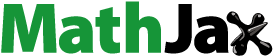
Abstract
Vegetative biomass contains a large number of macromolecular substances such as cellulose and hemicellulose, which can be used by microorganisms to produce biofuels and other chemical byproducts. In this study, a filamentous fungus with high β-glucosidase activity was isolated from mangrove soil in Hainan Province, China. Through morphological identification and internal transcribed spacer (ITS) sequence analysis, the strain was identified as Trichoderma reesei. Fermentation conditions were optimized with the response surface method to improve β-glucosidase activity. Inoculum, pH and liquid volume in flask were found to be the key factors. We further examined the optimal range of the three factors using steepest ascent path, and optimum conditions were further investigated according to a Box-Behnken design. We calculated the optimized fermentation conditions to be: carbon source (microcrystalline cellulose (MCC)) 1%, nitrogen source (yeast extract) 0.5%, pH 3.68, inoculum of 3.22 × 105 cfu/mL, temperature 28 °C, shaking speed of 160 rpm, and liquid volume in flask of 84.74 mL/250 mL. These conditions increased the β-glucosidase activity 6-fold to 1.13 U/mL compared to that before optimization. The resulting β-glucosidase had an optimum pH of 5.0 and an optimum temperature of 45 °C, and showed high thermal stability at 30-60 °C.
Introduction
Vegetative biomass, a heterogeneous complex composed of cellulose and hemicellulose, is the most abundant renewable biological resource on the planet [Citation1,Citation2]. The biodegradation of cellulosic material has been reported to have potential importance in numerous industrial and agricultural applications [Citation3,Citation4]. Cellulase, a catalyst for the degradation of cellulose to glucose, plays an important role in producing available carbon [Citation5]. Cellulase is composed of endo-1,4-β-glucanase (EGL), cellobiohydrolase or exo-1,4-β-glucanase (CBH), and β-glucosidase or cellobiase (BGL) [Citation6,Citation7]. Among these components, β-glucosidase is one of the main enzymes that biodegrades cellulose or hemicellulose into glucose [Citation8]. β-glucosidase catalyzes the hydrolysis of the β-glucoside bond in polysaccharides and releases glucose from the non-reducing end. Recently, β-glucosidase has received renewed research interest due to its role in cellulose decomposition and its application in a variety of other fields. Many studies have reported that several β-glucosidases have high enzyme activity in the hydrolysis of daidzin [Citation9], genistin [Citation10], stevioside [Citation11] and oleuropein [Citation12]. In addition, the extensive consumption of fossil fuels has increased the demand for β-glucosidase, especially for the enzymatic biocatalysis of cellulose for bioenergy [Citation13].
The saccharification of cellulose occurs in extreme environments with high temperatures (about 50-70 °C), low pH values or high concentrations of inhibitors [Citation14]. However, most β-glucosidases in nature have low expression, poor activity, poor pH and temperature tolerance, and cannot be produced in large quantities, which seriously limits the industrial and commercial uses of β-glucosidases. Therefore, the development of heat-resistant and acid-resistant β-glucosidase has been central to promoting its use in the bioconversion of cellulose biomass [Citation15]. Existing research shows that the fungus Trichoderma reesei has the potential to produce extracellular cellulase and is one of the most widely used cellulase-producing microorganisms [Citation16,Citation17]. Several β-glucosidases have been purified and characterized from a variety of fungi, including Aspergillus versicolor [Citation18] and A. oryzae [Citation19]. However, the activity of β-glucosidase produced by T. reesei is low, and the efficiency of biomass degradation is not high, which limits its industrial application [Citation20]. Under cellulase induction conditions, β-glucosidase secreted by T. reesei accounts for only about 1% of total cellulase [Citation21]. Several strategies have been employed in attempts to increase β-glucosidase activity, such as mixed-strain fermentation and homologous or heterologous expression [Citation17,Citation22,Citation23]. However, β-glucosidase activity remains a key bottleneck in the production of cellulase by T. reesei.
β-glucosidase is an inducible enzyme and is strongly influenced by culture conditions and media components. Statistical optimization modules, such as Taguchi and response surface methodology (RSM), can be used to identify the interacting effects of culturing variables and media components that lead to maximum enzyme production [Citation24]. In this paper, RSM was used to optimize the fermentation conditions, thereby improving the activity of β-glucosidase. We used a combination of a Plackett-Burman experimental design, the steepest ascent path and a Box-Behnken design to improve the activity of β-glucosidase, to further isolate, purify and determine the properties of β-glucosidase, and to provide a theoretical basis for future studies of β-glucosidase in T. reesei.
Materials and methods
Medium and chemicals
We used an extracellular-β-glucosidase-producing strain of T. reesei, which was newly isolated from the surface soil of mangroves in Dongzhai Port, Hainan Province, China. The ability of the strain to produce extracellular β-glucosidase was evaluated through qualitative chromogenic tests on esculin-containing media, in which the extracellular β-glucosidase produced a black zone around the colony [Citation25]. The medium used was as follows: CMC 1 g, peptone 0.1 g, FeSO4 · 5H2O 0.001 g, NaCl 0.05 g, MgSO4 0.05 g, 1 g esculin, 0.25 g ammonium iron (III) citrate, 1 g agar powder, with a constant volume of 100 mL. Extracellular β-glucosidase production was then quantitatively evaluated with liquid submerged fermentation. The fermentation medium was as follows: Microcrystalline cellulose (MCC) 1 g, yeast extract 0.3 g, K2HPO4·3H2O 2.62 g, MgSO4·7H2O 0.3 g, FeSO4·7H2O 0.005 g, ZnSO4·7H2O 0.0014 g, MnSO4·H2O 0.0017 g, CoCl2·6H2O 0.00287 g, and fixed volume to 100 mL with McILvaine buffer of pH 5. Both media were autoclave-sterilized for 20 min at 120 °C.p-Nitrophenyl-β-d-glucopyranoside (pNPG) and esculin were purchased from Solarbio Co., Ltd. (Beijing, People’s Republic of China), and yeast extract was purchased from OXOID Co., Ltd. (Besingstoke, UK). E.Z.N.A.® Fungal DNA Mini Kit was purchased from OMEGA Co., Ltd. (, America). The microscope was purchased from OLYMPUS Co., Ltd. (Tokyo, Japan). Phanta® Max Super-Fidelity DNA Polymerase was purchased from Vazyme Co., Ltd. (Nanjing, China).
Strain and culture conditions
We collected 10 g soil samples from mangrove in Hainan, added 90 mL aseptic water under aseptic conditions and shook the samples for 5 min. After letting samples stand for 30 min, the supernatant (∼10 mL) was added to 90 mL of enrichment medium (250 mL flask) and cultured at 180 rpm and 28 °C for 48 h. The enrichment medium was diluted in a gradient (10 mL), coated on primary screening plates, and cultured at 28 °C for 7 d. Colonies were selected and separated by four zones until a single strain was obtained. All isolated strains were inoculated on esculin agar medium and cultured overnight at 28 °C. The strains with large precipitation circles and dark color were selected for liquid fermentation. The selected fungi were inoculated on potato dextrose agar (PDA), and sporulation medium was obtained after 7 days to prepare the spore suspension. Spores were shaken and mixed in sterile water with an inoculation ring. Hyphae were filtered out of suspension using aseptic cotton on a 5 mL syringe, resulting in a single spore suspension. Spores were counted via a hemocytometer and then diluted with sterile saline to standardize the spore count. The spore solution at 103 cfu/mL was inoculated in 100 mL fermentation medium (250 mL flask). Enzyme activity in the supernatant of the fermentation broth was determined after shaking cultures at 28 °C and 160 rpm for 7 d.
Enzyme assay
One unit of enzyme activity (U) was defined as the amount of β-glucosidase required to hydrolyze the substrate to produce 1 μmol of product per minute under certain reaction conditions. Each assay had triplicates. The assay is described below:
The reaction systems containing 100 μL of 4 mmol/L pNPG and 100 μL of appropriately diluted enzyme solution were incubated at 40 °C and pH 5.0 for 10 min, followed by the addition of 600 μL of 1 mol/L Na2CO3 to terminate the reaction. After cooling down to room temperature, the OD400 was measured. The enzymatic activity was determined based on the amount of pNP released.
Molecular identification of strain S12
Total cellular DNA was obtained by E.Z.N.A.® Fungal DNA Mini Kit. Then the forward primer (TCCGTAGGTGAACCTGCGG) and reverse primer (TCCTCCGCTTATTGATATGC) were used to amplify ITS sequences using Phanta® Max Super-Fidelity DNA Polymerase (P505). Amplification conditions were as follows: ddH2O (18 μL), 2 × Phanta Max Buffer (25 μL), dNTP Mix (1, μL;10 mmol/L each), Forward primer (2 μL; 10 μmol/L), Reverse prime (2 μL; 10 μmol/L), Phanta Max Super-Fidelity DNA Polymerase (1 μL) and DNA template (1 μL).
The sequence data were investigated in the GenBank database using the BLAST program available in the National Center for Biotechnology Information (NCBI). The obtained unknown sequence was compared to all of the sequences available in the database to assess DNA similarities (Supplement 1).
Experimental design and analysis
Plackett-Burman experimental design
We selected 11 factors across 2 levels, with X8, X9, X10 and X11 set as null conditions to estimate the test error. The tested factors were carbon source (X1, %), nitrogen source (X2, %), pH (X3), inoculate amount (X4, cfu/mL), temperature (X5, °C), shaking speed (X6, rpm), and liquid volume in flask (X7, mL/250 mL). Each factor was tested at two levels with 12 replicates (). The first-order model was:
where β0 is the intercept model, βi is the regression coefficient, Xi is the fermentation process variable, Y is the enzyme specific activity (U/mL). Each variable was defined at two levels: high (+1) and low (–1). The difference between the average of at least three measurements obtained at the (+1) and (–1) levels of each factor was taken as the effect of that study design [Citation26].
Table 1. Design and results of Plackett-Burman experimental design.
Steepest ascent path analysis
The path of steepest ascent is a procedure for finding an optimum by moving in the direction that maximizes the increase in a given response [Citation27]. According to the level of each factor and effect size of the Plackett-Burman experimental design, we determined the change step of each key factor. According to the regression coefficients, we determined the ascent direction and selected the higher positive coefficient factors, while minimizing negative coefficient factors.
The Box-Behnken design
The Box-Behnken design was used to further investigate the optimal levels of the key factors in specific enzyme activity. Using analysis of variance (ANOVA) to identify significant experimental factors, the regression produces a response surface (). Then we evaluated the ANOVA via a modelling statistical analysis. The second-order polynomial coefficients were calculated and analyzed using the ‘Design Expert’ software package (Version 8.0, Stat-Ease Inc., Minneapolis, USA) with the following regression equation:
where Y is the specific activity of the enzyme, β0 is the offset term, βi is the linear effect, βii is the square effect, βij is the interaction effect, and Xi and Xj are the variables of the fermentation process.
Table 2. Box-Behnken design and results.
Verification experiment
After statistically identifying the optimal fermentation conditions, we carried out three parallel experiments under these conditions. The average of the results of these experiments was used to assess the reliability of the model and finalize the optimization conditions.
Biochemical characterization
pNPG was used as the substrate for biochemical characterization of the fermentation broth supernatant. The optimal temperature was determined at an optimal pH 5.0 for 10 min in the buffers with the temperatures range of 30–90 °C. The thermostability was determined by measuring the residual activity in buffer (50 mmol/L Na2HPO4-citric acid) at various temperatures (30–70 °C) for 60 min. The samples were rapidly cooled in an ice-water bath and residual activity was measured by the above method. The optimal pH was determined at 50 °C for 10 min in the buffers with 2.0–9.0 (50 mmol/L Na2HPO4-citric acid, pH 2.0–8.0; 50 mmol/L glycine-NaOH pH 8.0–9.0). The pH stability was examined by measuring the residual enzyme activity under the standard conditions (50 °C for 10 min) at different pH values (pH 2.0–9.0) for 60 min. The amount of residual activity was determined according to the activity assay method.
The highest specific activity was taken as equivalent to 100% specific activity.
Results
Screening and identification of strains
Preliminary screening results of esculin agar plate
After the first round of enrichment culture and preliminary screening, a total of 26 strains with different colony morphology were obtained by streaking. The screened strains were inoculated on esculin agar plates. Each strain was inoculated three times, with the average of the three trials taken as the result for that strain. Because β-glucosidase can hydrolyze the β-D-glucosidic bond in esculin to produce glucose and esculetin (6,7-dihydroxycoumarin), the free esculetin reacts with the ferric ions present in the medium resulting in a black precipitate. These black precipitate circles were assumed positive colonies (). After 12 h growth at 28 °C, 5 positive colonies were observed. The complete results are shown in . The highest enzyme activity of positive strains in the fermentation cycle was also compared. High activity was detected in the supernatant of 3 strains (). The fermentation activity of T. reesei strain S12 was the highest, reaching 0.191 U/mL after 7 days (). S12 was therefore selected as the target strain for further study.
Table 3. Results of plate precipitation ring determination of esculin.
Table 4. β-glucosidase activity in the supernatant of fermentation broth (7d).
Taxonomic identification of strain S12
Morphological identification: After culturing on PDA medium at 28 °C for 72 h, the colony radius of strain S12 was measured at 52 mm, and a diffusive yellow pigment formed on the back of the plate (). After 40 h, dark green spores were visible in the center of the colony in a disk shape. A large number of hyphae and spores were observed via optical microscope, with the branches approximately perpendicular to the superior branches. We preliminarily identified the fungus as an Ascomycetes using the Manual of Fungal Identification [Citation28].
Figure 2. Morphological characteristics and spore morphology of Trichoderma reesei S12 observed under microscope (OLYMPUS CX21FS1, 400×).
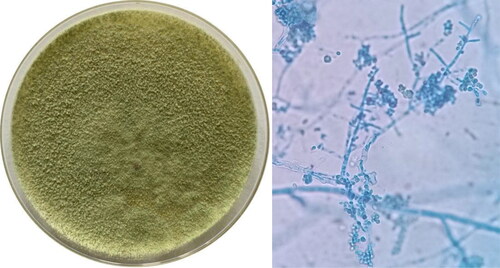
Molecular biological identification: We extracted total DNA from the fungus using E.Z.N.A.® Fungal DNA Mini Kit [Citation29], and successfully amplified the internal transcribed spacer (ITS) region, confirmed via gel electrophoresis (). We compared the resulting ITS sequence against the NCBI blastn database and confirmed that strain S12 belongs to Trichoderma reesei (>99% nucleotide similarity).
Plackett-Burman experimental design
The factors that significantly affected the specific enzyme activity of T. reesei in the fermentation medium were inoculum, pH, and liquid volume in flask ( and ; R2=0.9282). On the other hand, temperature had no significant effect. Thus, to increase enzyme production, we increased the inoculum and pH, and reduced the liquid volume in the flask.
Table 5. The level and effect evaluation of various factors in Plackett-Burman Design.
The path of steepest ascent
The steepest ascent path analysis () showed that by gradually increasing the inoculum, increasing the pH and decreasing the liquid volume in flask, the specific enzyme activity increased at first and then decreased. When the inoculum was 2.75 × 105 cfu/mL, pH was 3.5, and the liquid volume in flask was 85 mL, the specific enzyme activity reached its highest point, which was the maximum response range of the three factors. Therefore, we designed the response surface experiment with these factor levels as the central values.
Table 6. Experimental design and results of steepest ascent path.
Box-Behnken design and response surface methodology
After the optimal values of the three important factors were determined, we performed a response surface analysis with inoculum, pH and liquid volume in flask as the central points ( and ; R2 =0.986; p = 0.0004).
Table 7. Box-Behnken design regression analysis.
When the liquid volume in flask was fixed, the inoculum increased from 5 × 104 to 5 × 105 and the pH increased from 3.0 to 4.0 (). The β-glucosidase activity increased and subsequently decreased, and the vertex of the curved surface represented the maximum value of β-glucosidase activity (). We performed similar analyses to assess the interaction between inoculum and liquid volume in flask (), and pH and liquid volume in flask ().
Figure 4. Response surface (left) and the corresponding contours (right) showing the effects of inoculation amount and pH on the activity of β-glucosidase produced by Trichoderma reesei S12.
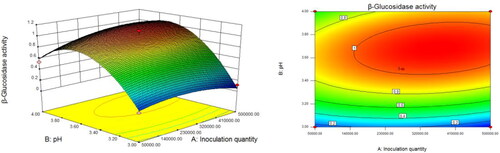
Model verification
According to the model, when the inoculum was 3.22 × 105 cfu/mL, the pH was 3.68 and the liquid volume in flask was 84.74 mL/250 mL, the predicted maximum response value was 1.13 U/mL. This predicted response was 6 times higher than before optimization. In order to validate these predicted results, we conducted shaking flask fermentation experiments three times under these optimal conditions. The results of these experiments were 1.13 U, 1.16 U and 1.12 U, which are similar to the predicted values. After optimization, the full fermentation conditions of T. reesei S12 strain were determined to be carbon source 1%, nitrogen source 0.5%, pH 3.68, inoculum of 3.22 × 105 cfu/mL, culture temperature of 28 °C, shaking speed of 160 rpm, liquid volume of 84.74 mL/250 mL.
Study on enzymatic properties of β-glucosidase from T. reesei
T. reesei β-glucosidase had the most suitable pH, pH stability, optimum temperature, and thermal stability for application in industrial processes ( and ). T. reesei showed very high β-glucosidase activity, and could tolerate a wide range of pH (3.0–5.0). The specific enzyme activity was relatively stable when pH was 2.0–5.0 but decreased sharply when pH reached 7 in the reaction mixture. β-glucosidase activity was also tolerant to high temperatures (up to 60 °C), although the thermal stability decreased sharply above 70 °C.
Discussion
Mangroves, which grow on relatively narrow intertidal zones with slightly acidic soils, are suitable habitat for salt-tolerant microbial species [Citation30]. Plant litter is abundant in the mangrove area of Hainan Island, which led us to suspect that this environment would be home to microorganisms that produce cellulose-degrading enzymes. In this unique environment, there may be many low pH-adapted microorganisms with high cellulase production. Previously, we successfully identified an acidophilic and heat-tolerant fungus from mangrove soil. In order to improve the level of enzyme production from this fungus, in this study we optimized the fermentation conditions via response surface methods with confirmation by single factor experiments.
We optimized and measured β-glucosidase activity using pNPG as a substrate. The optimum enzyme production conditions of T. reesei S12 strain after optimization were determined to be carbon source 1%, nitrogen source 0.5%, pH3.68, inoculum of 3.22 × 105 cfu/mL, temperature of 28 °C, shaking speed of 160 rpm, liquid volume in flask of 84.74 mL/250 mL. . Naturally, in a real-life scenario the values of pH and inoculum volume would be rounded up to pH 3.7 and 85 mL/250 mL. We repeated the experiments under the optimal conditions in triplicate, with the results consistently showing specific enzyme activity that was about 6 times higher than that of the original strainWe found that the three factors significantly affecting β-glucosidase production were pH, inoculation amount and liquid volume in flask. This is consistent with previous results, given that pH is known to strongly affect the production of many enzymes [Citation31]. We also saw that the interaction between pH and inoculum was significant, while the interaction between pH and liquid volume in flask, and between inoculum and liquid volume in flask were not significant.
According to prior research, the production of conidia by Trichoderma requires high levels of dissolved oxygen [Citation32]. An increase in dissolved oxygen can enhance the production of conidia as well as the speed of conidia production, thus affecting enzyme activity, while liquid volume in flask affects the demand for oxygen and the efficiency of transfer and exchange of nutrients. Low oxygen is not conducive to cell growth, produces acidic intermediate metabolites, reduces environmental pH and affects cell growth. On the other hand, too much oxygen will accelerate the cell aging, and then affect the cell metabolism and byproduct accumulation. Therefore, we expect that the appropriate liquid volume in flask relates to dissolved oxygen, with the optimal value promoting microbial growth and sporulation [Citation33]. Mandels and Reese [Citation34] found that reagent grade glucose can induce the synthesis of cellulase. Reagent-grade glucose contains impurities that have high induction activity for T. viride [Citation35]. After separation and purification, induction activity was highest in sophorose. Most cellulases from T. reesei are inducible enzymes [Citation36]. The leading candidate for controlling cellulolytic enzyme production in T. reesei is β-glucosidase, which is responsible for the production of an inducer, sophorose [Citation37]. The carbon source used in this experiment was microcrystalline cellulose (MCC), and its water-soluble enzymatic hydrolysis products play an inducing role in the culture process. We speculate that the effect of pH on β-glucosidase activity may be related to its water-soluble enzymatic hydrolysis products.
Cellulose saccharification is often carried out in extreme environments of high temperature, low pH or with high concentrations of inhibitors. Overall hydrolytic activity can be enhanced by thermostable enzymes through their higher specific activity and higher stability [Citation38]. β-glucosidases that are able to tolerate harsh conditions (e.g. acidic or basic pH conditions, as well as high temperatures) have greater potential for biotechnological applications in different industries, such as food [Citation39], wine [Citation40] and textile production [Citation41]. The demand for thermostable β-glucosidases is rapidly increasing, which has motivated studies on the production of this enzyme in a wide range of natural sources [Citation42]. Several kinds of acidophilic and heat-resistant β-glucosidases have been widely studied. Fritscher et al. cloned a β-glucosidase from a thermophilic reticular cyst nematode, which showed high thermal stability at 70 °C, 80 °C and 90 °C [Citation43]. Zou et al. found that a heat-resistant eosinophilic β-glucosidase still had 50% activity when incubated for 60 min at 95 °C [Citation44]. In this study, our optimized β-glucosidase exhibited promising enzymatic properties, including favorable temperature (45 °C) and pH (5.0) optima, and good thermostability (i.e. remaining stable at 30–60 °C). These unique thermostable and acidophilic properties suggest that our optimized β-glucosidase-producing T. reesei strain is an ideal candidate for biotechnological applications. When cellulase can maintain activity at higher temperatures (>60 °C) and lower pH value (4.0–5.0), it is more suitable for applications where acid and high temperatures are applied as pretreatments [Citation35].
We found that T. reesei S12 had high temperature tolerance and acid resistance, which may indicate that soil environments with rich organic matter like those in the Hainan mangroves are suitable for acidophilic and heat-resistant strains. These strains are of particular interest for their efficiency at degrading plant biomass and producing ethanol. At present, there is no existing literature on optimizing the fermentation media of T. reesei to improve the enzyme activity. The culture conditions developed in this study, which improved enzyme production 6-fold, will reduce the current production costs and aid in further applications of this important enzyme.
Conclusions
We optimized the fermentation conditions of T. reesei isolated from the surface soil of mangroves to produce β-glucosidase using response surface methodology. Based on the results obtained from Plackett-Burman experimental design, we found that the inoculum, pH and liquid volume in flask were critical environmental factors in enzyme production. The steepest ascent path determined the optimal range of their levels. In addition, we further optimized the values for these factors using the Box-Behnken design and response surface methodology. The final β-glucosidase activity was increased by 6 times compared to the starting conditions. These results provide a foundation for the further development and utilization of T. reesei β-glucosidase in mass culturing for artificial bioethanol production.
Supplemental Material
Download MS Word (22.2 KB)Acknowledgements
We would like to thank Dr. Larry Bowman at Yale University for his assistance with English language and grammatical editing.
Disclosure statement
No conflict of interest exits in this manuscript, and the manuscript was approved by all authors for publication.
Data availability statement
All data that support the findings reported in this study are available from the corresponding author upon reasonable request.
Additional information
Funding
References
- Abdel-Halim ES, Alanazi HH, Al-Deyab SS. Utilization of olive tree branch cellulose in synthesis of hydroxypropyl carboxymethyl cellulose. Carbohydr Polym. 2015;127:124–134.
- Zheng F, Tu T, Wang XY, et al. Enhancing the catalytic activity of a novel GH5 cellulase GtCel5 from gloeophyllum trabeum CBS 900.73 by site-directed mutagenesis on loop 6. Biotechnol Biofuels. 2018;11:76–89.
- Cao H, Zhang Y, Shi P, et al. A highly glucose-tolerant GH1 β-glucosidase with greater conversion rate of soybean isoflavones in monogastric animals. J Ind Microbiol Biotechnol. 2018;45(6):369–378.
- Deng P, Meng C, Wu Y, et al. An unusual GH1 β-glucosidase from marine sediment with β-galactosidase and transglycosidation activities for superior galacto-oligosaccharide synthesis. Appl Microbiol Biotechnol. 2020;104(11):4917–4927.
- Payne CM, Knott BC, Mayes HB, et al. Fungal cellulases. Chem Rev. 2015;115(3):1308–1448.
- Ausanio G, Califano V, Costantini A, et al. Matrix-Assisted pulsed laser evaporation of β-glucosidase from a dopa/quinone target. Enzyme Microb Technol. 2020;132:109414.
- Yao G, Wu R, Kan Q, et al. Production of a high-efficiency cellulase complex via β-glucosidase engineering in penicillium oxalicum. Biotechnol Biofuels. 2016;9(1):1–11.
- Huang Q, Wang K, Li H, et al. Enhancing cellulosic ethanol production through coevolution of multiple enzymatic characteristics of β-glucosidase from penicillium oxalicum 16. Appl Microbiol Biotechnol. 2020;104(19):8299–8308.
- Matsuura M, Obata A. βGlucosidases from soybeans hydrolyze daidzin and genistin. J Food Science. 1993;58(1):144–147.
- Du L, Wang Z, Zhao Y, et al. A β-glucosidase from novosphingobium sp. GX9 with high catalytic efficiency toward isoflavonoid glycoside hydrolysis and (+)-catechin transglycosylation. Appl Microbiol Biotechnol. 2014;98(16):7069–7079.
- Ko J-A, Ryu YB, Kwon H-J, et al. Characterization of a novel steviol-producing β-glucosidase from penicillium decumbens and optimal production of the steviol. Appl Microbiol Biotechnol. 2013;97(18):8151–8161.
- Gutierrez-Rosales F, Romero MAP, Casanovas MA, et al. Metabolites involved in oleuropein accumulation and degradation in fruits of Olea europaea L.: hojiblanca and arbequina varieties. J Agric Food Chem. 2010;58(24):12924–12933.
- Lenz F, Zurek P, Umlauf M, et al. Tailor-made β-glucosidase with increased activity at lower temperature without loss of stability and glucose tolerance. Green Chem. 2020;22(7):2234–2243.
- Kim JY, Hur SH, Hong JH. Purification and characterization of an alkaline cellulase from a newly isolated alkalophilic bacillus sp. HSH-810. Biotechnol Lett. 2005;27(5):313–316.
- Cao L, Chen R, Huang X, et al. Engineering of β-Glucosidase Bgl15 with simultaneously enhanced glucose tolerance and thermostability to improve its performance in High-Solid cellulose hydrolysis. J Agric Food Chem. 2020;68(19):5391–5401.
- Bischof RH, Ramoni J, Seiboth B. Cellulases and beyond: the first 70 years of the enzyme producer Trichoderma reesei. Microb Cell Fact. 2016;15(1):106–119.
- Nakazawa H, Kawai T, Ida N, et al. Construction of a recombinant Trichoderma reesei strain expressing Aspergillus aculeatus β-glucosidase 1 for efficient biomass conversion. Biotechnol Bioeng. 2012;109(1):92–99.
- Huang C, Feng Y, Patel G, et al. Production, immobilization and characterization of beta-glucosidase for application in cellulose degradation from a novel Aspergillus versicolor. Int J Biol Macromol. 2021;177:437–446.
- Qin Y, Fu Y, Li Q, et al. Purification and enzymatic properties of a difunctional glycoside hydrolase from Aspergillus oryzae HML366. Indian J Microbiol. 2020;60(4):475–484.
- Li CC, Lin FM, Li YZ, et al. A β-glucosidase hyper-production Trichoderma reesei mutant reveals a potential role of cel3D in cellulase production. Microb Cell Fact. 2016;15(1):113–151.
- Karkehabadi S, Helmich KE, Kaper T, et al. Biochemical characterization and crystal structures of a fungal family 3 β-glucosidase, Cel3A from Hypocrea jecorina. J Biol Chem. 2014;289(45):31624–31637.
- Almeida P, Oliveira T, Lucas R, et al. Heterologous production and biochemical characterization of a new highly glucose tolerant GH1 β-glucosidase from Anoxybacillus thermarum. Process Biochem. 2020;99:1–8.
- Wang K, Huang Q, Li H, et al. Co-evolution of β-glucosidase activity and product tolerance for increasing cellulosic ethanol yield. Biotechnol Lett. 2020;42(11):2239–2250.
- Amdoun R, Sahli F, Hamadache K, et al. Optimization of caulogenesis in populus nigra under lead (Pb) stress via response surface methodology (RSM) and desirability function analysis. Plant Cell Tiss Organ Cult. 2020;142(1):41–50.
- Mattéotti C, Thonart P, Francis F, et al. New glucosidase activities identified by functional screening of a genomic DNA library from the gut microbiota of the termite reticulitermes santonensis. Microbiol Res. 2011;166(8):629–642.
- Khalili E, Huyop F, Manan FMA, et al. Optimization of cultivation conditions in banana wastes for production of extracellular β-glucosidase by trichoderma harzianum rifai efficient for in vitro inhibition of macrophomina phaseolina. Biotechnol Biotechnol Equip. 2017;31(5):921–934.
- Box G, Hunter JS, Hunter WG. Statistics for experimenters: Design, innovation, and discovery. Hoboken, NJ: Wiley; 2005.
- Zhang GZ, Yang HT, Wen CJ. The key to the morphological classification and the molecular identification of trichoderma sp. J Shandong Agric Univ (Nat Sci Ed). 2011;42(2):309–316.
- Chakraborty S, Saha A, Neelavar Ananthram A. Comparison of DNA extraction methods for non-marine molluscs: is modified CTAB DNA extraction method more efficient than DNA extraction kits? 3 Biotech. 2020;10(2):69.
- Ceccon DM, Faoro H, Lana P. D C, et al. Metataxonomic and metagenomic analysis of mangrove microbiomes reveals community patterns driven by salinity and pH gradients in paranaguá Bay, Brazil. Sci Total Environ. 2019;694:133609.
- Yazici SO, Sahin S, Biyik HH, et al. Optimization of fermentation parameters for high-activity inulinase production and purification from rhizopus oryzae by Plackett-Burman and Box-Behnken. J Food Sci Technol. 2021;58(2):739–752.
- Wang J. Effect of liquid farmentation factors and deforming agent on conidial production by Trichoderma asperellum stain Tr148c. (M.D Dissertation). Anhui Agricultural University, Anhui, China. 2016.
- Han WQ, Wang F, Tian TW, et al. The effect of inoculum concentration and liquid volume on fumaric acid prodution from raw tapioca flour by Rhizopus arrhizus. J Beijing Univ Chem Technol (Nat Sci). 2011;38(5):95–99.
- Mandels M, Reese ET. Induction of cellulase in fungi by cellobiose. J Bacteriol. 1960;79(79):816–826.
- Kubicek CP, Mikus M, Schuster A, et al. Metabolic engineering strategies for the improvement of cellulase production by hypocrea jecorina. Biotechnol Biofuels. 2009;2(1):19–33.
- Havukainen S, Valkonen M, Koivuranta K, et al. Studies on sugar transporter CRT1 reveal new characteristics that are critical for cellulase induction in Trichoderma reesei. Biotechnol Biofuels. 2020;13:120–158.
- Luo Y, Valkonen M, Jackson RE, et al. Modification of transcriptional factor ACE3 enhances protein production in Trichoderma reesei in the absence of cellulase gene inducer. Biotechnol Biofuels. 2020;13(1):137–153.
- Jang WJ, Lee GH, Lee JM, et al. Improving enzyme activity, thermostability and storage stability of β-1,3-1,4-glucanase with poly-γ-glutamic acid produced by bacillus sp. SJ-10. Enzyme Microb Technol. 2021;143:109703–109710.
- Bhatia Y, Mishra S, Bisaria VS. Microbial beta-glucosidases: cloning, properties, and applications. Crit Rev Biotechnol. 2002;22(4):375–407.
- Palmeri R, Spagna G. β-Glucosidase in cellular and acellular form for winemaking application. Enzyme Microb Technol. 2007;40(3):382–389.
- Song JY, Imanaka H, Imamura K, et al. Development of a highly efficient indigo dyeing method using indican with an immobilized beta-glucosidase from Aspergillus niger . J Biosci Bioeng. 2010;110(3):281–287.
- Bien-Cuong D, Dang TT, Berrin JG, et al. Cloning, expression in Pichia pastoris, and characterization of a thermostable GH5 mannan endo-1,4-beta-mannosidase from Aspergillus niger BK01. Microb Cell Factories. 2009;8(1):59–59.
- Fritscher C, Messner R, Kubicek CP. Cellobiose metabolism and cellobiohydrolase I biosynthesis by Trichoderma reesei. Exp Mycol. 1990;14(4):405–415.
- Zou ZZ, Yu HL, Li CX, et al. A new thermostable β-glucosidase mined from Dictyoglomus thermophilum: Properties and performance in octyl glucoside synthesis at high temperatures. Bioresour Technol. 2012;118:425–430.