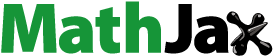
Abstract
Cadmium (Cd) is one of the most toxic heavy metal pollutants known to accumulate in the soil environment and pose a threat to public health. The tolerance of endophytic fungi isolated from Kosteletzkya virginica to cadmium was determined by the Oxford cup method. Trichoderma asperellum displayed the highest tolerance to cadmium on potato dextrose agar (PDA) medium and was selected subsequently for inoculating maize seedlings. Green fluorescein protein (GFP) labeled T. asperellum colonised in the roots of maize seedlings. Under Cd stress, the plant height, dry weight, fresh weight, root length, chlorophyll content and antioxidant enzyme activity of maize seedlings inoculated with T. asperellum were significantly higher than those of the control. T. asperellum also contributed to the up-regulated expression of some key genes in roots and leaves of maize seedlings, involving nitrogen absorption and metabolism to promote maize growth and Cd detoxification, accumulation, distribution, etc. for Cd transport to increase resistance to Cd.
Introduction
Heavy metals are a group of metallic chemical elements that have relatively high densities, atomic weights and atomic numbers. These heavy metals/metalloids include cadmium (Cd), mercury (Hg), lead (Pb), arsenic (As), zinc (Zn), copper (Cu), nickel (Ni) and chromium (Cr), which are significant environmental pollutants. The contents of heavy metals in soil have increased with the development of industrialisation and urbanisation during the past decades [Citation1]. As the most important heavy metal pollutant due to its high toxicity to living beings and long biological half-life in the human body and other organisms [Citation2, Citation3], Cd pollutants raise significant concerns throughout the world [Citation4]. In higher plants, oxidative stresses are often associated with Cd toxicity [Citation5–7]. In fact, Cd can decrease the content of glutathione and inhibition the activity of antioxidative, contributing to the accumulation of H2O2 [Citation8–11]. This change will cause lipid peroxidation, membrane damage and affect cell viability. Cd is unable to trigger Fenton-type reactions. It can indirectly activate the plasma membrane nicotinamide adenine dinucleotide phosphate (NADPH) oxidase, which generates superoxide radical anion (O2•−) using NADPH as the donor of electrons and induces generation of reactive oxygen species (ROS) [Citation12]. As a result, Cd will cause poor plant growth and low biomass accumulation [Citation13]. Cd has a long-term persistence in soil, and Cd pollution in urban soil is higher than that in agricultural soil, which poses a long-term threat to the environment [Citation14]. In order to alleviate the Cd content in soil and its pollution threat to human beings, animals and plants, it becomes more urgent to study Cd heavy metal.
At present, the mitigation measures of soil heavy metal pollution are mainly based on mechanical or physicochemical techniques, such as excavation and landfill, electrodialysis and soil cleaning [Citation15, Citation16]. However, there are limitations reported on these physicochemical approaches, such as high cost and low efficiency, when contaminants are present at low concentrations, and irreversible changes in soil physicochemical and biological properties, resulting in deterioration of soil ecosystems and introduction of secondary pollution. Some plants are used as remediators for heavy metals because of their ability to absorb high concentrations of heavy metals without affecting their normal development. Phytoremediation is the most feasible way to deal with pollution in heavily polluted areas. In addition, endophytic or rhizosphere microorganisms have been widely reported to play a supporting role in the mediation soil contaminated by heavy metals. By establishing symbiosis, plants recruit beneficial microbes through communications using signaling molecules. In this process, plants extend their roots into the soil matrix and establish a rhizosphere ecosystem to accumulate heavy metals and regulate their bioavailability, thus achieving remediation of contaminated soil. Microorganisms can also colonise plant tissues or directly stimulate root proliferation and promote plant growth, thus increasing the heavy metal tolerance and adaptability of host plants [Citation17]. Researchers found that associated with host plants endophytic strains live on plants, improve plant biomass and plant antioxidant systems, decrease lipid peroxidation products in response to stress [Citation17–19], increase tolerance to metals and use contaminants for remediation [Citation20, Citation21]. For example, plants inoculated with plant growth-promoting rhizobacteria (PGPR) containing 1-aminocyclopropane-1-carboxylic acid (ACC) deaminase showed increased biomass and got more attention [Citation22–24]. More and more plant-microorganism combined measures have been used to improve the performance of plant bioremediation in recent years. Moreover, microbial-assisted phytoremediation technology has the advantages of low cost, strong environmental adaptability and no secondary pollution during the treatment of heavy metal contaminated soil [Citation25]. However, many research studies have focused on bioremediation through bacterial strains. The role of endophytic fungi and arbuscular fungi has been neglected. In fact, certain interactions between fungi and plants at metal-contaminated sites can promote plant growth, mobilise heavy metals, promote seed germination and degrade organic pollutants [Citation26, Citation27].
In our previous studies, we isolated endophytic fungi from the roots of Kosteletzkya virginica and identified some different genera of endophytic fungi by morphological characteristics and molecular analysis. In order to screen Cd-tolerant endophytic fungi and evaluate their role in fungus-assisted phytoremediation, we investigated the resistance of different fungi to heavy metal Cd. Furthermore, we analysed the effect of Trichoderma asperellum, the most Cd resistant fungus, on antioxidant enzymes and the distribution of cadmium ions in different tissues of maize seedlings. Specifically, the expression of some genes related to cadmium ion transport, sequestration, detoxification and distribution in maize seedlings inoculated with T. asperellum was studied.
Little information is available on the effectiveness of maize seedlings inoculated with T. asperellum to increase Cd. In this study, we reported for the first time that T. asperellum induced increased activities of Superoxide dismutase (SOD), Peroxidase (POD) and Catalase (CAT) in maize seedlings under Cd stress, which may help to scavenge excess oxygen species, stabilise cell membranes and mitigate Cd-induced toxicity. After T. asperellum inoculation, maize seedlings significantly increased the accumulation of Cd in root and the immobilisation of Cd underground. Furthermore, our results revealed that ZmNADH:NR (Nicotinamide adenine dinucleotide: nitrate reductase) and ZmZIP (Zinc-regulated transporters, Iron-regulated transporter-like Proteins), performing a role in promoting nitrogen absorption and metabolism, Cd enrichment and distribution in different tissues, respectively, were contributors to improve plant growth and tolerance to Cd.
The combination of T. asperellum and maize has certain application prospects for phytoremediation. The results can provide a theoretical basis for soil improvement and for obtaining effective bioremediation tools to remove cadmium pollution.
Material and methods
Strain and plant material
The strains of T. asperellum, Ceriporia lacerate, Penicillium aurantiogriseum, Trichoderma viride, Alternaria tenuissima, Cladosporium sp., and Penicillium funiculosum isolated from Kosteletzkya virginica previously were used in this study. The seedlings of maize (Zea mays, inbred line Zheng 58; China) were cultivated in soil with a temperature of 28/22 °C and a photoperiod of 16 h/8 h (day/night).
Tolerance of fungi to Cd by the Oxford cup method
The tolerance to Cd was tested against T. asperellum, C. lacerate, P. aurantiogriseum, T. viride, A. tenuissima, Cladosporium sp., and P. funiculosum by the Oxford cup method. After potato dextrose agar (PDA) culture-medium was cooled down to 45 °C, 1 mL of the same concentration of sterile spore suspension (1 × 106 cfu/mL) was added in 99 mL PDA liquid medium and mixed. The mixture was poured into the upper layer of petri dishes with Oxford cup, then these cups were pulled out after solidification [Citation28]. Finally, different concentrations of CdCl2·2.5 H2O (0–0.35 mg/mL) were added in each hole which remained after Oxford cups were taken out of PDA culture-medium plates. The plates were then incubated at 28 °C for seven days, and the transparent area across the cup was considered as the tolerance to Cd.
Growth survey
Growth survey experiment was conducted using a 250 mL Erlenmeyer flask. Sterilised liquid PDA medium (50 mL) added with different concentrations of Cd was decanted in flasks, inoculated with 0.5 mL spore suspension (1 × 106 cfu/mL) (The concentration of Cd depended on the growth of different genus of strain) [Citation28]. The flasks for strains culturing were shaken in an orbital shaker at an agitation rate of 150 rpm at 28 °C for 7 days. Then, the fluid nutrient medium was filtered and the mycelia were washed three times with pure water and dried at 80 °C for 10 h until reaching constant weight. Finally, the dry weight of fungal biomass was measured.
Vector transformation and identification
Agrobacterium tumefaciens strain GV3101 containing pCAM-gfp vector was grown at 20 °C for 2 days in yeast extract broth (YEB) medium supplemented with kanamycin (20 µg/mL). The A. tumefaciens cells were diluted to OD600 = 0.15 in an induction medium (IM), either with 200 µM acetosyringone (IM + AS) or without AS (IM-AS). The cells were grown for an additional 6 h before mixing them with an equal volume of the conidial suspension from T. asperellum strain. The mixture (200 µL per plate) was plated on a nitrocellulose filter and placed on co-cultivation solid IM medium in the presence and absence of 200 µM AS. Following incubation at 28 °C for 2 days, the filter was transferred to PDA solid medium containing cefotaxime (200 µM) and hygromycin (250 µM) to screen positive transformants. Transformants labeled as GFP were identified using fluorescence microscopy and PCR analyses (with the forward primer: 5′-ATGGTGAGCAAGGGCGAGG-3′; the reverse primer: 5′-TTCTGCTGGTAGTGGTCGGC-3′). Screened transformants were used for colonisation experiments.
T. Asperellum transformant colonisation on the roots of maize seedlings
The surface-sterilised maize seeds were cultured on Murashige and Skoog (MS) solid medium for germination. T. asperellum was cultured on PDA solid medium at 28 °C for 7 days. Then spores were washed with pure water and diluted to 1 × 106 cfu/mL. Spore suspension was used to inoculate the roots of aseptic maize seedlings. Then, the roots of maize seedlings inoculated for 3 days were sectioned, and fluorescence was observed by laser scanning confocal microscope.
Determination of physiological index
T. asperellum was cultured for 5 days, centrifuged to collect the hyphae and resuspended in PBS. Healthy seedlings after germinating for 5 days were chosen and treated with resuspension of T. asperellum (5 mL/plant) [Citation29]. After culturing for 7 days, maize seedlings colonised with T. asperellum were treated with Cd of 100 mg/mL or 200 mg/mL for 15 days. Samples of leaves and roots were collected for analysis of phenotypic traits and activities of antioxidant enzymes were measured. The plant height, root length, dry weight, fresh weight and chlorophyll content were measured according to the method of Zhang [Citation30], and Cd contents of shoots and roots were measured by spectrophotometry [Citation31].
The activity of antioxidant enzymes
The activity of antioxidant enzymes of leaves and roots were measured. SOD was detected by p-nitro blue tetrazolium chloride photochemical reduction method, and 30 μL samples of enzyme extracts were incubated in 50 mM phosphate buffer (pH 7.8) containing 13 μM methionine, 63 μM NBT and 1.3 μM riboflavin. The reaction solution was then incubated for 10 min at a light intensity of 80 μmol m−2 s−1.
The SOD activity was spectrophotometrically detected at 560 nm with a spectrophotometer. The SOD activity was calculated using the following formula [Citation32]:
(1),
(1),
where A0 is the absorbance value of shading control group, As is the absorbance of sample, Vt is the volume of sample (mL), V1 is the volume of enzyme used in the measurement (mL) and W is the fresh weight of sample (g).
The CAT activity was determined using ultraviolet absorption spectrometry. 30 μL samples of enzyme extracts were incubated in 50 mM phosphate buffer (pH 7.0) containing 15 mM H2O2. The CAT activity was determined at 240 nm by measuring the consumption of H2O2 within 1 min and calculated as the amount of enzyme required to oxidise 1 μM H2O2 (extinction coefficient 0.0394 mM−1 cm−1) per minute. The CAT activity was calculated using the following formula [Citation32]:
(2),
(2),
where △A240 is the change in absorbance at 240 nm in the reaction solution, W is the fresh weight of the sample (g), Vt is the total volume of enzyme extracts (mL), Vs is the volume of enzyme used in the measurement (mL) and t is the reaction time (min).
The POD activity was detected with the guaiacol method, 30 μL samples of enzyme extracts were incubated in reaction buffer containing 1 mL of 50 mM phosphate buffer (pH 7.0), 1 mL of 0.3% H2O2 and 0.95 mL of 0.2% guaiacol. The POD activity was detected at 470 nm within 1 min and quantified by the amount of 1 μmol tetraguaiacol produced using its molar extinction coefficient (26.6 mM−1 cm−1) per minute. The POD activity was calculated using the following formula [Citation32]:
(3),
(3),
where △A470 is the change in absorbance at 470 nm in the reaction solution, W is the fresh weight of sample (g), Vt is the total volume of enzyme extracts (mL), Vs is the volume of enzyme used in the measurement (mL) and t is the reaction time (min).
Total RNA isolation
Frozen seedlings tissues were finely ground into powder in liquid nitrogen and total RNA extraction was performed using TRIzol reagent (Invitrogen, USA) according to the manufacturer’s instructions. The integrity and quality of the total RNA was checked by NanoDrop 1000 spectrophotometer and 2% agarose gel electrophoresis.
Reverse transcription quantitative PCR (RT-qPCR) analysis of transcript abundance
RT-qPCR was performed for all RNA samples to confirm the effects of T. asperellum to maize seedlings under Cd stress. First-strand cDNA synthesis was performed according to manufacturer’s instructions (HiFi-MMLV cDNA kit CW0744s). Reverse transcriptase quantitative RCR was performed using an Ultra SYBR Mixture ((LOW ROX) CW2601M). The generation of specific PCR products was confirmed by melting curve analysis. At a molecular level, some genes were induced by Cd or fungus [Citation33]. In order to identify some genes that mediate the detoxification, expressions of key genes involving detoxification, uptake and metabolism of nitrogen, Cd accumulation and distribution of different tissues of maize seedlings with T. asperellum colonisation in response of Cd stress were chosen for analysis. These genes contained enzyme-protective related genes ZmWRKY4 (WRKY-transcription factor 4), ZmSOD4 (superoxide dismutase 4) and ZmcAPX (ascorbate peroxidase), ZmZIP (Zinc-regulated transporters, Iron-regulated transporter-like Proteins), ZmPCS (phytochelatin synthase), ZmMTP (metal tolerance protein) involving divalent cation uptake, detoxification and transport respectively, nitrogen uptake and metabolic gene ZmNTR2.1, ZmNTR2.2 (high-affinity NO3- transporters), ZmNR1 (nitrate reductase 1), ZmNADH:NR and ZmMHA3 (plasma-membrane (H+)-ATPase3) which was related with transplasma membrane NO3- uptake, ZmMHA4 (proton-exporting ATPase4). Primer sequences of all the key genes above were designed according to reference [Citation33]. Housekeeping gene ZmACTIN (EU952376, forward primer: 5′-GCCATCCATGATCGGTATGG-3′ and reverse primer: 5′-GTCGCACTTCATGATGGAGTTG-3′) was used as reference gene for real-time RT-qPCR. Triplicates were performed on three independent experiments; analyses of real-time result were performed using Rotor-Gene (RG-3000).
Statistical analysis
Data were statistically analysed using SPSS® (version 22.0). The values are presented as mean ± standard deviation. P-values < 0.05 were considered significant and P-values < 0.01 were considered highly significant.
Results
Resistance of endophytic fungi isolated from Kosteletzkya virginica to Cd
Fungal tolerance to cadmium was examined including in T. asperellum, C. lacerate, P. aurantiogriseum, T. viride, A. tenuissima, Cladosporiumsp, and P. funiculosum, which are endophytic strains isolated from K. virginica with the Oxford cup method. The tolerance of different genera of fungi strains to Cd was investigated by the size of zone of inhibition (ZOI). According to the size of ZOI and the concentration of Cd, we observed that T. asperellum and Cladosporium sp. have the strongest tolerance to Cd, P. aurantiogriseum, C. lacerate, P. funiculosum are sensitive to Cd and A. tenuissima, T. viride are the most sensitive to Cd (). Growth inhibition rings began to appear when the Cd was 0.05 − 0.10 mg/mL, and the growth of hyphae was suppressed significantly.
Figure 1. Resistance of different genera of endophytic fungi to Cd. (A) The growth of seven endophytic fungi strains under different concentration of Cd stress. The concentration of Cd (mg/mL) in each cup hole is marked on the petri dish. (a–g) show respectively the growth of T. asperellum, T. viride, P. funiculosum, P. aurantiogriseum, Cladosporium sp., C. lacerate and A. tenuissima on PDA solid medium in Oxford cup and in PDA liquid medium containing different concentrations of Cd. (B) Growth curves of the mycelia of seven endophyte strains cultivated in PDA liquid medium supplemented with different concentrations of Cd (ranging from 0 to 0.3 mg/mL) for one week.
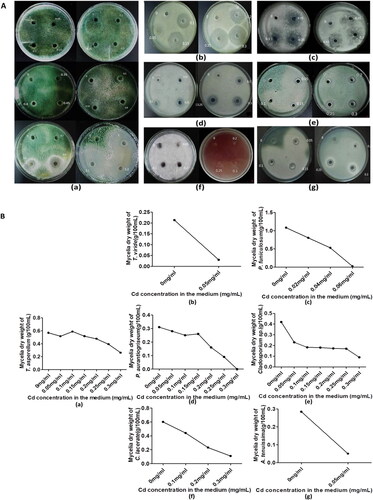
To further study the effect of Cd on these 7 genera of endophytic fungi, we measured their growth curves in different concentrations of Cd. The change trends in dry weight of mycelium are shown in . With the increase of Cd concentration, the dry weight of all mycelia genera showed a decreasing trend. P. funiculosum, P. aurantiogriseum and A. tenuissima are sensitive to Cd, which was consistent with the results of Oxford Cup experiment. Mycelia growth was completely inhibited when the concentration of Cd reached 0.05 mg/mL.
In contrast, T. asperellum was the most tolerant to Cd among the seven genera of fungi. The change rate of dry weight decreased gradually with the increased concentration of Cd. The growth of T. asperellum on the petri dish was not inhibited when the concentration of Cd was lower than 0.8 mg/mL. A clear zone of inhibition appeared when the concentration of Cd was 1 mg/mL. When the Cd concentration was 0. 3 mg/mL, the growth of T. asperellum was not significantly inhibited and the dry weight of mycelia was 0.262 g. In addition, it was noteworthy that the dry weight of mycelia of T. asperellum had increased slightly when the cadmium concentration was 0.10 mg/mL compared to the control. Therefore, we chose T. asperellum as the associated microorganism to inoculate maize seedlings for subsequent phytoremediation experiments under Cd contamination conditions.
Construction of GFP-labeled T. asperellum and colonisation of T. asperellum in the roots of maize seedlings
The present GFP-labeled T. asperellum line was generated by transforming the vector of pCAM-GFP into T. asperellum with the method of Agrobacterium-mediated transformation. After the recombinant plasmids were verified by PCR, the mycelium grown in selective PDA medium with resistance to kanamycin was placed on microscope slides and observed under confocal laser-scanning microscope (Germany, LSM780). As displayed in , transformants of T. asperellum were monitored to have bright-green color, while non-transformants without GFP have no fluorescence signal. As shows, transformant of T. asperellum had been successfully amplified and the non-transformants of T. asperellum had no gene fragments in the corresponding size.
Figure 2. Colonisation of T. asperellum within the roots of maize seedlings. (A) The fluorescence observation of GFP-labeled T. asperellum. (a) Non-transformed T. asperellum under bright field, (b) non-transformed T. asperellum under fluorescence field, (c) transformed T. asperellum under bright field, (d) fluorescence image of transformed T. asperellum under fluorescence field. (B) Amplification of GFP gene in transformant of GFP-labeled T. asperellum. Lane M represents the marker of DM 2000, Lane 0 represents the amplification product of GFP and Lane 1–3 represents PCR products of non-transformant and transformed T. asperellum, respectively. (C) Colonisation of GFP-labeled T. asperellum within roots of maize seedlings. (a) Roots in maize seedlings without colonisation with T. asperellum under bright field, (b) roots without colonisation of T. asperellum under fluorescence field, (c) roots in maize seedlings colonised with T. asperellum under bright field, (d) roots colonised with transformmant of T. asperellum under fluorescence field.
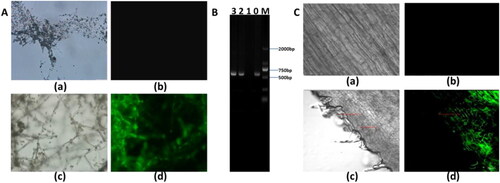
To determine the colonisation of T. asperellumin maize seedlings, roots of maize seedlings were inoculated with transformants T. asperellum for three days, and then root sections were sampled and observed by laser confocal scanning microscope. As shown in , compared to maize seedlings without inoculation, GFP fluorescence was found within the roots and spores, and mycelium could be clearly seen in bright green, which confirmed that T. asperellum successfully colonised the roots of maize seedlings within.
T. Asperellum improves the growth of maize seedlings under Cd stress
In order to verify the growth of maize seedlings inoculated with T. asperellum under Cd stress, we measured the physiological indices of maize seedlings after Cd treatment. The growth of maize seedlings in response to Cd is shown in . Cd induced a significant growth retardation in maize seedlings. The leaves became withered and had few lateral roots. This development inhibition was alleviated after inoculation with T. asperellum under the same Cd concentration compared to the control group (non-inoculated).
Figure 3. Effect of T. asperellum on the growth of maize seedlings under Cd stress. (A) The growth of maize seedlings inoculated with 5 mL of resuspension of T. asperellum with treatment of different concentration of Cd. (B) The plant height (a), root length (b), dry weight (c), fresh weight (d) and chlorophyll content (e) of maize seedlings inoculated with 5 mL of resuspension of T. asperellum with treatment of different concentration of Cd. p values were obtained using Student’s t test. p < 0.05 is indicated by ** and p < 0.001 is indicated by ***.
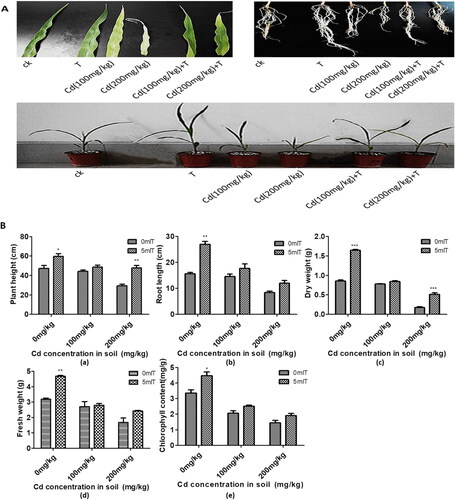
The growth response of seedlings inoculated with T. asperellum after exposure to different concentrations of Cd is displayed in . Colonisation of T. asperellum (watering the roots of maize seedlings with 5 mL resuspension of T. asperellum) increased plant height, roots length, fresh weight, dry weight and chlorophyll contents compared to that without inoculated seedlings under different concentration of Cd. These physiological indices above increased by 34.26%, 44.79%, 105.02%, 218.06% and 78.79%, respectively, when the concentration of Cd was 200 mg/kg.
Enhancement of the antioxidant enzyme activities in inoculated maize seedlings under Cd stress
Previous studies suggested that inoculation with PGPR had a positive effect on antioxidant enzyme activities of plant under abiotic stress resistance [Citation34]. As shows, the CAT, SOD and POD activities in roots and leaves of colonised plants were higher than that in non-inoculated seedlings under Cd-stress conditions. Antioxidant enzyme activities of SOD, POD and CAT increased 86.3%, 68.4% and 60.1% respectively, in the leaves of plants colonised with T. asperellum under Cd stress. An even more significant increase of all antioxidant enzyme activity was observed in the roots of maize seedlings after inoculation. These results indicated that T. asperellum promoted plant tolerance to Cd through accumulating antioxidant enzyme activities, which probably scavenged oxygen radicals produced under Cd stress.
Figure 4. The antioxidant enzyme activities in leaves and roots of maize seedlings inoculated with 5 mL of resuspension of T. asperellum with treatment of different concentration of Cd (0 mg/kg, 100 mg/kg and 200 mg/kg). Cd and Cd + T represent respectively treatment with Cd solution lonely and treatment with Cd solution after inoculation with T. asperellum. Plant treated with 0 mg/mL Cd solution was used as the control.
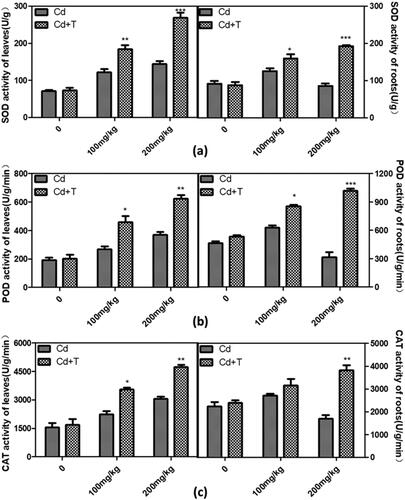
Effect of colonisation with T. asperellum on Cd accumulation within maize seedlings
After maize seedlings were cultivated for 15 days under Cd stress, the Cd accumulation in maize seedlings was measured. As shown in and , the Cd accumulation in aboveground sections of maize seedlings had no significant difference, while a significant difference in underground sections was observed compared to that of non-inoculated plant when the concentration of Cd was 100 mg/kg. When the concentration of Cd increased to 200 mg/kg, inoculation of T. asperellum promoted the Cd accumulation in roots (from 95.86 to 174.96 µg/kg) and decreased the Cd accumulation in shoots (from 16.87 to 12.11 µg/kg), respectively.
Table 1. Cd content in maize seedlings treated with 100 mg/kg Cd.
Table 2. Cd content in maize seedlings treated with 200 mg/kg Cd.
The transport coefficient is an index used to evaluate the ability of plants to transport and enrich heavy metals from underground to aboveground part. As shown in , the transport coefficient of maize seedlings decreased after colonisation with T. asperellum. This means that, if these maize seedlings inoculated with T. asperellum were used as phytoremediation tools, colonisation with T. asperellum could enrich Cd in plant seedling roots, immobilise Cd underground and decrease its bioavailability, thereby preventing Cd migration into the ecosystem and reducing the likelihood of Cd entering into the food chain.
Table 3. Cd transfer coefficient of maize.
Effect of T. asperellum colonisation in maize seedlings under Cd stress on the expression of stress-related genes and nitrogen metabolism-related genes
The expression of stress-related and nitrogen metabolism related genes changes to deal with the external environment [Citation35]. As shown in , after treatment with Cd, the expression of ZmZIP was down-regulated significantly in leaves and roots, while this gene was up-regulated after colonisation with T. asperellum. It can help plants accumulating more Cd-ions to decrease the hazards of Cd to soil. In the meantime, it is interesting that the expression of selected genes has increased in seedling roots in varying degrees. This suggests that, after inoculation with T. asperellum, most genes associated with heavy metal transport and metabolism were up-regulated, resulting in more absorption of Cd from soil, reducing the toxicity of heavy metals to plants.
Figure 5. Analysis of expression pattern of stress-related genes and nitrogen metabolism-related genes (y-axis) with different treatments (x-axis). (A) Expression of related genes in roots of maize seedlings inoculated with T. asperellum and with treatment of Cd. (B) Expression of related genes in leaves of maize seedlings inoculated with T. asperellum and with treatment of Cd. Warmer colors within the heat map indicate up-regulated and cooler colors are down-regulated.
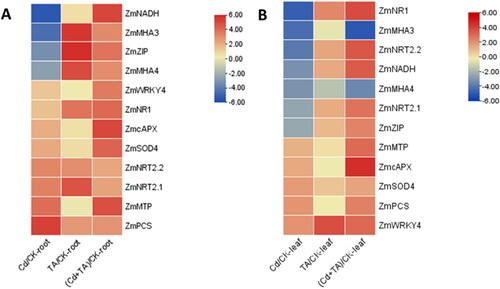
It is worth noting that the expression of ZmNRT2.1 and ZmNRT2.2, which was up-regulated in seedling roots and down-regulated in leaves under Cd stress, was both up-regulated in roots and leaves after colonising with T. asperellum. This means that the ability to transport NO3- in both roots and leaves was improved, therefore, T. asperellum could relieve the toxicity of Cd and promote the growth of maize seedlings through increasing the uptake and metabolism of nitrogen. In summary, colonisation of T. asperellum in maize seedlings could regulate the expression of related genes to reduce the damage of Cd to plants.
Discussion
Cadmium, released to the environment by several industrial and agricultural activities, is toxic for humans, animals and plants even at low concentrations. While plants treated with Cd show reduced growth compared to the control [Citation36], the mechanism of Cd toxicity is not yet completely understood [Citation37]. There have been no reports on the tolerance of T. asperellum to Cd and its application in fungi-assisted phytoremediation. Our experiment used modified Oxford cup method to investigate the tolerance of endophytic fungi to Cd and their potential for phytomediation. Based on the growth curve of T. asperellum under different concentrations of Cd, its tolerance to Cd was verified further. Thus, T. asperellum can be used as a biological adsorbent and remediator of cadmium pollution.
The gfp gene is the most important tool to study the interaction between target microorganisms and host due to its easy expression, good stability, lack of toxic effect on host cells or tissues and low consumption of bioenergy or other cofactors [Citation38]. Using gfp-tagged endophytes is the most effective research method to probe its colonisation [Citation39, Citation40]. Here, gfp-tagged T. asperellum was inoculated in the roots of maize seedlings and proliferated by extending the mycelium to the intercellular space within maize seedlings, which had been observed using confocal laser-scanning microscopes. T. asperellum, a biocontrol and plant growth promoting fungus, can enhance the resistance of maize to biological and Cd stresses, similar results were observed in salt tolerance in other reports [Citation41, Citation42].
It is well known that heavy metal pollution can induce the overproduction of ROS in plants. ROS can cause lipid peroxidation, membrane damage and inactivation of enzyme, thus affecting cell viability [Citation5, Citation43]. The antioxidant enzyme activity have directly role in scavenging harmful free radicals, which can help plants resist the adverse environment. SOD catalyses the efficient removal of superoxide radical. Expression of cytosolic SOD was reported to be stimulated upon Cd stress in higher plant [Citation44]. Recently, there are reports that increased POD activity under Cd stress lead to increased number and intensity of PODs in plant growth and development [Citation45]. In our study, the activity of POD was increased under Cd stress, implying that maize has the capacity to resist to Cd stress to a certain extent. The content of POD and CAT of maize seedlings increased significantly further after inoculation with T. asperellum compared to that of non-inoculated under the same concentration of Cd, which would help scavenging effectively oxygen radicals of maize seedlings produced by Cd stress, protecting cell membrane structure and enhancing plant resistance to Cd stress. Similar results were observed in the activity of SOD, which was also accumulated significantly after inoculation with T. asperellum under Cd stress, especially under high concentration of Cd. Therefore, T. asperellum-assisted phytomediation probably decreased ROS and promoted the growth of plants and resistance to Cd.
In addition, T. asperellum can regulate Cd accumulation within roots to prevent the Cd transport to aboveground section. Different from the essential trace elements such as Cu and Zn, the transport of toxic metal Cd in the xylem of maize is not completely determined by the water flow caused by transpiration tension, so the distribution of Cd to buds is reduced [Citation46]. In our study, the content of Cd was decreased in the aboveground section and increased in the underground section with inoculation with T asperellum. Colonisation of T. asperellum increased the accumulation of Cd and promoted the transfer of Cd from shoot to root.
Study on the expression of related gene of maize seedlings inoculated T. asperellum under Cd stress can help us to shed light on the molecular mechanism of promoting Cd enrichment in roots. It was reported that heavy metal accumulation and distribution in plants consists of several mechanisms, including: (1) metal uptake into roots, (2) xylem-loading and transport to the shoot and (3) phloem-mediated redistribution of metals from mature leaves to sink tissues, covering younger leaves, roots and seeds [Citation47]. The transport of Cd in maize seedlings was related to both key genes, ZmMTP encoding metal tolerance protein [Citation48, Citation49] and ZmZIP related to a resistance reaction of the plants. In general, it is believed that Cd2+ enters root cells via transporters for nutritional ions, for example, the ZmZIP family, and ZmMTP is one of the most important Cd vacuolar polyvalent chelate transporter proteins in plant roots and leaves. Our results showed that Cd stress significantly down-regulated ZmZIP and up-regulated ZmMTP in both root and leaves. On the contrary, the expression of ZmZIP and ZmMTP were both significantly up-regulated after colonisation with T. asperellum. This suggested that Cd accumulation was enhanced further after T. asperellum inoculation and that Cd was possibly enriched in mycellium of T. asperellum strain instead of in the maize seedling cells.
Furthermore, phytochelatin (PC) synthase is an enzyme that catalyses phytochelatin synthesis from glutathione. Some studies have shown that PC transport between tissues is bidirectional and that PC synthase has a strong effect on Cd distribution in the plant [Citation50, Citation51]. As shown in a previous report, the phloem is indeed responsible for Cd redistribution, likely as a PC–Cd complex [Citation52]. In the present study, ZmPCS expression was more significantly increased in roots compared to leaves under Cd stress as there is a significant up-regulation in leaves after colonisation with T. asperellum. This means that T. asperellum could induce the redistribution of Cd from leaves to roots, which is consistent with the result that Cd transfer coefficient in maize seedlings inoculated with T. asperellum decreases under 100 and 200 mg/kg concentrations of Cd.
Some studies have illustrated the mechanisms underlying Cd detoxification and the Cd tolerance-related genes that regulate the adaptive response of several plants have been identified [Citation30]. It is well known that ZmWRKY transcription factors act as positive regulators in abiotic stress responses by activation of the cellular antioxidant systems [Citation53, Citation54]. In our study, Cd induced up-regulation of ZmWRKY4 expression in roots and leaves, and the expression of ZmSOD4 and ZmcAPX were increased. These genes were also up-regulated after colonisation with T. asperellum. The synchronized expression patterns indicate that ZmWRKY4 might play a pivotal role in regulating both the ZmSOD4 and the ZmcAPX expression in response to Cd stress.
Another mechanism of Cd detoxification is related to phytochelatin, which are nitrogen- and sulphur-containing peptides synthesis linked to NO3- uptake and metabolism. Cd tolerance may involve a preferential accumulation of NO3- in Arabidopsis roots [Citation55, Citation56]. Here, we observed in maize that Cd can rapidly induce the mRNA levels of genes encoding NO3- transporters (ZmNRT2.1, ZmNRT2.2) in roots, as well as NO3- reductases (ZmNR1) in roots and leaves. After inoculation with T. asperellum, ZmNR1 transcript progressively accumulated in roots and leaves to increase the detoxification. NO3- uptake is energised by the plasma membrane (PM) H+-ATPase activity [Citation57]. Here, the expression of ZmMHA3and ZmMHA4 encoding PM proton pumps protein (PMH+- ATPases) was down-regulated in leaves and roots under Cd stress, which was consistent with the results of in a previous study [Citation58]. These ZmMHAs were up-regulated in roots after colonisation of T. asperellum to promote ATP hydrolysis rates to sustain the proton electrochemical gradient for speeding uptake the NO3-.
Some studies have shown the role of mycorrhiza in the increase of plant tolerance to Cd. It was shown in peas that inoculation with mycorrhizal fungi (Glomus intraradices) increased plant tolerance to Cd [Citation59]. This study suggests that colonisation with T. asperellum might help maize better adapt to Cd stress not only by regulating ZmNR, ZmSOD4 and ZmAPX expression for Cd detoxification but through transport of PC complexation and MTP for redistribution Cd in their roots.
Conclusion
In summary, inoculation with T. asperellum might suppress Cd transport from root to shoot, which is the key mechanism for maize seedlings to improve tolerance in response to Cd stress. These mechanisms may be due to the sequestration of Cd in mycelium of T. asperellum, contributing to blocking or reducing Cd uptake actively. The results of this study showed that Cd immobilisation in roots was highly enhanced by T. asperellum. In the future, maize inoculated with T. asperellum might be used in fungi-assisted phytoremediation program related to Cd-polluted soils.
Authors’ contributions
Yuqi Guo and ZengYuan Tian designed the research. Mengting He performed most of the experiments. All authors performed data analyses.
Research involving human and animal participants
This article does not contain any studies with human participants or animals performed by any of the authors.
Disclosure statement
The authors declare they have no conflict of interest.
Data availability statement
All data that support the findings reported in this study are available from the corresponding author upon reasonable request.
Additional information
Funding
References
- Han YL, Yuan HY, Huang SZ, et al. Cadmium tolerance and accumulation by two species of iris. Ecotoxicology. 2007;16(8):557–563.
- Günther K, Ji G, Kastenholz B. Characterization of high molecular weight cadmium species in contaminated vegetable food. Fresenius J Anal Chem. 2000;368(2–3):281–287.
- Branca JJV, Morucci G, Pacini A. Cadmium-induced neurotoxicity: still much ado. Neural Regen Res. 2018;13(11):1879–1882.
- Wei B, Yang L. A review of heavy metal contaminations in urban soils, urban road dusts and agricultural soils from China. Microchem J. 2010;94(2):99–107.
- Dixit V, Pandey V, Shyam R. Differential antioxidative responses to cadmium in roots and leaves of pea (Pisum sativum L. cv. Azad). J Exp Bot. 2001;52(358):1101–1109.
- León AM, José M, Palma FJC, et al. Antioxidative enzymes in cultivars of pepper plants with different sensitivity to cadmium. Plant Physiol Biochem. 2002;40(10):813–820.
- Boominathan R, Doran PM. Cadmium tolerance and antioxidative defenses in hairy roots of the cadmium hyperaccumulator, Thlaspi caerulescens. Biotechnol Bioeng. 2003;83(2):158–167.
- Paradiso A, Berardino R, de Pinto MC, et al. Increase in ascorbate-glutathione metabolism as local and precocious systemic responses induced by cadmium in durum wheat plants. Plant Cell Physiol. 2008;49(3):362–374.
- Buendia-Gonzalez L, Orozco-Villafuerte J, Cruz-Sosa F, et al. Prosopis laevigata a potential chromium (VI) and cadmium (II) hyperaccumulator desert plant. Bioresour Technol. 2010;101(15):5862–5867.
- Milone MT, Sgherri C, Clijsters H, et al. Antioxidative responses of wheat treated with realistic concentration of cadmium. Environ Exp Bot. 2003;50(3):265–276.
- Yang XE, Long XX, Ye HB, et al. Cadmium tolerance and hyperaccumulation in a new Zn-hyper accumulating plant species (Sedum alfredii Hance). Plant Soil. 2004;259(1/2):181–189.
- Rodriguez-Serrano M, Romero-Puertas MC, Zabalza A, et al. Cadmium effect on oxidative metabolism of pea (Pisum sativum L.) roots. Imaging of reactive oxygen species and nitric oxide accumulation in vivo. Plant Cell Environ. 2006;29(8):1532–1544.
- Liu L, Li J, Yue F, et al. Effects of arbuscular mycorrhizal inoculation and biochar amendment on maize growth, cadmium uptake and soil cadmium speciation in Cd-contaminated soil. Chemosphere. 2018;194:495–503.
- Fan L, Song J, Bai W, et al. Chelating capture and magnetic removal of non-magnetic heavy metal substances from soil. Sci Rep. 2016;6:21027.
- Bournonville B, Nzihou A, Sharrock P, et al. Stabilisation of heavy metal containing dusts by reaction with phosphoric acid: Study of the reactivity of fly ash. J Hazard Mater. 2004;116(1-2):65–74.
- Ward ML, Bitton G, Townsend T. Heavy metal binding capacity (HMBC) of municipal solid waste landfill leachates. Chemosphere. 2005;60(2):206–215.
- Fasani E, Manara A, Martini F, et al. The potential of genetic engineering of plants for the remediation of soils contaminated with heavy metals. Plant Cell Environ. 2018;41(5):1201–1232.
- Afridi MS, Mahmood T, Salam A, et al. Induction of tolerance to salinity in wheat genotypes by plant growth promoting endophytes: Involvement of ACC deaminase and antioxidant enzymes. Plant Physiol Biochem: PPB. 2019;139:569–577.
- Shahid M, Javed MT, Masood S, et al . Serratia sp. CP-13 augments the growth of cadmium (Cd)-stressed Linum usitatissimum L. by limited Cd uptake, enhanced nutrient acquisition and antioxidative potential. J Appl Microbiol. 2019;126(6):1708–1721..
- Hussain A, Kamran MA, Javed MT, et al. Individual and combinatorial application of Kocuria rhizophila and citric acid on phytoextraction of multi-metal contaminated soils by Glycine max L. Environ Exp Bot. 2019;159:23–33.
- Kanwal U, Ali S, Shakoor MB, et al. EDTA ameliorates phytoextraction of lead and plant growth by reducing morphological and biochemical injuries in Brassica napus L. under lead stress. Environ Sci Pollut Res Int. 2014;21(16):9899–9910.
- Ali NA, Bernal MP, Ater M. Tolerance and bioaccumulation of copper in Phragmites australis and Zea mays. Plant Soil. 2002;239(1):103–111.
- Chiu KK, Ye ZH, Wong MH . Enhanced uptake of As, Zn, and Cu by Vetiveria zizanioides and Zea mays using chelating agents. Chemosphere. 2005;60(10):1365–1375.
- Lin Q, Shen KL, Zhao HM, et al. Growth response of Zea mays L. in pyrene–copper co-contaminated soil and the fate of pollutants. J Hazard Mater. 2008;150(3):515–521.
- Zhang S, Lin H, Deng L, et al. Cadmium tolerance and accumulation characteristics of Siegesbeckia orientalis L. Ecol Eng. 2013;51:133–139.
- Read DJ, Ducket JG, Francis R, et al. Symbiotic fungal associations in ‘lower’ land plants. Philos Trans R Soc Lond B Biol Sci. 2000;355(1398):815–830.,
- Aly AH, Debbab A, Proksch P . Fungal endophytes: unique plant inhabitants with great promises. Appl Microbiol Biotechnol. 2011;90(6):1829–1845..
- Mohamed RM, Abo-Amer AE. Isolation and characterization of heavy-metal resistant microbes from roadside soil and phylloplane. J Basic Microbiol. 2012;52(1):53–65.. PMID: 22435113.
- García-Sánchez M, Palma JM, Ocampo JA, et al. Arbuscular mycorrhizal fungi alleviate oxidative stress induced by ADOR and enhance antioxidant responses of tomato plants. J Plant Physiol. 2014;171(6):421–428.
- Zhang J, Han W, Huang L, et al. Leaf chlorophyll content estimation of winter wheat based on visible and near-Infrared sensors. Sensors (Basel)). 2016;16(4):437.
- Cheng D, Tan M, Yu H, et al. Comparative analysis of Cd-responsive maize and rice transcriptomes highlights Cd co-modulated orthologs. BioMed Central. 2018;19:709..
- Wang H, Chen Z, Liu G, et al. Alterations of growth, antioxidant system and gene expression in Stylosanthes guianensis during Colletotrichum gloeosporioides infection. Plant Physiol Biochem. 2017;118:256–266.
- Rizzardo C, Tomasi N, Monte R, et al . Cadmium inhibits the induction of high-affinity nitrate uptake in maize (Zea mays L.) roots. Planta. 2012;236(6):1701–1712.
- Bhattacharyya PN, Jha DK. Plant growth-promoting rhizobacteria (PGPR): emergence in agriculture. World J Microbiol Biotechnol. 2012;28(4):1327–1350.
- Hashem A, Tabassum B, Fathi Abd Allah E. Bacillus subtilis: a plant-growth promoting rhizobacterium that also impacts biotic stress. Saudi J Biol Sci. 2019;26(6):1291–1297.
- Figlioli F, Sorrentino MC, Memoli V, et al. Overall plant responses to Cd and Pb metal stress in maize: growth pattern, ultrastructure, and photosynthetic activity. Environ Sci Pollut Res Int. 2019;26(2):1781–1790.
- Suzuki N, Koizumi N, Sano H. Screening of cadmium-responsive genes in Arabidopsis thaliana. Plant Cell Environ. 2001;24(11):1177–1188.
- Errampalli D, Leung K, Cassidy MB, et al. Applications of the green fluorescent protein as a molecular marker in environmental microorganisms. J Microbiol Methods. 1999;35(3):187–199.
- Shi Y, Li C, Yang H, et al. Colonization study of gfp-tagged Achromobacter marplatensis strain in sugar beet. J Microbiol. 2017;55:267–272.
- Xu JX, Li ZY, Lv X, et al. Isolation and characterization of Bacillus subtilis strain 1-L-29, an endophytic bacteria from Camellia oleifera with antimicrobial activity and efficient plant-root colonization. PLoS One. 2020;15(4):e0232096.
- Zhang Y, Tian C, Xiao J, et al. Soil inoculation of Trichoderma asperellum M45a regulates rhizosphere microbes and triggers watermelon resistance to Fusarium wilt. AMB Expr. 2020;10(1):189.
- Zhao L, Wang Y, Kong S. Effects of Trichoderma asperellum and its siderophores on endogenous auxin in Arabidopsis thaliana under iron-deficiency stress. Int Microbiol. 2020;23(4):501–509.
- Singh S, Eapen S, D’Souza SF . Cadmium accumulation and its influence on lipid peroxidation and antioxidative system in an aquatic plant, Bacopa monnieri L. Chemosphere. 2006;62(2):233–246.
- Ferreira RR, Fornazier RF, Vitória AP, et al. Changes in antioxidant enzyme activities in soybean under cadmium stress. J Plant Nutr. 2002;25(2):327–342.
- Kochhar S, Kochhar VK. Expression of antioxidant enzymes and heat shock proteins in relation to combined stress of cadmium and heat in Vigna mungo seedlings. Plant Sci. 2005;168(4):921–929.
- Nguyen C, Soulier AJ, Masson P, et al. Accumulation of cd, Cu and Zn in shoots of maize (Zea mays L.) exposed to 0.8 or 20 nM Cd during vegetative growth and the relation with xylem sap composition. Environ Sci Pollut Res Int. 2016;23(4):3152–3164.
- Mendoza-Cózatl DG, Jobe TO, Hauser F, et al. Long-distance transport, vacuolar sequestration, tolerance, and transcriptional responses induced by cadmium and arsenic. Curr Opin Plant Biol. 2011;14(5):554–562.
- Wang JL, Li T, Liu GY, et al. Unraveling the role of dark septate endophyte (DSE) colonizing maize (zea mays) under cadmium stress: physiological, cytological and genic aspects. Sci Rep. 2016;6:22028.
- Migocka M, Kosieradzka A, Papierniak A, et al. Two metal-tolerance proteins, MTP1 and MTP4, are involved in Zn homeostasis and Cd sequestration in cucumber cells. J Exp Bot. 2015;66(3):1001–1015.
- Gong JM, Lee DA, Schroeder JI. Long-distance root-to-shoot transport of phytochelatins and cadmium in Arabidopsis. Proc Natl Acad Sci U S A. 2003;100(17):10118–10123.
- Chen A, Komives EA, Schroeder JI. An improved grafting technique for mature arabidopsis plants demonstrates long-distance shoot-to-root transport of phytochelatins in arabidopsis. Plant Physiol. 2006;141(1):108–120.
- Shukla D, Kesari R, Mishra S, et al. Expression of phytochelatin synthase from aquatic macrophyte Ceratophyllum demersum L. enhances cadmium and arsenic accumulation in tobacco. Plant Cell Rep. 2012;31(9):1687–1699.
- Agarwal P, Reddy MP, Chikara J. WRKY: its structure, evolutionary relationship, DNA-binding selectivity, role in stress tolerance and development of plants. Mol Biol Rep. 2011;38(6):3883–3896.
- Hong C, Cheng D, Zhang G, et al. The role of ZmWRKY4 in regulating maize antioxidant defense under cadmium stress. Biochem Biophys Res Commun. 2017;482(4):1504–1510.
- Wang T, Hua Y, Chen M, et al. Mechanism enhancing Arabidopsis resistance to cadmium: the role of NRT1.5 and proton pump. Front Plant Sci. 2018;9:1892.
- Astolfi S, Zuchi S, Passera C . Role of sulphur availability on cadmium-induced changes of nitrogen and sulphur metabolism in maize (Zea mays L.) leaves. J Plant Physiol. 2004;161(7):795–802.
- McClure PR, Kochian LV, Spanswick RM, et al . Evidence for cotransport of nitrate and protons in maize roots: I. Effects of nitrate on the membrane potential. Plant Physiol. 1990;93(1):281–289.
- Nocito FF, Espen L, Crema B, et al. Cadmium induces acidosis in maize root cells. New Phytol. 2008;179(3):700–711.
- Rivera-Becerril F, Calantzis C, Turnau K, et al. Cadmium accumulation and buffering of cadmium-induced stress by arbuscular mycorrhiza in three Pisum sativum L. genotypes. J Exp Bot. 2002;53(371):1177–1185.