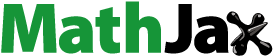
Abstract
One of the deadliest forms of malignancy affecting females worldwide alongside breast cancer is cervical cancer, which urgently calls for novel treatment approaches. Gold nanocarriers are attaining acceptance due to their inert nature, minimal side-effects, low cytotoxicity and easy fabrication. Synthesis of gold nanorods conjugated with graphene and folic acid was carried out, which were finally loaded with bevacizumab for improved therapeutics. The drug loaded folic acid conjugated graphene-gold nanorods were irradiated with non-infrared laser for improved penetration of the drugs into the specifically targeted tissue. The graphene-gold nanorods with/without drugs and irradiation at different power densities (0.25 and 0.5 W/cm2) were studied. Transmission electron microscopy, zeta potential and polydispersity index established the integrity of prepared graphene nanorods carriers. The encapsulation efficiency and drug loading of the graphene-gold nanorods were determined. In vitro cytotoxicity assay was carried out via 3‐(4,5‐dimethylthiazol‐2‐yl)‐2,5‐diphenyltetrazolium bromide, or MTT assay, with HeLa cells. There was non-significant difference between drug loading and encapsulation with irradiated and non-irradiated graphene-gold nanorods. But with irradiation the blank graphene-gold nanorods had increased cytotoxicity. The graphene-gold nanorods were released in a sustained manner as depicted by in vitro release studies. The drug loaded nanorods were also internalized by HeLa cells and distributed within the cellular compartment. We also tested drug loaded folic acid conjugated graphene-gold rods in vivo in U8GMG tumor bearing mice and results clearly indicated the irradiated ones were most efficient in suppressing tumors.
Introduction
Cervical cancer is one of the most frequent and the deadliest form of malignancy alongside breast cancer. Both are associated with excessive mortality and hence the target for regular pioneering inventions in nanobiomedicine. Human Papillomavirus (HPV) infections are generally the causing factor of cervical carcinoma and approximately 75% of cases echo HPV infections as a single risk cause [Citation1]. The arrival of several vaccines as preventive measures for cervical cancer does not significantly change the situation owing to their unavailability across several areas worldwide making the management sufficiently difficult [Citation2–5]. There has not been any noteworthy difference in the overall situation despite progress in diagnosis and treatment. Some areas of therapeutics need urgent and predominant attention. The approaches for treatment of cervical cancer are manifold, including surgery, radiation therapy, chemotherapy and targeted drug delivery [Citation6, Citation7]. Chemotherapy is a dominant and potent therapeutic approach for cervical carcinoma. Targeted drug delivery aims at seeking various nanoformulations which suppresses carcinoma and they are noted to cause fewer side effects than the conventional chemotherapy. It has also been increasingly noticed that a single drug may not be effective in eliminating the cancer cells and hence requires the aid of combinatorial formulations where two or more anticancer drugs are encapsulated in a nanocarrier.
Photothermal therapy has been emerging as an essential tool for treatment of carcinoma [Citation8]. Photothermal effect may be defined as conversion of non-infrared [Citation9] light, which has greater penetration in tissues, to heat radiation [Citation10]. The near infrared (NIR) absorbing nanomaterials are targeted to the cancerous tissues. Then irradiation with laser causes thermal heat ablation of cancer cells selectively since they have increased sensitivity to heat than normal cells [Citation9]. The targeting of these NIR-absorbing nanomaterials conjugated with folic acid may be extremely helpful since studies have depicted very high targeting of folic acid to several carcinomas including cervical carcinoma owing to the presence of more abundant folate receptors than normal cells [Citation11–14]. Moreover, a widely explored theranostic area is localized surface plasmon resonance (LSPR), which is exhibited by several metal nanoparticles, and has been applied for photothermal therapy of cancer [Citation15]. Amongst them, several studies have reported gold nanovehicles which have increased plasmonic effect and better tunable properties owing to the durable plasmonic coupling of the gold nanoparticles in the vesicular surface [Citation16]. Surrounded by a huge number of gold nanostrategies [Citation17–19], gold nanorods have attracted importance owing to their absorption peaks which lie in the infrared-region [Citation20]. Along with gold, graphene oxide nanoparticles have also been explored for drug encapsulation and enhanced photothermal therapy for anticancer therapeutics [Citation21]. The advantage with graphene oxide is that it is an excellent drug carrier where both hydrophobic and hydrophilic drugs may be loaded by hydrophobic-hydrophobic interactions or π-π stacking [Citation22, Citation23]. Even though graphene oxide can absorb light in NIR region and then via nonradioactive decay, release the same as heat, due to the broad absorption spectrum and little quantum efficiency comparatively low photothermal conversion efficiency has been reported [Citation24]. It has also been studied that the photothermal efficacy of graphene oxide may be improved if applied as a coating/anchored on the surface of a plasmonic nanoparticles [Citation25, Citation26].
Therefore, in our study we have created a one-of-its-kind drug delivery vehicle consisting of folic acid conjugated graphene-gold nanorods and loaded with an anticancer drug, bevacizumab. Bevacizumab is a potent anticancer drug, an immunoglobulin which targets and blocks all isoforms of vascular endothelial growth factor-A (VEGF-A), which plays an active role in cancer growth and metastasis [Citation27, Citation28]. This, is turn impedes the flow of blood to the tumor tissues and decreases their growth. However, bevacizumab is associated with high cytotoxicity and low tumor penetration [Citation29, Citation30]. So, we hypothesized that encapsulating graphene oxide and bevacizumab with the nanorods would probably reduce the cytotoxicity by avoiding direct contact of the graphene oxide and bevacizumab with normal tissues in physiological environment and also enhance the photothermal effect of the graphene oxide encapsulated with the gold nanorods.
Materials and methods
Materials
Hydrogen tetrachloroaurate (III) trihydrate (HAuCl4·3H2O), cetyltrimethylammonium bromide (CTAB, 98%), ascorbic acid (99%), sodium borohydride (96%), sodium citrate (99%), silver nitrate (99%), polyvinyl alcohol (PVA, MW 9,000 ∼ 10,000), Chloroacetic acid (ClCH2COOH), folic acid, N-(3-dimethylamino propyl-N0-ethylcarbodiimide) hydrochloride (EDC-HCl), N-hydroxysuccinimide (NHS) and hydrazine hydrate (50-60%) were procured from Sigma-Aldrich, China. All other solvents unless otherwise mentioned were procured from China. Trypsin, 3-(4, 5-dimethylthiazol-2-yl)-2,5-diphenyl-ltetrazolium bromide (for MTT), cell culture Dulbecco’s Modified Eagle Medium (DMEM), penicillin, fetal bovine serum (FBS) and streptomycin were acquired from Gibco Invitrogen. Bevacizumab was procured from China National Medicine Corporation and utilized as established. Procurement of the dialysis bags (MWCO = 10,000) was done from Spectrum Laboratories Inc.
Synthesis of folic acid conjugated reduced graphene oxide (FA-GO)
The preparation followed the procedure from Song et al. [Citation15], which is modified Hummer’s method [Citation31] explained in detail below. This is essentially a rapid and safe method which while keeping the original process intact has been modified over the years to produce graphene oxide conjugate. Ten milliliters of aqueous solution of graphene oxide at 2 mg/mL concentration was sonicated for 1 h following addition of NaOH (0.1 g/mL) for solution neutralization. The graphene oxide was centrifuged several times for purification and finally dispersed in Milli-Q water. The carboxylation of the obtained graphene oxide was carried out by addition of NaOH (1.2 g) and ClCH2COOH (1.0 g). This was followed by bath sonication at 500 W for 3 h for conversion of OH groups to COOH. Dilute hydrochloric acid was utilized for neutralization of graphene oxide–COOH solution. Further purification was done by repeated rinsing and filtrations. Next, EDC and NHS were mixed with graphene oxide-COOH solution (10 mL, 1 mg/mL) followed by sonication for 2 h. Subsequently, 0.5% FA (2 mL, with pH adjusted to 8.0 by NaHCO3 solution) was added and the overnight stirring of folic acid conjugated with graphene oxide (FA-GO) mixture was done.
Preparation of bevacizumab loaded FA-GO (Be-FA-GO)
One milliliter of aqueous solution of bevacizumab (2 mg/mL) was gradually mixed into 1 mL of FA-GO (0.5 mg/mL) aqueous solution, and the resultant solution was continuously stirred in darkness for 12 h. The obtained Be-FA-GO was stored at 4 °C for further use. Free and excess bevacizumab was separated by recurrent washes and ultrafiltration with a 100 kDa filter (Millipore).
Preparation of gold nanorods
Five milliliters of 0.2 mol/L Cetyltrimethylammonium bromide (CTAB) was mixed with deionized water, stirred at 30 °C for 5 min until clear followed by addition and stirring of 5.0 mL gold(III) chloride trihydrate (HAuCl4·3H2O) (1.0 mmol/L) for 5 min. Then, 300 µL of 4.0 mmol/L AgNO3 and 12.0 µL of 37% HCl were added to the above solution. Subsequently, a solution of 75 µL of 85 mmol/L ascorbic acid was quickly mixed with the solution, followed by injection of 7.5 µL of 0.01 mol/L NaBH4. The solution was kept still for 6 h. Then, gold nanorods (AuN) were purified by many centrifugation cycles followed by a wash with deionized water. The procedure was followed with some changes from Song et al [Citation15].
Final conjugation of Be-FA-GO-AuN
The double emulsion method (water in oil in water) was performed as elaborated by Song et al. [Citation15] with certain changes to suit our study. Be-FA-GO (0.5 mg) was mixed with 0.14 mL of deionized water, which forms the internal aqueous phase, W1, and further mixed with 1 mL of chloroform, which forms the oil phase, O, comprising 5 mg AuN. Pulsed sonication at 100 W and 23 KHz was applied for the synthesis of major W1/O emulsion (MISONZX ultrasonic liquid processors) for 1 min. An aqueous solution of Be-FA-GO (W1) was emulsified within the chloroform solution containing amphiphilic AuN. It was instantaneously transferred into 5 mL of 2% (w/v) PVA aqueous solution, which was the re-emulsification solution, W2, and again homogenized for 1 min at 6500 rpm to finally form a W1/O/W2 double emulsion. For evaporation of the oil phase, the prepared emulsion was additionally agitated at 25 °C for 12 h. Upon the removal of chloroform, Be-FA-GO was loaded onto the inner surface of the AuN. After complete removal of chloroform, which was confirmed by the clearing of mixture, the resulting Be-FA-GO-AuN was cleaned by centrifugation at 3500 g for 5 min thrice (MISONZX ultrasonic liquid processors, XL-2000 series). The obtained Be-FA-GO-AuN was dispersed in deionized water for further use. Only FA-GO-AuN was also prepared without loading drug bevacizumab.
Characterization of Be-FA-GO-AuN
The morphological analysis of FA–GO–AuN was done by JEM-2100HR transmission electron microscopy [Citation30] operated at 200 kV before and after irradiation with 808 nm laser at power densities of 0.25 W/cm2 and 0.5 W/cm2 for 3 min. The average size distributions of Be-FA-GO-AuN and FA-GO-AuN were calculated using a Malvern zeta sizer (Malvern Instrument Inc.). Dilutions of the specimens were done with PBS, which was uniformly dispersed with Milli-Q water, after which the analysis was carried out. The zeta potential of Be-FA-GO-AuN and FA-GO-AuN was also determined. The measurements were accomplished by means of a cell dipped in aqueous solution in a programmed way involving addition of diluted specimens in the cell for capillary measurement and attuning the locus of cell. The experiments were carried out in triplicate.
Raman spectra of Be-FA-GO-AuN and FA-GO-AuN were determined by means of a confocal Raman microspectrometer (UK) with a laser source of 514 nm. Graphene oxide and its optical properties were portrayed using an ultraviolet–visible (UV–Vis) absorbance spectrometer (NanoDrop, ND-1000). FTIR spectra were determined via a Nicolet 6700 FT-IR spectrometer.
Drug loading and encapsulation efficiency
The encapsulation efficiency of Be-FA-GO-AuN was calculated from Dixit et al. [Citation32] with a few modifications. Ten milliliters of distilled water were used to dissolve 50 mg of Be-FA-GO-AuN sample. The samples were heated for 5 min and kept immobile for 48 h following which ultracentrifugation of the sample was carried out (Thermo Fischer Scientific) at 40,000 rpm for 2 h. A 0.22-mm membrane filter (MDI, India) was used to filter the supernatant obtained from each sample. The absorbance of the Be-FA-GO-AuN sample was measured at 280 nm using an UV/Visible spectrophotometer. The measurements were carried out thrice (n = 3).
To calculate the drug-loading capacity and drug encapsulation efficiency, the following formulas were applied:
In vitro release assay
The in vitro release assay was carried out in two different pHs, 7.4 and 4.0 to simulate the normal physiological environment and tumor microenvironment respectively. It was also investigated at different power densities of 808 nm laser radiation. To examine the NIR laser- and pH activated bevacizumab release behaviors, 2 mL of Be-FA-GO-AuN aqueous solution was moved in a dialysis tube with a membrane cut-off of 3000 Da. The dialysis tube was then submerged in 15 mL deionized water contained within a 25-mL bottle. The NIR laser-activated bevacizumab release investigation was done with 808 nm laser irradiation at power densities 0.25 W/cm2, 0.5 W/cm2, 0.75 W/cm2 and 1.0 W/cm2 for 3 min. For the pH activated bevacizumab release, the pH of the solution of the glass bottle was adjusted to 4.0 by addition of HCl (1 mol/L) solution. The amount of bevacizumab released over different time periods (4, 8, 16, 24 h) was determined by analysis of the UV absorbance of bevacizumab at 280 nm.
In vitro chemo-photothermal treatment of HeLA cells
Cell culture
The HeLa cell line (human cervical cancer) was procured from ATCC USA (CRM-CCL-2™). The cells were cultured in RPMI 1640 medium at 37 °C with 5% CO2 in a humid or moist milieu. FBS (10%) was supplemented to the RPMI 1640 for cytotoxicity analyses besides 2 mmol/L L-glutamine, 50 UI/mL penicillin and 50 UI/mL streptomycin [Citation33, Citation34]. The medium was renewed every 2-3 days per week. The medium was incubated with trypsin to remove the confluent cells and they were seeded at 300 μL per well into 96-well plates at a density of 106 cells/mL. The cells were then exposed to: PBS (control, with and without laser), FA-GO-AuN (without laser), FA-GO-AuN (with laser, power density 0.25 W/cm2), FA-GO-AuN (with laser, power density 0.5 W/cm2), Be-FA-GO-AuN (without laser), Be-FA-GO-AuN (with laser, power density 0.25 W/cm2), Be-FA-GO-AuN (with laser, power density 0.5 W/cm2). The cultures were kept at 37 °C for 24 h for the cells to attain confluency thereby attaching themselves to the well plates.
MTT assay
As described above, more confluent cells were cultured. MTT assay was the preferred one for in vitro cytotoxicity evaluation. Cells were harvested at 5 × 103 cells/well in 96-well plates followed by 72 h incubation to obtain viable cells at the time of following addition; FA-GO-AuN (0.1 mL), Be-FA-GO-AuN (0.1 mL) and PBS (0.1 mL). One plate of each was kept without laser treatment, while the others of each were subjected to 808 nm laser treatment with different power of 0.25 W/cm2 and 0.5 W/cm2, respectively, for a time period of 3 min (4 interventions in 2 h) as reported by You et al. [Citation35]. Further incubation of the cells was carried out for 48 h. Thereafter, 20 μL of MTT solution (5 mg/mL) was added to each well and the plate was incubated at 37 °C for 4 h. Measurement of the absorbance was carried out at 492 nm using a microplate reader after the incubation.
In vivo combined chemo-photothermal cancer therapeutic approach
Ethics statement
The animal studies were done under the strict supervision of the institutional Ethics Committee (Ethical Clearance No. 117/IAEC/HUM/2019). The whole protocol was thoroughly reviewed and approved by the Committee before commencing the experiments.
Procedure
Combined chemo-photothermal therapeutic effect in vivo was studied using U87MG-tumor-bearing mice. The mice were divided into 7 groups of 2 animals each. The animals were kept in the animal care facility of the Department. The mice were housed in sterile cages under laminar flow hoods in a room with a 12 h light/12 h dark cycle and controlled temperature. They were fed autoclaved chow and water ad libitum. The animals remained in the home cages throughout the experiment. Each mouse was subcutaneously injected with U87MG glioblastoma cell line obtained from American Type Culture Collection (ATCC), USA (10 × 106 cells/mouse). The cells were injected in the dorsolateral region near the midrib of mice, and tumor volume was approximately grown to 60 mm3. Body weight changes in the mice groups were recorded every day. Thereafter the mice were treated intravenously with the following: (1) PBS only; (2) FA-GO-AuN (without laser); (3) FA-GO-AuN (with laser, power density 0.25 W/cm2); (4) FA-GO-AuN (with laser, power density 0.5 W/cm2), (5) Be-FA-GO-AuN (without laser); (6) Be-FA-GO-AuN (with laser, power density 0.25 W/cm2); (7) Be-FA-GO-AuN (with laser, power density 0.5 W/cm2); (8) free bevacizumab (without laser); free bevacizumab (with laser, power density 0.25 W/cm2) and free bevacizumab (with laser, power density 0.5 W/cm2). The irradiation with laser was carried out for 3 min in all cases. A digital Vernier caliper was used to quantify the tumor size at 5-day interludes after the laser irradiation. The tumor volume V (mm3) was deliberated using the following formula:
Where L and W are the length and width of the tumor in millimeters. Then the percentage of tumor volume was calculated.
Data analysis
Measurement of tumor volume and body weight change was done from day 0 to day 24. Evaluation of any notable variation in the animals with respect to tumor volume and body weight change was calculated using analysis of variance (ANOVA). Differences were considered statistically significant at the level of p < 0.05.
Results
Preparation and characterization of Be-FA-GO-AuN
We followed a slightly modified double emulsion method for synthesis of Be-FA-GO-AuN (W1/O/W2). The prepared bevacizumab loaded folic acid conjugated graphene oxide and gold nanorods conjugate were characterized by particle size distribution, morphology and size. The TEM micrographs in depict that the Be-FA-GO-AuN and FA-GO-AuN are regular, elliptical in shape without any agglomerations. They are homogenously distributed. However, upon irradiation with laser at 0.5 and 0.25 W/cm2 for 3 min noticeable changes occurred. These are depicted in , which show disrupted nanoparticles. The disruption of size and shape led to irregularly shaped nanorods. The heat-inducing photothermal effect of the NIR irradiation will be discussed later. The size and zeta potential of the Be-FA-GO-AuN and FA-GO-AuN are listed in . The sizes are approximately 150 nm and 60 nm of Be-FA-GO-AuN and FA-GO-AuN with polydispersity index (PDI) of 0.026 and 0.017, respectively. The zeta potential of Be-FA-GO-AuN and FA-GO-AuN was calculated to be approximately −25.3 and −19.2 mV, respectively.
Figure 1. TEM micrographs of FA-GO-AuN (A); Be-FA-GO-AuN (B); FA-GO-AuN (C) irradiated with 808 nm Laser with power density of 0.25 W/cm2; Be-FA-GO-AuN (D) irradiated with 808 nm Laser with power density 0.25 W/cm2; 1E. FA-GO-AuN (E) irradiated with 808 nm Laser with power density 0.5 W/cm2; and Be-FA-GO-AuN (F) irradiated with 808 nm Laser with power density 0.5 W/cm2.
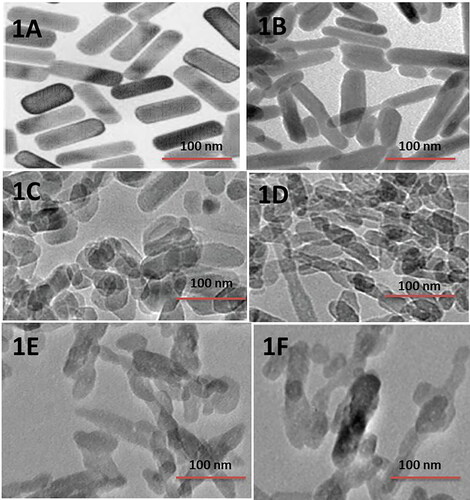
Table 1. Average particle size, zeta potential and PDI of Be-FA-GO-AuN and FA-GO-AuN.
The FTIR results are shown in . In accordance with the bevacizumab spectra, the specific peaks in Be-FA-GO-AuN were seen at 2930 cm−1, which corresponds to C–H stretching, 1646 cm−1 and 1553 cm−1, which correspond to amide I and II, respectively) [Citation36]. This indicated that bevacizumab was loaded successfully into the nanoparticles. However, in both Be-FA-GO-AuN and FA-GO-AuN, the FA spectra which corresponded to the bands between 1481 and 1511 cm−1 were seen. This related to the specific absorption peaks of phenyl and PT ring. The band at 1601 cm−1 corresponds to the NH-bending band. The 1691 cm−1 corresponded to the peak of C = O. Several small shifts occurred in Be-FA-GO-AuN and FA-GO-AuN between 3000 cm−1 and 3600 cm−1, which successfully proved that FA is also conjugated with the drug loaded graphene oxide conjugated gold nanorods.
The presence of bevacizumab in the drug loaded solution is accountable for the UV absorption in the region of 310 nm to 400 nm as seen in [Citation37]. The presence of bevacizumab in Be-FA-GO-AuN is the factor responsible for the absorbance at 350 nm.
Raman spectrum of bevacizumab, FA-GO-AuN and Be-FA-GO-AuN were observed in . The spectra were obtained in the range of 1100-1900 cm−1 with 10 s exposure time and spectral accumulation of 16 cm−1. The strongest peak observed was that corresponding to Au at 1596 cm−1. The other peak at 1636 cm−1 corresponded to aromatic stretching vibration of carbonyl groups (C = O). The FA groups corresponded to the peaks at 1236, 1376 and 1590 cm−1, which was due to rocking vibration of C = N, C = C asymmetric stretching vibration and aromatic stretching vibration of C = O carbonyl groups.
Drug-loading and encapsulation efficiency
The encapsulation efficiency and drug loading capacity of Be-FA-Go-AuN is depicted in . The encapsulation efficiency was 92% and the drug-loading capacity was calculated to be 45%. The values indicated efficient encapsulation of bevacizumab within the FA-GO-AuN.
Table 2. Encapsulation efficiency and drug loading capacity of Be-Fa-GO-AuN.
On-demand in vitro drug release
The on-demand in vitro drug release exhibited a very interesting trend. It was seen that the Be-FA-GO-AuN exhibited a pH dependent and NIR laser irradiation dependent drug release. In fact, this study was performed immediately after the preparation of Be-FA-Go-AuN to understand which power densities of NIR laser may be used for further studies. Four different power densities of NIR 808 nm laser were used to study the in vitro drug release behaviour. We will view the results first in pH 7.4. It was observed that at 4 h after irradiation, the nanorods without irradiation and those irradiated at 0.25 W/cm2 released a minimum amount of drug, whereas those irradiated with 1.0 W/cm2 exhibited an abnormal burst release of drug. During the subsequent time intervals, the drug released from Be-FA-GO-AuN was sufficient at 0.25 and 0.5 W/cm2, but quite abnormal at 0.75 W/cm2 releasing almost all the amount of drug loaded. The amount of drug released at 1.0 W/cm2 was also minimal (). The results of pH 4.0 followed a similar trend as exhibited in . There is one exception though. There is a burst release of drug after 4 h after irradiation with 0.75 and 1.0 W/cm2. However, the release of drug was steady after irradiation with 0.25 and 0.5 W/cm2 power densities over a time period of 24 h. Hence, we proceeded with these two power densities for the other studies.
MTT assay
The therapeutic cytotoxicity of the FA-GO-AuN and Be-FA-GO-AuN was clearly expressed in the MTT assay (). It was observed that PBS with or without irradiation was not significantly cytotoxic to the cancer cells. Free Bevacizumab with and without radiation were cytotoxic toward the HeLa cells. However, upon irradiation with different power densities, the cytotoxic effect was reduced, which was an interesting observation. The FA-GO-AuN without irradiation resulted in about 55% viable cells, which decreased to 46% with irradiation at 0.5 W/cm2 and 51% with 0.25 W/cm2. On treatment with Be-FA-GO-AuN without irradiation, the viable cells were 35%, which decreased to 22% upon treatment with 0.25 W/cm2 and 18% upon irradiation with 0.5 W/cm2. It was therefore noted that irradiation with different power densities caused noticeable changes toward the cytotoxicity to HeLa cells.
In vivo chemo-photothermal assay
The in-vivo chemo photothermal assay images are shown in representing the tumour sizes and representing the tumor volume. After the intravenous administration of PBS, FA-GO-AuN, Be-FA-GO-AuN (with and without laser at different power densities of 0.5 and 0.25 W/cm2) and free bevacizumab (with and without laser at different power densities of 0.5 and 0.25 W/cm2), the tumor volumes were recorded every 5 days for 25 days. The tumors treated with PBS (with/without irradiation) grew rapidly showing no visible signs of inhibition. In 25 days, the tumor volume increased by approximately 200%. Even, FA-GO-AuN with irradiation did not show any signs of reduction of tumor volume, although the increase in size was nominal (25%). Without irradiation, there was increase in tumor size albeit less than that in the control. However, the power density of 0.5 W/cm2 showed a greater efficiency in keeping the size in control. With Be-FA-GO-AuN without irradiation, the tumor size remained almost the same. But with irradiation at power density of 0.25 W/cm2, the size of tumor decreased by 38%, whereas with irradiation at a power density of 0.5 W/cm2, the tumor volume decreased by almost 85%. Therefore, the tumors treated with Be-FA-GO-AuN had the smallest tumor volumes and two of the mice exhibited tumor ablation. With free bevacizumab, the non-irradiated tumors showed much less decrease in tumor volume, lesser than the ones irradiated with different power densities of 0.5 and 0.25 W/cm2. The highest tumor reduction was therefore obtained by treatment with Be-FA-GO-AuN irradiated at a power density of 0.5 W/cm2. There was an apparently little body weight fluctuation observed in the mice in all the groups ().
Figure 7. Tumor sizes in mice upon treatment with Be-FA-Go-AuN, FA-GO-AuN, free bevacizumab and PBS non-irradiated and irradiated at different power density of 0.25 W/cm2 and 0.5 W/cm2.

Discussion
A life-threatening malady like cervical cancer would generally require a multi-therapeutic approach along with chemotherapy to restrict and regress its diversifications. However, the chemotherapeutic regimens are associated with such cytotoxic side-effects that a big question on its utility and efficacy occurs. Therefore, what we require today is targeted cytocompatible chemo-photothermal drug conjugated therapy. In our study, we have designed a similar approach. The targeted drug delivery was due to the FA binding to the drug loaded graphene oxide conjugated AuN. The malignant cells would have an elevated level of FA, which would take up the Be-FA-GO-AuN without being endocytosed by other normal cells, which have low levels of FA receptors [Citation38]. These drug-loaded graphene oxides conjugated gold nanorods were aimed toward a targeted controlled release of bevacizumab aiming toward higher loading efficiency, small mean particle size (150 nm), a little PDI (0.026) and a negative value of zeta potential (-21 mV). The negative charge of the nanorods was the deprotonated end carboxylic acid groups of folic acid. However, there was an almost 150% increase in the size of the nanorods upon drug loading, which was due to large size of the bevacizumab (149 kDa and mean radius 21.4 nm) [Citation39]. This also indicated a high adsorption of the drug on the inner surface of the nanorods. The usage of the term “adsorption” instead of “absorption” was as per the deductions from the results of in vitro drug release study. The negative value (-21 mV) of the zeta potential is an indication that the formulation is stable because at least −20 mV is required for the formulation to be stable [Citation27, Citation40]. The nanorods Be-FA-GO-AuN and FA-GO-AuN are smooth, regularly elliptical in shape, which was less affected by shear, and are easily taken up by the cells because of the better interaction with the cells due to the shape. Upon treatment with 808 nm laser irradiation at 0.25 and 0.5 W/cm2, the Be-FA-GO-AuN and FA-GO-AuN were damaged due to the heat generation, which resulted in the irregular shape. This was also indicative that upon laser irradiation, the release of bevacizumab from the nanorods would be easier, which would have been difficult otherwise because of the large size of the bevacizumab molecules. Additionally, it was noted in the FTIR peaks that the stretching of the COOH group on the graphene oxide corresponded to the 1691 cm−1, which was also due to sp2 arrangement in the basal plane of graphene oxide. The stretching and small shifting of the peaks also were indicative of the functionalization of FA with the graphene oxide sheets which improved the biocompatibility too. Raman spectroscopy was done to ascertain the surface vibrations and rotational frequency of the nanorods. It also helped us to understand the structural fingerprints of bevacizumab, FA-GO-AuN and Be-FA-GO-AuN. The spectra clearly indicated the conjugates were evidently attached with the AuN, which may be used for targeted bevacizumab delivery to cervical cells.
The on demand in vitro drug release behaviors revealed some interesting possibilities. The pH range was deliberated based on the normal physiology and the diseased condition of the human body. These two pH values are of extreme biological significance. It was revealed that the decrease in pH led to increased drug release over a 24 h’ time. It was also understood that power densities beyond 0.5 W/cm2 (0.75 and 1.0 W/cm2) led to excessive heat generation resulting in immediate destruction of Be-FA-GO-AuN, which caused the burst release of drugs. The destruction of Be-FA-GO-AuN was essentially caused by polymer degradation, which may be influenced by varied factors like enzymatic hydrolysis/cleavage and physico-chemical properties of the polymer [Citation41]. This abnormal release of drugs may also be due to the weak electrostatic attraction between bevacizumab and the GO conjugated nanorods. The drug was in all probability adsorbed on the inner surface of the nanorods and not absorbed in the core. Upon stimulation with irradiation at power densities of 0.25 and 0.5 W/cm2, there was sufficient heat release to cause the steady release of the drug. In the non-irradiated Be-FA-GO-AuN, the change/decrease in pH had altogether non-significant effect as there was minimal release of drugs.
The in vivo chemo-photothermal therapy explained a lot of the observations. The rapid increase in size of tumors exhibiting no signs of tumor suppression upon treatment with PBS (with/without irradiation) was due to the absence of drug. However, upon treatment with free bevacizumab without irradiation, the size did not reduce significantly since the therapy was probably not targeted with folic acid and the drug may not have accumulated in the tumors. Upon irradiation, a similar trend was observed, which led us to believe that irradiating with even lowest power density may have caused some irreversible changes in the drug which has led to loss of functionality or unavailability of accumulation in the targeted tissue. The rest of the formulations were effective in reducing the tumor size albeit nominally as per the release of drug as viewed in the in-vitro release study. They did not exhibit very high anticancer potency indicating that single therapy of either drug or irradiation may not be effective enough to reduce the tumor size. The highest tumor reduction capacity was observed in Be-FA-GO-AuN (with power densities of 0.25 and 0.5 W/cm2), which was due to the synergistic effect of drug, FA and gold nanorods (synergistic chemo photothermal therapy). This suggests that the NIR laser at different power density and tumor microenvironment were responsive of irradiated Be-FA-GO-AuN, which stimulated the on-demand release and synergistic effects of drug, FA, GO, gold nanorods along with NIR irradiation. Be-FA-GO-AuN could be suitable for a tumor ablation therapeutic approach, signifying that NIR-light induced an augmentation in the efficacy of drug delivery which had a toxic effect against cancer cells. Another significant observation is that little body weight loss was observed at the end of 25 days, indicating no significant damage/cytotoxicity to other body organs. There were also no visible signs of distress/trauma to the animals at the end of the study.
Conclusions
We propose a novel therapeutic approach for cervical cancer treatment with bevacizumab loaded FA and GO conjugated gold nanorods or Be-FA-GO-AuN as a synergistic chemo-photothermal therapy. This constituted of passive tumor targeting by Be-FA-GO-AuN by conjugation of FA, which would be taken up by the FA receptors expressed selectively in the cancerous tissues. These Be-FA-GO-AuN exhibited pH and laser irradiation dependent drug release behavior, which showed limited cytotoxicity due to minimization of the non-specific spread of the drug in the tissues. The prepared nanocarriers also exhibited greatly reduced tumors upon irradiation at certain power densities. They also are more potently cytotoxic toward the cancerous HeLa cells upon irradiation at different power densities. Further studies of this novel therapeutic approach may focus on multimodal imaging of the nanocarriers and the detailed understanding of the optimal conditions of chemotherapy along with photothermal therapy. Dual modality of photothermal therapy and optical imaging of these nanocarriers may also be focussed in order to improve the approach.
Ethical clearance for animal trials
Ethical Clearance No. 117/IAEC/HUM/2019 awarded by Institute Small Animal Ethics Committee, Hubei University of Medicine.
Acknowledgment
The authors would like to thank Taihe Hospital and The First Clinical College (affiliated to Hubei University of Medicine) for providing the necessary resources for this work.
Disclosure statement
No potential conflict of interest was reported by the author(s).
Data availability statement
The raw data used in this research may be made available by direct requests to the corresponding author.
Funding
The author(s) reported there is no funding associated with the work featured in this article.
References
- Tomoaia G, Horovitz O, Mocanu A, et al. Effects of doxorubicin mediated by gold nanoparticles and resveratrol in two human cervical tumor cell lines. Colloids Surf B Biointerfaces. 2015;135:726–734.,
- Farooq MU, Novosad V, Rozhkova EA, et al. Gold nanoparticles-enabled efficient dual delivery of anticancer therapeutics to HeLa cells. Sci Rep. 2018;8(1):12.
- Schiller JT, Castellsagué X, Garland SM. A review of clinical trials of human papillomavirus prophylactic vaccines. Vaccine. 2012;30:F123–F38.
- Hildesheim A, Herrero R, Wacholder S, Costa Rican HPV Vaccine Trial Group, et al. Effect of human papillomavirus 16/18 L1 viruslike particle vaccine among young women with preexisting infection: a randomized trial. Jama. 2007;298(7):743–753.
- Moss C, Kaye SB. Ovarian cancer: progress and continuing controversies in management. Eur J Cancer. 2002;38(13):1701–1707.
- Wang J. Combination treatment of cervical cancer using folate-decorated, ph-sensitive, carboplatin and paclitaxel co-loaded lipid-polymer hybrid nanoparticles, drug design. Drug Des Devel Ther. 2020;14:823–832.
- Yuan Y-G, Gurunathan S. Combination of graphene oxide-silver nanoparticle nanocomposites and cisplatin enhances apoptosis and autophagy in human cervical cancer cells. Int J Nanomedicine. 2017;12:6537–6558.
- Ahmad R, Fu J, He N, et al. Advanced gold nanomaterials for photothermal therapy of cancer. J Nanosci Nanotechnol. 2016;16(1):67–80.
- Ghosh S, Sahoo N, Sajanlal P, et al. Anomalous subsurface thermal behavior in tissue mimics upon near infrared irradiation mediated photothermal therapy. J Biomed Nanotechnol. 2014;10(3):405–414.
- Gonçalves AS, Rodrigues CF, Moreira AF, et al. Strategies to improve the photothermal capacity of gold-based nanomedicines. Acta Biomater. 2020;116:105–137.
- Zhang G, Liu F, Jia E, et al. Folate-modified, cisplatin-loaded lipid carriers for cervical cancer chemotherapy. Drug Deliv. 2016;23(4):1393–1397.
- Xu L, Bai Q, Zhang X, et al. Folate-mediated chemotherapy and diagnostics: an updated review and outlook. J Control Release. 2017;252:73–82.
- Li L, Tao R, Song M, et al. Fabrication of self-assembled folate-biotin-quaternized starch nanoparticles as co-carrier of doxorubicin and siRNA. J Biomater Appl. 2017;32(5):587–597.
- Ma Z, Hu P, Guo C, et al. Folate-mediated and pH-responsive chidamide-bound micelles encapsulating photosensitizers for tumor-targeting photodynamic therapy. Int J Nanomedicine. 2019;14:5527–5540.
- Song J, Yang X, Jacobson O, et al. Sequential drug release and enhanced photothermal and photoacoustic effect of hybrid reduced graphene oxide-loaded ultrasmall gold nanorod vesicles for cancer therapy. ACS Nano. 2015;9(9):9199–9209.
- Song J, Pu L, Zhou J, et al. Biodegradable theranostic plasmonic vesicles of amphiphilic gold nanorods. ACS Nano. 2013;7(11):9947–9960.
- Cui Q, Xia B, Mitzscherling S, et al. Preparation of gold nanostars and their study in selective catalytic reactions. Colloids Surf, A. 2015;465:20–25.
- Wang Q, Min F, Zhu J. Preparation of gold nanowires and its application in glucose biosensing. Mater Lett. 2013;91:9–11.
- Chi T-T, Tu Y-C, Li M-J, et al. Photothermal optical coherence tomography based on the localized surface plasmon resonance of Au nanoring. Opt Express. 2014;22(10):11754–11769.
- Zhu F, Tan G, Zhong Y, et al. Smart nanoplatform for sequential drug release and enhanced chemo-thermal effect of dual drug loaded gold nanorod vesicles for cancer therapy. J Nanobiotechnol. 2019;17(1):15.
- Dembereldorj U, Choi SY, Ganbold EO, et al. Gold nanorod-assembled PEGylated graphene-oxide nanocomposites for photothermal cancer therapy . Photochem Photobiol. 2014;90(3):659–666.
- Zhang W, Guo Z, Huang D, et al. Synergistic effect of chemo-photothermal therapy using PEGylated graphene oxide. Biomaterials. 2011;32(33):8555–8561.
- Kim H, Namgung R, Singha K, et al. Graphene oxide-polyethylenimine nanoconstruct as a gene delivery vector and bioimaging tool. Bioconjug Chem. 2011;22(12):2558–2567.
- Zhu Y, Murali S, Cai W, et al. Graphene and graphene oxide: synthesis, properties, and applications. Adv Mater. 2010;22(35):3906–3924.
- Moon H, Kumar D, Kim H, et al. Amplified photoacoustic performance and enhanced photothermal stability of reduced graphene oxide coated gold nanorods for sensitive photoacoustic imaging. ACS Nano. 2015;9(3):2711–2719.
- Zhu X, Shi L, Schmidt MS, et al. Enhanced light-matter interactions in graphene-covered gold nanovoid arrays . Nano Lett. 2013;13(10):4690–4696.
- Sousa F, Cruz A, Fonte P, et al. A new paradigm for antiangiogenic therapy through controlled release of bevacizumab from PLGA nanoparticles. Sci Rep. 2017;7(1):13.
- Ugˇurlu N, Aşık MD, Çakmak HB, et al. Transscleral delivery of bevacizumab-loaded chitosan nanoparticles. J Biomed Nanotechnol. 2019;15(4):830–838.
- Battaglia L, Gallarate M, Peira E, et al. Bevacizumab loaded solid lipid nanoparticles prepared by the coacervation technique: preliminary in vitro studies. Nanotechnology. 2015;26(25):255102.
- Varshochian R, Jeddi-Tehrani M, Mahmoudi AR, et al. The protective effect of albumin on bevacizumab activity and stability in PLGA nanoparticles intended for retinal and choroidal neovascularization treatments. Eur J Pharm Sci. 2013;50(3-4):341–352.
- Hummers WS, Jr, Offeman RE. Preparation of graphitic oxide. J Am Chem Soc. 1958;80(6):1339–1339.
- Dixit N, Vaibhav K, Pandey RS, et al. Improved cisplatin delivery in cervical cancer cells by utilizing folate-grafted non-aggregated gelatin nanoparticles. Biomed & Pharmacother = Biomed & Pharmacother. 2015;69:1–10.
- Pradhan R, Mandal M, Mitra A, et al. Monitoring cellular activities of cancer cells using impedance sensing devices. Sens Actuators, B. 2014;193:478–483.,
- Pradhan R, Mandal M, Mitra A, et al. Assessing cytotoxic effect of ZD6474 on MDA-MB-468 cells using cell-based sensor. IEEE Sensors J. 2014;14(5):1476–1481.
- You J, Zhang G, Li C. Exceptionally high payload of doxorubicin in hollow gold nanospheres for near-infrared light-triggered drug release. ACS Nano. 2010;4(2):1033–1041.
- Zhang X-P, Sun J-G, Yao J, et al. Effect of nanoencapsulation using poly (lactide-co-glycolide) (PLGA) on anti-angiogenic activity of bevacizumab for ocular angiogenesis therapy. Biomed Pharmacother. 2018;107:1056–1063.
- Sakurai K, Akiyama H, Shimoda Y, et al. Effect of intravitreal injection of high-dose bevacizumab in monkey eyes. Invest Ophthalmol Vis Sci. 2009;50(10):4905–4916.
- Wang WY, Cao YX, Zhou X, et al. Delivery of folic acid-modified liposomal curcumin for targeted cervical carcinoma therapy. Drug Des Devel Ther. 2019;13:2205–2213.
- Erickson HP. Size and shape of protein molecules at the nanometer level determined by sedimentation, gel filtration, and electron microscopy. Biol Proced Online. 2009;11:32–51.
- Shah R, Eldridge D, Palombo E, et al. Lipid nanoparticles: Production, characterization and stability. 2015.
- Kaja S, Hilgenberg JD, Everett E, et al. Effects of dilution and prolonged storage with preservative in a polyethylene container on Bevacizumab (Avastin™) for topical delivery as a nasal spray in anti-hereditary hemorrhagic telangiectasia and related therapies. Hum Antibodies. 2011;20(3–4):95–101.