility of Lactobacillus plantarum, a natural metabolic engineer. Microb Cell Fact. 2011; 10(Suppl. 1):S3.
- Neubauer H, Glaasker E, Hammes WP, et al. Mechanism of maltose uptake and glucose excretion in Lactobacillus sanfrancisco. J Bacteriol. 1994;176(10):3007–3012.
- Moller MS, Fredslund F, Nakai H, et al. Enzymology and structure of the GH13_31 glucan 1,6-α-glucosidase that confers isomaltooligosaccharide utilization in the probiotic Lactobacillus acidophilus NCFM. J Bacteriol. 2012;194(16):4249–4259.
- Sanz ML, Cote GL, Gibson GR, et al. Prebiotic properties of alternansucrase maltose-acceptor oligosaccharides. J Agric Food Chem. 2005;53(15):5911–5916.
- Sanz ML, Cote GL, Gibson GR, et al. Influence of glycosidic linkages and molecular weight on the fermentation of maltose-based oligosaccharides by human gut bacteria. J Agric Food Chem. 2006;54(26):9779–9784.