Abstract
In our previous study, we firstly cloned the gene of a novel ReNHase (NHase from Rhodococcus erythropolis CCM2595), and the strain was shown to degrade only phenol, hydroxybenzoate, p-chlorophenol, aniline and other aromatic compounds. Here in, we further purified the ReNHase from R. erythropolis CCM2595 and detected its properties of biodegradation. We constructed a plasmid with the gene of ReNHase with His-tag. The encoding ReNHase was cloned and overexpressed in recombinant Escherihia coli and confirmed by sodium dodecyl sulphate polyacrylamide gel electrophoresis (SDS-PAGE). The substrate scope of the new heterologous ReNHase was tested and the efficiency of degradation of nitriles by the ReNHase was also investigated by high efficiency liquid chromatography. We also studied the effect of temperature and pH on the catalysis of adiponitrile by the purified ReNHase. The recombinant E.coli showed high catalytic regioselectivity with high substrate affinity towards dinitriles (especially for adiponitrile) whereas lower affinity towards mononitriles. Compared to whole-cell catalysis, the catalytic time was shortened significantly with the purified ReNHase. The enzyme activity of crude recombinant E.coli was 635 U g−1 (DCW), while the specific activity of purified ReNHase was 63.107 U mg−1. The apparent Km value for the purified ReNHase is 6.6252 mmol L−1, which revealed the good affinity between the purified ReNHase and adiponitrile. The reaction Kcat is 82.77 s−1 and Kcat/Km is 1.249 × 104 (mol−1 L s−1), which showed high catalytic activity towards adiponitrile. We propose that this purified ReNHase may be applied for the industry and sewage treatment for environmental protection.
Introduction
Nitrile hydratase (NHase; EC 4.2.1.84) is a metalloenzyme. NHases are classified into Co-type and Fe-type NHases according to the metal ions associated with the enzyme [Citation1]. NHase catalyses the hydration of nitrile material to the corresponding amides, which are accessible from their respective nitrile precursors [Citation2]. Nitrile precursors are widely used, especially in the manufacture of drugs, synthetic fibers and plastics, and often exhibit low-solubility in aqueous solutions [Citation3,Citation4]. Due to their high toxicity, nitrile residues in the environment can cause serious pollution problems. When nitrile materials enter the natural environment, they pose serious threats to the survival of animals and plants, human life and ecological environment.
Some wild strains containing NHase have been widely used in the industrial production of acrylamide [Citation5,Citation6], nicotinamide and other amides, which have high industrial value [Citation7–10]. In the traditional chemical industry, the synthetic production of amides is always performed with harsh reaction conditions and produces a large amount of by-products and pollutants. On the other hand, the enzyme catalysis method is performed under mild conditions, with good specificity and selectivity. This process is always economically viable and create fewer environmental problems [Citation11,Citation12].
At present, some amides are produced by NHase using wild-type bacterial cells, but the method of obtaining enzymes from wild-type strains is rather accidental and the substrate tolerance of enzymes is poor [Citation13]. Therefore, highly efficient expression of NHase was sought by gene cloning. This fungus has many advantages where other wild fungi cannot compete. Its substrate tolerance, short fermentation period, ease of realising large-scale culture and industrial production, make it to have a promising and broad application prospect [Citation14,Citation15].
In our previous study [Citation16], ReNHase of Rhodococcus. erythropolis CCM2595 manifested high biocatalytic activity. It showed high regioselectivity and substrate specificity for dinitriles, but not for mononitriles. Herein, we report the activity of further purified NHase from R. erythropolis CCM2595 and the investigation of its enzyme properties. Compared to whole-cell catalysis, the catalysis time of the substrate spectrum by purified NHase was shortened significantly. The enzyme activity of purified NHase was much higher than that from crude recombinant E.coli. The Km and Kcat values for the purified NHase were also determined to evaluate the catalytic activity towards adiponitrile, which was a very important substrate to yield 5-Cyanovaleramide during the bioconversion methods [Citation17].
Materials and methods
Chemicals
All the experimental reagents used in this experiment were of analytical grade or above. Nitrile compounds (adiponitrile, malononitrile, terephthalonitrile, 3-cyanopyridine, benzonitrile), amide compounds (4-cyanobenzamide, terephthalamide, nicotinamide, benzamide, adipamide) were purchased from Shanghai Macklin Biochemical Co., Ltd. 5-Cyanovaleramide was bought from Toronto Research Chemicals Inc., Canada.
Plasmid construction
The strain R. erythropolis CCM2595 was the source of nitrile hydration enzyme gene. The gene encoding GenBank accession No JQ023030.2 was amplified by polymerase chain reaction (PCR) with Seamless Cloning Kit (Beyotime Institute of Biotechnology, Beijing, China). The PCR primer sequences were as follows: fo rward: AATT TTGT TTAA CTTTA AGA AGGA GATA TACA TATG TCAG TAA CGAT CGA CC ACA CAA CGG AG, reverse: GGA TCT CAG TGG TGG TGG TGG TGG TGC TCG AGT GCG GCC GCA AGC TTT CAA ACG GTC TGG TCG GT. ReNHase with His-tag was expressed by plasmid pET24a (+). The nitrile hydratase gene was cloned to the NdeI (CATATG) and HindIII (AAGCTT) loci of pET24a (+). The inserted genes were sequenced using Chromas and DNASTAR systems. The constructed plasmid was transformed into E. coli Arctic Express (DE3) strain as the host cell.
Culture condition
Recombinant E. coli cells were grown at LB culture medium (peptone 10 g L−1, yeast extract 5 g L−1, NaCl 10 g L−1), with a kanamycin (50 μg mL−1) and culture at 37 °C, shaken with 220 rpm for 8 h. When the optical density at 600 nm (OD 600) of the culture reached 0.8, the isopropyl methyl isopropyl β-D-1-sulfhydryl galactoside (IPTG) was added at the final concentration of 0.1 mmol L−1. The ReNHase expression was induced at 16 °C at 18 h. Cells were cultured and collected by centrifugation at 4 °C, 9000 g for 10 min, washed twice with phosphate buffer (50 mmol L−1, pH 7.0) and resuspended with binding buffer at pH = 7.5 (50 mmol L−1 phosphate buffer, 300 mmol L−1 NaCl, 20 mmol L−1 imidazole, 22 mmol L−1 n-butyic acid, 10 mmol L−1 Hepes) which were later kept at 4 °C for further purification.
Purification and identification of the recombinant proteins
All purification steps were performed at 0-4 °C. The harvested frozen cells were suspended in binding buffer and lysed by sonication on ice. After centrifugation at 16,200 g for 20 min, the resulting supernatant was filtered through a 0.22-μm pore-size filter. The enzyme was purified by AKTA Pure system (GE AKTA Pure M). Firstly, the column was equilibrated with binding buffer at a flow rate of 0.5 mL min−1. After injecting supernatant onto the column, proteins were eluted with elution buffer with pH7.5 (50 mmol L−1 phosphate buffer, 500 mmol L−1 NaCl, 500 mmol L−1 imidazole, 22 mmol L−1 n-butyric acid, 10 mmol L−1 Hepes) and the active fractions were collected. Overnight dialysis with 0.1 mol L−1 phosphate buffer was performed. The purified proteins were analysed by sodium dodecyl sulphate–polyacrylamide gel electrophoresis (SDS-PAGE) and the protein concentration was quantified by the Bradford method.
Enzyme activity assays
The purified NHase activity was assayed in a reaction mixture (500 µL) containing 20 mmol L−1 substrate, 50 mmol L−1 phosphate buffer (pH 7.0) and purified NHase protein (approximately 0.5 mg mL−1). The reaction was performed at 35 °C for 10 min and terminated by the addition of 500 µL of methanol. The amount of product formed was determined by monitoring the absorbance with high-pressure liquid chromatography and extrapolation from a standard curve. The purified NHase activity was defined as the amount of enzyme used to catalyse the production of 1 µmol 5-cyanopentamide per minute.
Effects of temperature and pH on enzyme activity
We used the adiponitrile to assay the optimum temperature and pH of the purified NHase catalytic activity. We determined the optimal temperature of the purified NHase in a reaction mixture (500 µL), which contains10 mmol L−1 substrate, 50 mmol L−1 phosphate buffer (pH 7.0) and purified NHase protein (about 0.5 mg mL−1). The optimum temperature was determined by testing at temperature between 20 °C and 50 °C. The optimal pH was determined by performing tests at the optimal temperature. Aliquots of phosphate-buffered saline at different pH values between pH 5.8 and 8 which contained 10 mmol L−1 adiponitrile were used.
Kinetics of adiponitrile reaction catalysed by the purified NHase
Three hundred micrilitres of phosphate buffer (pH 7.0, 50 mmol L−1) was placed into the 1.5 mL EP tube. The final concentration of adiponitrile was tuned to 2-20 mmol L−1. After mixing, 0.5 mg mL−1 purified enzyme solution was added to initiate the nitrile hydration reaction. The reaction was conducted at 35 °C for 0-10 min. 500 μL methanol was added to the EP tube to stop the reaction, and centrifugation was performed at 16,200 g for 10 min. The supernatant was taken to detect the concentration of 5-cyanopentamide formed, and the initial reaction speed was calculated at V0.
Substrate spectrum
A reaction mixture (500 µl) containing 20 mM substrate (adiponitrile, malononitrile, 3-cyanopyridine, benzonitrile and terephthalonitrile), 50 mmol L−1 phosphate buffer (pH 7.4) and purified protein (approximately 0.5 mg mL−1). The reaction was performed at 35 °C for 10 min and termination reaction. The amount of product formed was determined by monitoring the absorbance with High performance liquid chromatography (HPLC) and extrapolation from a standard curve.
Analytical methods
HPLC quantified the product by using the ultimate LP-C18 column (4.6 × 250 mm, 5 μm) and an ultraviolet detector. For adiponitrile and malononitrile, the following solvent systems are 25 mmol L−1 H3PO4 buffer (pH 2.5) and methanol (89/11, v/v) at a concentration of 1 mL min−1. The monitoring wavelength was 200/210 nm. The solvent systems used for 3-cyanopyridine, benzonitrile and terephthalonitrile were water and acetonitrile (67/33, v/v), (55/45, v/v), and (75/25, v/v), respectively, was prepared at a concentration of 0.8 mL min−1.
Data analysis
Quantitative data are presented as mean values with standard error of the means (±SEM). Statistical analysis was done using Student’s t-test. Differences were considered statistically significant at p < 0.05 level.
Results
Expression and purification of the ReNHase with his-tag in recombinant E. coli
For the heterologous expression of ReNHase in E. coli, the gene of ReNHase -with His-tag (NHase from R. erythropolis CCM2595) were inserted into pET-24a(+) resulting in the recombinant plasmid (). E. coli transformant harbouring recombination gene was used for expression of ReNHase with His-tag. Sodium dodecyl sulphate-polyacrylamide gel electrophoresis (SDS-PAGE) analyses showed that the target proteins were successfully expressed in E. coli (). The target ReNHase with His-tag protein was purified by AKTA protein purification system. After four times of sampling (sample volume 10 mL each), elution buffer was used to elute the samples, and the active ReNHase with His-tag were collected. Dialysis was also performed overnight with 0.1 mol L−1 phosphate buffer. The purified protein was analysed using SDS-PAGE () and the protein concentration was 0.5 mg mL−1, which was quantified using the Bradford method.
Figure 2. SDS-PAGE analyses for the expression and purification of the ReNHase with His-tag. (a) Expression of the ReNHase with His-tag from recombinant E. coli. Lane 1: 16 °C induced crushed supernatant (Arctic Expression (DE3)); Lane 2: 16 °C induced crushed fragmentation (Arctic Expression (DE3)); Lane 3: 37 °C induced crushed supernatant (BL21 (DE3)); Lane 4: 37 °C induced crushed fragmentation (BL21 (DE3)); Lane 5: Negative control of supernatant; Lane 6: Negative control of precipitation. Lane M: Protein marker (Beyotime Institute of Biotechnology, Beijing, China). (b) Purification products of ReNHase with His-tag (The red arrow indicates the target protein). Lane 1:ReNHase with His-tag before purification; Lane 2:ReNHase with His-tag after purification (twofold dilution). Lane M: Protein marker.
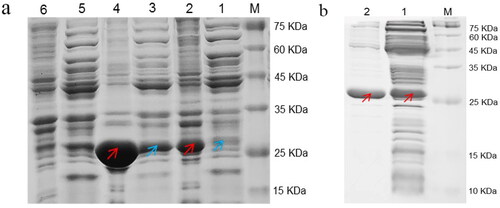
The conversion of nitriles by purified ReNHase
The regioselectivity of different Rhodococcus strains may vary, which can be adapted to different industrial needs [Citation18]. Here in, the biocatalytic properties of different nitriles spectrum by purified ReNHase were investigated (). The substrate affinity of the dinitriles catalysed by purified ReNHase was much higher (especially for adiponitrile) than mononitrile. Significantly, the purified ReNHase exhibited higher activity during the conversion reaction of different nitriles by shortened catalytic time and increased yield of monocyanate products. Compared to whole-cell catalysis, as shown in , the catalytic time of the substrate spectrum was shortened significantly: 20 mmol L−1 adiponitrile, malononitrile and 2 mmol L−1 terephthalonitrile (after ultrasound, the mixture was homogeneous) were completely transformed within only 2 min, 1 h and 10 min, respectively. As for mononitriles, it is noteworthy that the conversion of mononitriles increased and the catalytic time decreased when compared with whole-cell catalysis ().
Figure 3. Comparison of nitriles catalysis by whole cells and nitrile hydratase. (a) Comparison of reaction time; (b) Comparison of conversion of nitriles; (c) Comparison of regioselectivity of nitriles. The error bars represent ± SEM.
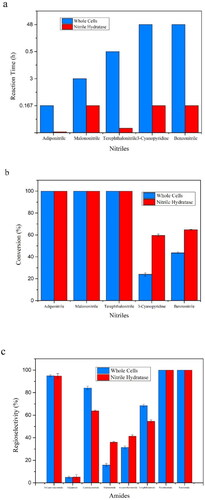
Table 1. Different nitriles catalysed by the purified ReNHase.
Determination of regioselectivity of adiponitrile
NHase was demonstrated to be a potential alternative tool for 5-cyanovaleramide synthesis. In our work, the conversion of adiponitrile and the production of 5-cyanovaleramide and adipamide can be observed when treated with the purified ReNHase. As shown in , 20 mmol L−1 adiponitrile was completely converted to 5-cyanovaleramide and adipamide within 2 min, in which the reaction time was much shorter when compared with whole-cell catalysis. The main product was still 5-cyanovaleramide, though only a small amount of adipamide was produced (). We also detected that the enzyme specific activity of the purified ReNHase was 63.107 U mg−1, while the whole-cell catalysed reaction of adiponitrile was 635 U g−1 (DCW). The enzyme specific activity of the purified ReNHase was achieved by a 98.4-fold increase when compared with the whole-cell catalysed reaction.
Figure 4. Production of 5-cyanovaleramide and adipamide from adiponitrile. (a)Time course of production of 5-cyanovaleramide and adipamide from adiponitrile by the purified ReNHase. Symbols: adiponitrile (■), 5- cyanovaleramide (●), adipamide (▲). The error bars represent mean ± SEM. (b) HPLC analysis of catalytic reaction at the 2-minute reaction.
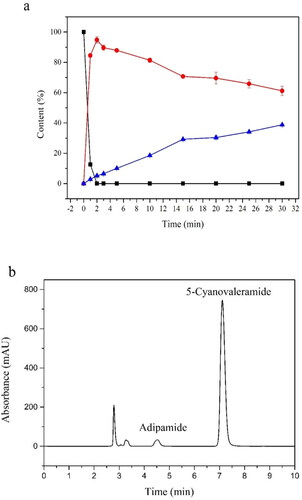
Kinetics of catalysis of adiponitrile by the purified ReNHase
The kinetic parameters of the reaction by the purified ReNHase followed the Michaelis–Menten model (). The calculated Km is 6.6252 mmol L−1. Kcat is the turnover number, which is the number of substrate molecule each enzyme site converts to product per unit time. Herein, we found that the reaction Kcat is 82.77 s−1, which indicates that 82.77 substrate adiponitriles were transformed into 5-cyanovaleramide per unit time by a single ReNHase. Another way to measure the catalytic efficiency of the ReNHase is to determine the Kcat/Km ratio, which allows a way to test how effective the ReNHase is on substrate adiponitriles. In your study we found the Kcat/Km is 1.249 × 104 (mol−1 L s−1).
Effects of temperature and pH on the activity of purified ReNHase towards adiponitrile
The effect of temperature on the catalysis of adiponitrile to 5-cyanovaleramide by the purified ReNHase is shown in . The detected temperature range is from 20 °C to 50 °C. When the temperature was increased from 20 °C to 35 °C, the reactivity was enhanced significantly. As the temperature continued to increase, the reaction activity decreased. The results showed that the purified ReNHase lost part of its activity at a high temperature. Therefore, the optimal temperature for the reaction by the purified ReNHase was 35 °C. We also investigated the effect of pH on the catalysis of adiponitrile by the purified ReNHase. As shown in , when the pH value increased from 5.8 to 7.4, the reactivity was enhanced. However, as the pH value continued to increase, the reactivity was decreased significantly. The results showed that the optimum pH value of the reaction was 7.4. Altogether, in our work, the optimum temperature and pH of the ReNHase were 35 °C and 7.4, respectively.
Discussion
The nitrile hydratase (ReNHase) derived from R. erythropolis CCM2595 that exhibited potential in the bioconversion of nitriles especially in dinitrile, was shown to degrade only phenol, hydroxybenzoate, p-chlorophenol, aniline and other aromatic compounds [Citation19]. Escherichia coli as a host organism is widely used in recombinant DNA technology because of its well-studied genome and high efficiency culture on a large scale. Although E.coli enables the production of valuable foreign proteins at high expression rates and high concentrations, the expression of proteins in E. coli often leads to the formation of inclusion bodies (IBs) which contain a high proportion of the recombinant proteins in incorrect steric structures without the desired biological activities [Citation20]. In our work, SDS-PAGE analyses showed that the target NHases were successfully expressed in E. coli (), but there was a large part of IBs that appeared in the form of fragments in the E. coli Arctic Expression (DE3) and BL21 (DE3) (). In our future work, we will construct the recombinant ReNHase with fusion tags (such as NT tag, MBP tag, PGB1 tag and SUMO tag) to eliminate the proportion of IBs. These fusion tags have contributed to increased protein solubility and expression level, which have been proved to promote recombinant protein expressions [Citation21].
Since the discovery of the first NHase from Arthrobacter sp. J1 in 1980, many NHase-producing strains belonging to the genera Pseudomonas, Serratia, Rhodococcus, Corynebacterium, Nocardia, Pseudonocardia, etc. have been isolated from the natural environment [Citation22]. Rhodococcus has been the main source of new NHases up to now. However, the regioselectivity of different Rhodococcus strains may vary, which can be adapted to different industrial needs [Citation18]. In our results, we found that the substrate affinity of the dinitriles catalysed by purified ReNHase was much higher (especially for adiponitrile) than mononitrile (). Significantly, the purified ReNHase exhibited higher activity during the conversion reaction of different nitriles by shortened catalytic time and increased yield of monocyanate products. In our previous study, during the whole-cell catalysis, 20 mmol L−1 adiponitrile, malononitrile, 2 mmol L−1 terephthalonitrile(after ultrasound, the mixture was homogeneous) could be completely transformed within 10 min, 3 h and 30 min, respectively [Citation16].
5-Cyanovaleramide is an important intermediate of azafenidin, caprolactam and nylon 6 production. In addition to the high reaction temperature and pressure during the chemical synthesis of 5-cyanovaleramide from adiponitrile, it produced the byproduct adipamidethe because of the poor regioselectivity of this reaction, which led to the reduced yield and purity of 5-cyanovaleramide [Citation23]. In our work, the main product was still 5-cyanovaleramide, though only a small amount of adipamide was produced () when treated with the purified ReNHase only within 2 min. In our previous study, the adiponitrile could completely be consumed within 10 min when it was treated with whole-cell catalysis [Citation16]. The amount of 5- cyanovaleramide decreased and the amount of adipamide increased in a time-dependent manner. The results showed that the purified ReNHase maintained high regioselectivity of the biocatalysis of adiponitrile when compared with whole-cell catalysis. Apart from that, the conversion time was shortened markedly when treated with purified ReNHase. At present, the number of microorganisms which can produce 5-cyanovaleramide from adiponitrile is still limited. Chen et al. [Citation24] reported that the NHase from Pseudomonas strain SY031 can completely hydrolyse 10 mmol L−1 adiponitrile within 500 min at 35 °C with small amounts of adipamide generated. Shen et al. [Citation25] screened the strain R. ruber CGMCC3090 with moderate conversion capability and regioselectivity to adiponitrile. In their work, they optimised the reaction parameters for the biosynthesis of 5-cyanovaleramide and found that 100% of 1.5 mol L−1 adiponitrile (162 g L−1) can be converted into 5-cyanovaleramide through 100 min at 30 °C [Citation25]. In our work, 20 mmol L−1 adiponitrile was completely converted within only 2 min with 95% selectivity to 5-cyanovaleramide and 5% selectivity to adipamide. The above data suggested the purified ReNHase showed further improvement to the high catalytic efficiency for potential industrial applications.
The Michaelis-Menten constant (Km) can reflect the affinity between enzyme and substrate, that is, the smaller the Km value is, the greater the affinity between enzyme and substrate is [Citation26]. Kcat is the turnover number, which is the number of substrate molecule each enzyme site converts to product per unit time. Another way to measure the catalytic efficiency of the ReNHase is to determine the Kcat/Km ratio, which allows to test how effective the ReNHase is on substrate adiponitriles. The greater the ratio is, the higher the rate of catalysis is; conversely, the lower the ratio is, the slower the catalysis is [Citation17]. In our work, we showed the Km is 6.6252 mmol L−1, which revealed the good affinity between the purified ReNHase and adiponitrile. Kcat is 82.77 s−1, which means that 82.77 substrate adiponitriles were transformed into 5-cyanovaleramide per unit time by a single ReNHase (). The Kcat/Km is 1.249 × 104 (mol−1 L s−1), which showed high catalytic activity towards adiponitrile when compared to other reports [Citation27,Citation28].
The production of 5-cyanovaleramide from adiponitrile by chemical hydrolysis requires harsh conditions like high temperature and pressure, because the nitrile bond in nitrite compounds is very stable [Citation29]. However, bioconversion of adiponitrile to 5-CVAM mediated by regio-selective nitrile hydratase has become an attractive and promising alternative, which required mild temperature environment. As shown in , the optimum temperature and pH of the ReNHase were 35 °C and 7.4.
In summary, the ReNHase showed its high regioselectivity with higher substrate specificity towards dinitriles (especially adiponitrile) whereas lower specificity towards mononitrile. However, the purified enzyme can easily be deactivated and cannot been used repeatedly. Compared with free, immobilised enzyme can not only maintain its high efficiency, but also exhibit mild enzyme-catalysed reaction characteristics [Citation26, Citation30,Citation31]. Therefore, in order to overcome the deficiency of free enzyme for the application in industry practices, the preparation of the immobilisation of the purified ReNHase will be subjected to further study.
Conclusions
We purified ReNHase from the crude extract of recombined E.coli and further investigated the biocatalysis activity of different nitrile substrates by the purified ReNHase. In addition to the maintainability of the high regioselectivity towards dinitriles, we found the purified ReNHase exhibited higher activity during the conversions of various dinitriles by shortening the catalytic time and produced an increased yield of monocyanate product when compared to whole-cell catalysis we have previously reported. Especially for adiponitrile, the purified ReNHase can be completely converted to 5-cyanovaleramide and adipamide with high regioselectivity, in which the reaction time was much shortened when compared with whole-cell catalysis. Our finding provides the potential industrial application of the purified ReNHase with high catalytic regioselectivity in future.
Disclosure statement
No potential conflict of interest was reported by the author(s).
Data availability statement
All data that support the findings reported in this study are available from the corresponding author upon reasonable request.
Additional information
Funding
References
- Yu HJS, Wang M, Liang Y, et al. Biodegradation of nitriles by rhodococcus. In: Alvarez H, editors. Biology of rhodococcus. Cham: Springer; 2019.
- Xia Y, Peplowski L, Cheng Z, et al. Metallochaperone function of the self-subunit swapping chaperone involved in the maturation of subunit-fused cobalt-type nitrile hydratase. Biotechnol Bioeng. 2019;116(3):481–489.
- Cheng Z, Xia Y, Zhou Z. Recent advances and promises in nitrile hydratase: From mechanism to industrial applications. Front Bioeng Biotechnol. 2020;8:352.
- He YC, Xu JH, Su JH, et al. Bioproduction of glycolic acid from glycolonitrile with a new bacterial isolate of Alcaligenes sp. ECU0401. Appl Biochem Biotechnol. 2010;160(5):1428–1440.
- Jiao S, Li F, Yu H, et al. Advances in acrylamide bioproduction catalyzed with Rhodococcus cells harboring nitrile hydratase. Appl Microbiol Biotechnol. 2020;104(3):1001–1012.,
- Lankathilaka KPW, Stein N, Holz RC, et al. Cellular maturation of an iron-type nitrile hydratase interrogated using EPR spectroscopy. J Biol Inorg Chem. 2019;24(7):1105–1113.
- Xia Y, Cui W, Cheng Z, et al. Improving the thermostability and catalytic efficiency of the subunit-fused nitrile hydratase by semi-rational engineering. ChemCatChem. 2018;10(6):1370–1375.,
- Kamal A, Kumar MS, Kumar CG, et al. Bioconversion of acrylonitrile to acrylic acid by rhodococcus ruber strain AKSH-84. J Microbiol Biotechnol. 2011;21(1):37–42.
- Turk SC, Kloosterman WP, Ninaber DK, et al. Metabolic engineering toward sustainable production of Nylon-6. ACS Synth Biol. 2016;5(1):65–73.
- Bhalla TC, Kumar V, Kumar V, et al. Nitrile metabolizing enzymes in biocatalysis and biotransformation. Appl Biochem Biotechnol. 2018;185:925–946.
- Sheldon RA, van Pelt S. Enzyme immobilisation in biocatalysis: why, what and how. Chem Soc Rev. 2013;42(15):6223–6235.
- Yang Z, Pei X, Xu G, et al. Efficient Production of 2,6-difluorobenzamide by recombinant Escherichia coli expressing the aurantimonas manganoxydans nitrile hydratase. Appl Biochem Biotechnol. 2019;187(2):439–448.
- Sharma B, Dangi AK, Shukla P. Contemporary enzyme based technologies for bioremediation: A review. J Environ Manage. 2018;210:10–22.
- Gileadi O. Recombinant protein expression in E. coli: A historical perspective. Methods Mol Biol. 2017;1586:3–10.
- Mashweu AR, Chhiba-Govindjee VP, Bode ML, et al. Substrate profiling of the cobalt nitrile hydratase from rhodococcus rhodochrous ATCC BAA 870. Molecules. 2020;25(1):238.
- Wang L, Liu S, Du W, et al. High Regioselectivity Production of 5-Cyanovaleramide from adiponitrile by a novel nitrile hydratase derived from rhodococcus erythropolis CCM2595. ACS Omega. 2020;5(29):18397–18402.
- Eisenthal R, Danson MJ, Hough DW. Catalytic efficiency and kcat/KM: a useful comparator?Trends Biotechnol. 2007;25(6):247–249.
- Gong JS, Shi JS, Lu ZM, et al. Nitrile-converting enzymes as a tool to improve biocatalysis in organic synthesis: recent insights and promises. Crit Rev Biotechnol. 2017;37(1):69–81.,
- Rucka L, Volkova O, Pavlik A, et al. Expression control of nitrile hydratase and amidase genes in Rhodococcus erythropolis and substrate specificities of the enzymes. Antonie Van Leeuwenhoek. 2014;105(6):1179–1190.
- Su Z, Lu D, Liu Z. Refolding of inclusion body proteins from E. coli. Methods Biochem Anal. 2011;54:319–338.
- Sarr M, Kronqvist N, Chen G, et al. A spidroin-derived solubility tag enables controlled aggregation of a designed amyloid protein. Febs J. 2018;285(10):1873–1885.
- Prasad S, Bhalla TC. Nitrile hydratases (NHases): at the interface of academia and industry. Biotechnol Adv. 2010;28(6):725–741.
- Hann EC, Eisenberg A, Fager SK, et al. 5-Cyanovaleramide production using immobilized Pseudomonas chlororaphis B23. Bioorg Med Chem. 1999;7(10):2239–2245.
- Chen J, Huang YT, Deng SG, et al. Biotransformation of Adiponitrile to 5-cyanovaleramide by Pseudomonas Sp. SY031 Resting Cells. AMR. 2013;791-793:204–207.
- Shen YB, Wang M, Li XD, et al. Highly efficient synthesis of 5-cyanovaleramide by Rhodococcus ruber CGMCC3090 resting cells. J Chem Technol Biotechnol. 2012;87(10):1396–1400.
- Xu J, Zhang R, Han Z, et al. The highly-stable immobilization of enzymes on a waste mycelium carrier. J Environ Manage. 2020;271:111032.
- Mitra S, Holz RC. Unraveling the catalytic mechanism of nitrile hydratases. J Biol Chem. 2007;282(10):7397–7404.
- Rao S, Holz RC. Analyzing the catalytic mechanism of the Fe-type nitrile hydratase from Comamonas testosteroni Ni1. Biochemistry. 2008;47(46):12057–12064.
- Heidari A, Asoodeh A. A novel nitrile-degrading enzyme (nitrile hydratase) from Ralstonia sp.ZA96 isolated from oil-contaminated soils. Biocatal Agric Biotechnol. 2019;21:101285.
- Gennari A, Fuhr AJ, Volpato G, et al. Magnetic cellulose: Versatile support for enzyme immobilization - A review. Carbohydr Polym. 2020;246:116646.
- Zhang JP, Zhu AX, Lin RB, et al. Pore surface tailored SOD-type metal-organic zeolites. Adv Mater. 2011;23(10):1268–1271.