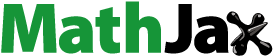
Abstract
To investigate the effects of different dominant species on the microbial functional diversity of vegetation concrete substrates, the rhizosphere and non-rhizosphere soils of three dominant species (Pennisetum alopecuroides (PA), Arthraxon hispidus (AH) and Pueraria lobata (PL)) of vegetation concrete were studied using the Biolog-Eco method at Xiangjiaba Hydropower Station. The results showed that the microbial activity and microbial functional diversity indices in the rhizosphere soil of the three dominant plants were significantly higher than those in the non-rhizosphere soil and that the microbial activity and functional diversity indices in the rhizosphere and non-rhizosphere soil of AH, as well as in the rhizosphere soil of PL, were at a high level. The carbon sources predominantly used by rhizosphere soil microbes were carbohydrates, amino acids and polymers, while the carbon sources predominantly used by non-rhizosphere soil microbes were carboxylic acids, amino acids and polymers. The governing elements of the carbon source metabolic features of the microbial community were the soil moisture content (WC), organic matter (OM), total nitrogen (TN), potential of hydrogen (pH), microbial carbon (MBC), microbial nitrogen (MBN) and microbial phosphorus (MBP). Based on a thorough examination of these aspects, it was concluded that vegetation had altered the microbial community structure and functional diversity of the vegetation concrete substrate, perhaps improving the overall metabolic activity. A. hispidus with high adaptability should be prioritized in the regeneration of vegetation on concrete slopes.
Introduction
Soil microorganisms are an essential component of the soil ecosystem, and they play a significant role in the material and energy cycles [Citation1–3]. Microorganisms generate a unique inorganic-organic-biological complex in the soil with a mix of minerals and organic matter, which promotes the circulation of soil nutrient elements and has a natural role in filtering and purifying the ecological environment (Pronk et al., 2017). Thus, soil microorganisms are an important factor in maintaining the operation of the terrestrial ecosystems (Shao 2006) [Citation4]. The functional diversity of the soil microbial community is one of the most significant markers for expressing the condition and function of the soil microbial community, referring to the degree of diversity of activities such as microbial decomposition, nutrient transfer and stimulation or inhibition of plant development in the soil ecosystem. The microbial functional diversity in soil is critical for clarifying the role of the microbial community in various environments and evaluating soil fertility [Citation5, Citation6].
Soil micro-domain structure and spatial division are direct variables influencing soil micro-biodiversity, and plant rhizosphere is one of the major forms of soil micro-domain structure [Citation7]. The rhizosphere is the interface between soil, plants and microorganisms, as well as the site of material and energy circulation [Citation8]. To support plant development, the root system collects nutrients from the soil. At the same time, vegetation has a substantial impact on the community features of microorganisms through surface degradation breakdown and root system secretion of organic waste [Citation9, Citation10]. Many academics have conducted significant studies on plant rhizosphere microorganisms in natural ecological systems such as salty land, hilly areas, grassland and dry zones [Citation11–14], as well as non-natural ecological systems such as farmland and polluted land, which are influenced by humans [Citation15–17]. However, there are few publications on the microbial functional variety of artificial configuration substrate during the ecological restoration process.
As a significant cascade hydropower station in the Jinsha River basin, Xiangjiaba Hydropower Station occupies a vast area of land, and its construction has destroyed a considerable quantity of surface vegetation, posing a danger to the long-term growth of ecosystems in the region. The Vegetation Concrete Base Spraying technique (CBS) [Citation18] has been widely used in this area to solve the problem of the inability of exposed slopes to grow vegetation or the slow rate of vegetation reconstruction by spraying a layer of natural soil on the slope and providing the habitat material required for plant growth [Citation19, Citation20]. In the course of ecological restoration, we should consider not only the reconstruction of plants but also the activity and variety of soil microbes, which are critical to soil reunification and the transformation of soil nutrients [Citation21, Citation22]. The presence of vegetation not only supplies material and energy for soil microorganism reproduction and growth, but it also affects the activity and variety of soil microorganisms by influencing the physical and chemical characteristics of soil [Citation23]. As a result, investigating the link between plant, soil, and microorganisms is beneficial to understanding the improvement of vegetation to the soil environment.
The aim of this study was to compare the rhizosphere and non-rhizosphere soils of three dominating plants in the vegetation concrete slope restoration at Xiangjiaba. The Biolog-Eco approach was used to investigate the link between the average color change rate, microbial diversity index, microbial carbon source consumption type, and soil physical and chemical parameters of distinct plant rhizosphere and non-rhizosphere soils. The impacts of the rhizosphere of different dominating species on the soil microbial functional diversity were studied to give a theoretical foundation for hydropower station slope rehabilitation.
Materials and methods
Site description
The study was conducted at the Xiangjiaba Hydropower Station (28°22’15"–28°39’16"N, 104°3’27"–104°25’25"E), which is located at the outflow of Xiangjiaba Valley in the lower parts of the Jinsha River mainstream and the triangle created by the Jinsha and Hengjiang rivers. The research area has a hot, dry tropical monsoon climate in the South Subtropical Zone. It is typical of a dry-hot valley with minimal precipitation and distinct dry and wet seasons. Meteorological statistics indicate that the annual average temperature is 15.0°C∼18.1 °C, the lowest temperature is −5.9 °C, and the highest temperature is 39 °C, the annual average relative humidity is about 82%, the annual rainfall is 850-1500 mm, and more than 90% of the rainfall falls between June and November.
The research was carried out on the vegetation concrete slope in the perturbation area of the Xiangjiaba hydroelectric power station, which was finished at the end of 2006. The gradient is approximately 53 degrees, and the altitude is 328.5 meters. The substrate is a mix of excavated raw soil from the area, cement, organic matter, compound fertilizer, concrete greening additives and water preservation agents. The thickness of the slope substrate is 10 cm, the original pH of the base material is 8.43, and the total organic carbon content is 86.35 g/kg. The plants in this area are mainly Pennisetum alopecuroides, Arthraxon hispidus, Pueraria lobata, Vitex negundo, Broussonetia papyrifera, Indigofera amblyantha among others.
Soil sample collection
The rhizosphere and non-rhizosphere soil of three plants (Pennisetum alopecuroides (PA), Arthraxon hispidus (AH) and Pueraria lobata (PL)) were chosen based on the results of a vegetation survey. In this study, R stands for rhizosphere soil and S stands for non-rhizosphere soil. Thus, PA-R, PA-S, AH-R, AH-S, PL-R, PL-R, and PL-S represent respectively the rhizosphere and non-rhizosphere soil of the three plants.
In July 2016, soil samples were obtained using the shaking-off method of Riley and Barber [Citation24]. We randomly chose 8-10 plants from three dominating species and dug the soil with entire roots in three quadrats (20 × 20 m) (the volume depends on the range of roots). To begin, a significant amount of soil with no roots was gently shaken off and combined into a plastic bag to be considered non-rhizosphere dirt. The soil connected to the root surface was then forcefully shaken down to get the rhizosphere soil. The soil was separated into two portions after the stones, plant remnants and artificial pollutants were removed. One half was kept in a refrigerator at 4 °C for Biolog analysis, while the other was allowed to dry naturally and was sieved through a 100-mesh sieve to determine the soil physical and chemical parameters. shows the soil physical and chemical parameters associated with different plants, whereas shows the soil microbial biomass.
Table 1. Physical and chemical properties of soil.
Table 2. Microbial biomass of soil.
Determination method and data analysis
The soil potential of hydrogen (pH), moisture content (WC) and organic matter (OM) were determined according to Zhang [Citation25]. The pH was measured using a glass electrode in a 1:2.5 soil/water solution, and WC was assessed in samples oven-dried at 105 °C. OM was measured by the potassium dichromate method. Total nitrogen (TN), total phosphorus (TP), total potassium (TK), available phosphorus (AP), available nitrogen (AN) and available potassium (AK) were determined spectrophotometrically using the modified Berthelot reaction and a Skalar autoanalyzer (Skalar Analytical B.V., Breda, The Netherlands) [Citation26]. Microbial biomass carbon (MBC), microbial biomass nitrogen (MBN), and microbial biomass phosphorus (MBP) were measured using the fumigation method [Citation27–29].
The microbial activity and functional diversity of soil microorganisms were determined by the Biolog-ECO method [Citation30]. The new soil sample (5 g dry soil) was placed in a 45-mL sterile triangular bottle, and the bottle opening was sealed with tin foil paper. For 20 min, the triangular bottle was put on the shaking table, and the rotation speed was set to 190 r/min while standing for 10 min. On the ultra-clean workbench, the supernatant was diluted to 10−3. Using an electronic liquid shifter, the diluted bacterial solution was added to the Biolog ecological culture plate, 150 L per hole. The inoculated ecological plate was cultivated in a constant temperature incubator with the temperature set to 25 °C for 10 days, and the readings on the Biolog reader were read every 24 h.
The average well color development (AWCD) of the solution absorbance value in micro-plate wells [Citation31] was used to describe the microbial functional diversity, and the Shannon–Wiener Index (H), Evenness Index (E), Richness Index (S), Simpson’s diversity Index (D) and McIntosh Index (U) were used to determine the soil microbial community functional diversity index.
(1)
(1)
(2)
(2)
(3)
(3)
(4)
(4)
(5)
(5)
In the formula, is the optical density value of each well,
is the optical density of the control well.
is the number of the well.
is the proportion of the total difference between the optical density of the culture well and the control well.
is the richness index, which refers to the total number of carbon sources used and is the number of wells with
greater than 0.
is the relative absorbance value of each well and
is the sum of the relative absorbance values.
All results are reported as mean values with standard error (±SE) of repeated experiments. To find variations in soil physicochemical features, we employed one-way analysis of variance (ANOVA) with a significance level of p < 0.05. ANOVA and Pearson correlation analyses were carried out using SPSS 22.0. Conoco 4.5 was used to do a principal component analysis (PCA) and redundancy analysis (RAD) on 120 h of Ecoplate data to examine the influence of different engineering eco-restoration strategies on soil microbial populations.
Results
Characteristics of metabolic activity of microorganisms in rhizosphere and non-rhizosphere soil of different plants using all carbon sources
depicts the AWCD of continuous culture for 10 days every 24 h. The general shifting trend of AWCD of soil microorganisms was constant across all plots, progressively increasing with the incubation time, although the increased range of each incubation period was varied. The growth rate of AWCD did not vary considerably before 24 h but increased dramatically from 24 h to 120 h. At this time, the microbial metabolic activity was at its peak, and the capacity to utilize carbon sources was at its peak. After 120 h, the rate of AWCD rise decreased and tended to stay stable. The AWCD of AH-R, AH-S, and PL-R remained high throughout the culture process, followed by PA-R, PA-S, and PL-S, which stayed low throughout. At 120 h the AWCD of rhizosphere and non-rhizosphere soil of various plants ranged from 0.36 to 0.86. The AWCD variant was AH-R > PL-R > AH-S > PA-R > PA-S > PL-S. Except for PL-R and AH-S, PA-R and PA-S, PA-S and PL-S, the differences between the remaining soils were significant (p < 0.05) according to the significance analysis. Rhizosphere soils had greater microbial metabolic activity than non-rhizosphere soils.
Figure 1. The AWCD of soil microbial communities in rhizosphere and non-rhizosphere of different plants.
Note: PA-R, rhizosphere soil of Pennisetum alopecuroides; PA-S, non-rhizosphere soil of Pennisetum alopecuroides; AH-R, rhizosphere soil of Arthraxon hispidus; AH-S, rhizosphere soil of Arthraxon hispidus; PL-R, rhizosphere soil of Pueraria lobata; PL-S, rhizosphere soil of Pueraria lobata.
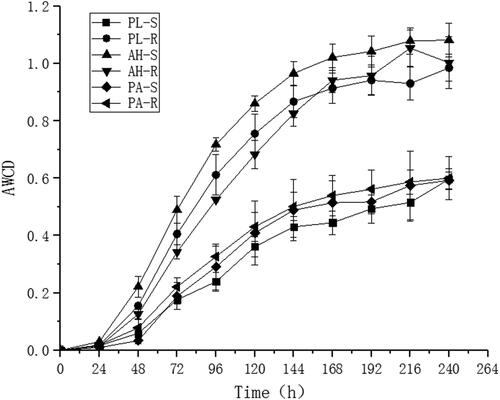
Diversity indices for microbial community in rhizosphere and non-rhizosphere soil of different plants
To further clarify the differences between rhizosphere and non-rhizosphere soil microbial communities of different plants, the Shannon–Wiener Index (H), Evenness Index (E), Richness Index (S), Simpson’s diversity Index (D) and McIntosh Index (U) were calculated by analyzing the functional diversity of soil microbial communities after 120 h of culture. As can be seen from , the various ranges of S and H, which represent the richness and diversity of microbial communities, were 24.67–30.00 and 2.59–3.24, respectively. There were some discrepancies between the rhizosphere and non-rhizosphere soils of three plants. H revealed that AH-R and PL-R were more than PA-R (p < 0.05), and AH-S was greater than PL-S and PA-S (p < 0.05). S showed that PL-R and PA-R outperformed AH-R (p < 0.05), while PL-S outperformed AH-S and PA-S (p < 0.05). E expressing community evenness and U reflecting carbon source utilization types have variance ranges of 0.73–0.94 and 3.01–5.53, respectively. E demonstrated that AH-R and PL-R were greater than PA-R (P 0.05), U indicated that AH-R and PL-R were greater than PA-R (p < 0.05), and AH-S was greater than PL-S and PA-S (p < 0.05). The fluctuation range of D, which represented the most prevalent species of the microbial community, was 0.92-0.97, with no significant difference across plots. The functional diversity indices of microbial communities in rhizosphere and non-rhizosphere soils under three distinct plant types were substantially different (p < 0.05). Except for S, the indices of rhizosphere soil in PL were greater than those of non-rhizosphere soil. The rhizosphere soil indices in AH were greater than the non-rhizosphere soil indices, but only S and U in PA were higher than the rhizosphere soil indices. Different vegetations had minimal influence on the common microbial groups of the vegetation concrete substrate but had large indigenous effects on the uniformity of specific species and communities. In summary, the functional diversity indices of microbial communities in rhizosphere and non-rhizosphere soils of the three vegetations were considerably different, and the considerable indigenous roots of plants influenced the functional diversity of soil microbial communities.
Table 3. Diversity indices for soil microbial communities.
Utilization of different carbon sources by microbial communities in rhizosphere and non-rhizosphere soil of different plants
The Biolog EcoPlate contains 31 carbon sources of 6 types: 10 carbohydrates, 7 carboxylic acids, 6 amino acids, 4 polymers, 2 phenolic compounds and 2 amines according to the properties of chemical groups. The utilization characteristics of microbial communities on 31 carbon sources were affected by the characteristics of the microbial communities. The AWCD of diverse carbon sources is calculated by averaging the light absorption values of each carbon source. In this study, the ability of rhizosphere and non-rhizosphere soil microorganisms of three plants to metabolize six types of carbon sources was shown to be substantially different (p < 0.05) (). The utilization rates of carbohydrates, amino acids and amines were 0.27–0.79, 0.19–0.92 and 0.13–0.54, respectively. In both rhizosphere and non-rhizosphere soils, AH was the highest, followed by PL and the lowest PA. Carboxylic acid, phenolic acid, and polymers were used in amounts ranging from 0.03–0.52, 0–0.8, and 0.04–0.64, respectively. In rhizosphere soil, AH was the greatest, followed by PL, while PA was the lowest. Different criteria were applied to these three types of carbon sources in non-rhizosphere soil. The capacity of the rhizosphere soil microbial community of PL to metabolize six types of carbon sources was greater than that of the non-rhizosphere soil microbial community among the three different vegetations. Aside from phenolic acid, the capacity of the rhizosphere soil microbial community of AH to metabolize various forms of carbon sources was shown to be greater than that of non-rhizosphere soil. The capacity of the PA rhizosphere soil microbial community to metabolize carbohydrates and amino acids was greater than that of the non-rhizosphere soil microbial community, but the ability of the rhizosphere soil microbial community of PA to metabolize the other four carbon sources was the opposite.
Table 4. Utilization of different carbon sources by microbial communities.
Carbohydrates, amino acids, phenolic acids, and polymers were the preferred carbon sources for AH-R and AH-S bacteria in terms of consumption. Carbon sources chosen by PL-R and PL-S microbes were carbohydrates and amino acids, but carbon sources utilized by PA-R and PA-S microorganisms differed significantly. Carbon sources chosen by PA-R bacteria were carbohydrates and polymers, whereas carboxylic acids, amino acids and polymers were preferred by PA-S microorganisms. The findings revealed that plant roots were useful in improving the ability of the soil microbial community to consume carbon sources in vegetation concrete substrate, and the impacts of different vegetation on carbon source utilization revealed diverse rules.
Principal component analysis of metabolism function of the microbial community in rhizosphere and non-rhizosphere soil of different plants
The PCA analysis of the microbial consumption of the 31 carbon sources retrieved four major components with a total contribution rate of 93.6% (). The variance contribution rates of the first principal component (PC1), second principal component (PC2), third principal component (PC3) and fourth principal component (PC4), respectively, were 56.6%, 19.7%, 9.5% and 7.8%. The first principal component (PC1) and second principal component (PC2) explained 76.4% of the overall variation, and the variance contribution rate of the principal component was low. Therefore, only the first two main components were retrieved for analysis in this study. Six soil samples were arranged in four quadrants, and the distance between each sample showed the ability of the carbon source to be utilized. The correlation analysis between the principal component and AWCD revealed that PC1 was highly positively linked with AWCD (97.2%) but not PC2 (23.6%). As a result, PC1 may better reflect the total activity of the microbial community. The main component analysis score of soil microbial carbon source consumption on PC1 was AH-R > PL-R > AH-S > PA-R > PA-S > PL-S, which corresponded to the order of AWCD values after 120 h of soil microbial culture. The relationship between PC1 and the AWCD value of the carbon source was investigated. The correlation study between PC1 and AWCD revealed that there were 9 types of carbon sources that were positively connected with a correlation coefficient larger than 0.88, including 3 types of amino acids and carboxylic acids, 2 types of amines, and 1 kind of carbohydrate (). As a result, the variations in microorganisms between rhizosphere and non-rhizosphere soils of the three plants are mostly due to the use of amino acid and carboxylic acid carbon sources.
Figure 2. PCA analysis of carbon source utilization in soil microbial community.
Note: PA-R, rhizosphere soil of Pennisetum alopecuroides; PA-S, non-rhizosphere soil of Pennisetum alopecuroides; AH-R, rhizosphere soil of Arthraxon hispidus; AH-S, rhizosphere soil of Arthraxon hispidus; PL-R, rhizosphere soil of Pueraria lobata; PL-S, rhizosphere soil of Pueraria lobata.
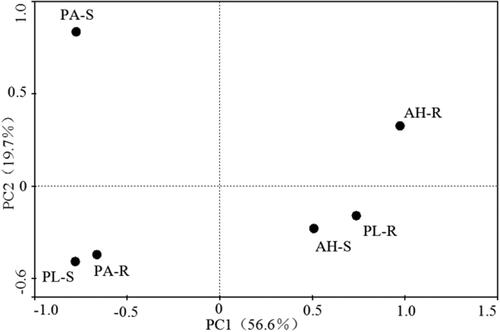
Table 5. Major carbon sources significantly associated with PC1 in soil.
Relationship between functional diversity of the microbial community and soil factors in rhizosphere and non-rhizosphere soil of different plants
The link between the soil microbial metabolic diversity index and soil physicochemical parameters was investigated further to highlight the inherent relationship between functional diversity of microbial communities and soil environmental variables. The data in revealed a strong positive connection between H and OM, pH (p < 0.05). E was inversely associated with TN (p < 0.05). S was shown to be positively connected with AP and AK, whereas D was positively correlated with OM, pH (p < 0.01) and AN (p < 0.05). Furthermore, U was shown to be positively linked with OM (p < 0.01) and MBC, MBP (p < 0.05).
Table 6. Soil related factor matrix.
The RDA ordination biplot () depicts the relationship between environmental factors and microbial utilization of the 31 carbon sources. The first, second, third and fourth axes have eigenvalues of 0.548, 0.154, 0.126 and 0.101, respectively. The total explained variation of carbon source metabolism characteristics and soil physical-chemical properties of soil microbial communities on the four axes reached 92.9%, indicating that soil physical-chemical properties were closely related to carbon source metabolism of soil microbial communities and had significant effects. In the first and second axis, the cumulative explained variance was 70.2%. The first axis was mostly associated with OM, pH, MBC, MBN and MBP, whereas the second axis was primarily associated with WC and TN. It is possible that the carbon source metabolism in microbial communities was controlled by WC, OM, TN, pH, MBC, MBN and MBP.
Figure 3. RDA analysis of carbon source metabolism and soil properties in soil microbial community.
Note: OM, organic matter; TN, total nitrogen; AN, available nitrogen; TP, total phosphorus; AP, available phosphorus; TK, total potassium; AK, available potassium; pH, potential of hydrogen; WC, moisture content; MBC, microbial biomass carbon; MBN, microbial biomass nitrogen; MBP, microbial biomass phosphorus; PA-R, rhizosphere soil of Pennisetum alopecuroides; PA-S, non-rhizosphere soil of Pennisetum alopecuroides; AH-R, rhizosphere soil of Arthraxon hispidus; AH-S, rhizosphere soil of Arthraxon hispidus; PL-R, rhizosphere soil of Pueraria lobata; PL-S, rhizosphere soil of Pueraria lobata.
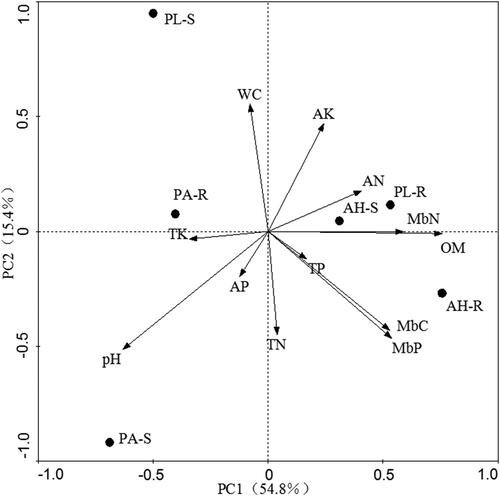
Discussion
The results of this experiment showed that the metabolic activity of soil microorganisms using carbon sources in various vegetations increased with incubation time, which was consistent with previous study findings [Citation32–34]. During incubation, the microbial activity showed some differences among the rhizosphere soil of the three plants. The organization and function of the rhizosphere soil microbial community varied between vegetation types and genotypes of the same plant [Citation35, Citation36]. In this study, AH had the highest soil microbial activity in the three rhizosphere soils, followed by PL, and PA had the lowest. PL is a leguminous plant; hence this was the key cause for this. Rhizobium symbiotic with leguminous plants can significantly enhance the rhizosphere soil nutrient content, microbial population and microbial community structure, consequently increasing the soil microbial activity [Citation37, Citation38]. Because AH may progressively adjust to habitat stress by self-regulation, it exhibits high resilience and adaptability even in thin soil layers [Citation39, Citation40]. The thickness of the slope soil layer restored by vegetation concrete is 10 cm, and AH has superior adaptation in this environment, which may explain why AH is a gramineous plant with higher rhizosphere soil microbial activity than PL and PA. There were also some differences in microbial activity among the non-rhizosphere soil of the three plants, but they showed a different trend compared to the differences among the microbial activity in the rhizosphere soil of the three plants. According to the microbial activity, the non-rhizosphere soil of the three plants ranked as follows: AH > PA > PL. The primary cause of this phenomenon was that non-rhizosphere soil was less impacted by plant roots and more affected by litterfall [Citation11, Citation41]. Litter breakdown could considerably increase the organic carbon and nitrogen content of the soil, and carbon and nitrogen are critical elements for microbial metabolism [Citation9, Citation42]. Because of the vertical and horizontal intersection of plant roots in the soil, microbial activity in the rhizosphere soil of the three plants was much higher than that in the non-rhizosphere soil. The content of soil nutrients around the root system was considerably enhanced by secretion, root hairs and root epidermal cells [Citation43]. Simultaneously, root exudates and nutrients absorbed from the outside gather on the root surface, enriching the nutrients in the rhizosphere micro-region and providing a growth substrate and suitable development environment for soil microorganisms [Citation44, Citation45].
To some extent, the diversity, evenness, dominance and richness of distinct microbial functional diversity indices were evaluated as a whole to indicate differences in soil microbial community composition. Except for the Shannon–Wiener Index and Evenness index of rhizosphere soil microorganisms and the Richness Index of rhizosphere soil of Pueraria lobata, which were lower than those of non-rhizosphere soil, the indices of the rhizosphere soil microorganisms of the other plants were higher than those of the non-rhizosphere soil microorganisms, according to the findings of this study. It is clear that plant restoration has altered the soil microbial community structure of the vegetation concrete substrate and significantly boosted soil microbial functional activity [Citation11, Citation46, Citation47].
Many variables, including plant species, litter input, temporal and geographical variations and soil physicochemical qualities, have been found to influence the types, activities and distribution of soil microbial communities [Citation48]. Song et al. [Citation49] reported a strong or highly significant inverse relationship between soil bulk density and the soil microbial community diversity index. Nitrogen, according to Ren et al. [Citation50] and Dai et al. [Citation47], is an essential element influencing microbial populations. Cao et al. [Citation46] discovered that soil alkali-hydrolysable nitrogen and accessible phosphorus were strongly associated with microbial carbon source consumption in soil. The correlation analysis between the soil microbial functional diversity index and soil factors in this study also revealed that soil microbial Shannon–Wiener index was closely related to pH, and pH was the key factor driving the distribution of soil bacterial community, which was consistent with findings of Shen et al [Citation51]. The correlation between TP, TN and McIntosh index was not significant, unlike the research results of Cheng et al. [Citation52]. The reason may be that the plant root system led to some difference in the characteristics of the rhizosphere and non-rhizosphere soils of the plants, which in turn led to the difference in the results. The carbon source metabolism features of the microbial community in the rhizosphere and non-rhizosphere soil of the three plants soil were studied. The findings revealed that the soil moisture content, organic matter, total nitrogen, pH, microbial carbon, microbial nitrogen and microbial phosphorus were the primary regulators of the carbon source metabolism characteristics in the microbial communities. The functional diversity of the microbial communities in rhizosphere and non-rhizosphere soils of various vegetations could be effectively separated using principal component analysis. Amino acids and carboxylic acids were the key contributions to the separation of the principal components. The improved use of carbohydrates, carboxylic acids and amino acids by soil microbes aided in the transformation of soil accessible nutrients, showing that plant restoration aided in enhancing microorganism activity and hence aided in nutrient transformation [Citation53].
Conclusions
The physical and chemical characteristics, microbial biomass, microbial activity, microbial functional diversity index and carbon source utilization type of vegetation concrete substrate are all influenced differently by various plants. The microbial activity and functional diversity indices of rhizosphere soils were largely higher than those of non-rhizosphere soils, showing that plants have a favorable role in supporting microorganism reproduction and growth in vegetation concrete substrate. The indices of microbial activity and microbial functional diversity in the rhizosphere and non-rhizosphere soil of Arthraxon hispidus and the rhizosphere soil of Pueraria lobata were at a high level, whereas the indices of microbial activity and microbial functional diversity in the rhizosphere and non-rhizosphere soil of Pennisetum alopecuroides and the non-rhizosphere soil of Pueraria were at a relatively low level, which indicated that Arthraxon hispidus and Pueraria lobata had positive significance for the improvement of the rhizosphere microenvironment of the vegetation concrete substrate in Xiangjiaba area. Carbohydrates, amino acids and polymers were the primary carbon sources utilized by the rhizosphere soil microbes of the three dominating plants in the research region. Carboxylic acids, amino acids and polymers were the most common carbon sources used by non-rhizosphere soil microbes. The governing elements of carbon source metabolism in microbial communities include soil moisture content, organic matter, total nitrogen, pH, microbial carbon, microbial nitrogen and microbial phosphorus. The restricted number of carbon sources available on Biolog microplates cannot completely represent the complicated scenario of soil microbial carbon source use. The following stage will employ high-throughput sequencing technology for genomic analysis to further reveal the structure and function of substrate microbial communities, providing a foundation for choosing appropriate species for ecological restoration in the disturbance region.
Author contribution statement
Lu Xia: paper framework, conceiving and planning the experiments, writing and provided funding; Bingqing Zhao: sample collection, data processing, writing; Ting Luo: sample collection, writing; Wennian Xu: data processing, provided funding; Ting Guo: sample collection, data processing; Dong Xia: conceiving and planning the experiments, re-writing, provided funding.
Disclosure statement
No potential conflict of interest was reported by the authors.
Data availability statement
The data that support the findings of this study are openly available in [repository name “Data of soil microbial functional diversity between rhizosphere and non-rhizosphere of different dominant species in vegetation concrete slope”] at https://www.scidb.cn/s/RZviAb, reference number [10.11922/sciencedb.01393].
Additional information
Funding
References
- Fierer N. Embracing the unknown: disentangling the complexities of the soil microbiome. Nat Rev Microbiol. 2017;15(10):579–590.
- Preston S, Wirth S, Ritz K, et al. The role played by microorganisms in the biogenesis of soil cracks: importance of substrate quantity and quality. Soil Biol Biochem. 2001;33(12–13):1851–1858.
- Raaijmakers JM, Mazzola M. ECOLOGY. Soil immune responses. Science. 2016;352(6292):1392–1393.
- Waksman SA. Soil microbiology. Hoboken (NJ): John Wiley & Sons; 1952.
- Prestonmafham J, Boddy L, Randerson PF. Analysis of microbial community functional diversity using sole-carbon-source utilisation profiles – a critique. FEMS Microbiol Ecol. 2006;42(1):1–14.
- Xian G, Hu JL. Scientific connotation and ecological service function of soil microbial diversity. Acta Pedol Sin. 2008;45(5):892–900. (In Chinese).
- Luo XQ, Hao XH, Cheng T, et al. Effects of long-term different fertilization on microbial community functional diversity in paddy soil. Acta Ecol Sin. 2009;29(2):740–748. (In Chinese)
- Liu JW, Li XZ, Yao MJ. Research progress on assembly of plant rhizosphere microbial community. Acta Microbiol Sin. 2021;61(2):231–248. (In Chinese).
- Wang XP, Yang X, Yang N, et al. Effects of litter diversity and composition on litter decomposition characteristics and soil microbial community. Acta Ecol Sin. 2019;39(17):6264–6272. (In Chinese).
- Zheng H. Impacts of litter decomposition of eucalyptus on soil microbial community: A Microcosm Study. Acta Pedol Sin. 2019;56(2):432–442. (In Chinese).
- An SS, Li GH, Chen LD. Soil microbial functional diversity between rhizosphere and non-rhizosphere of typical plants in the hilly area of Southern Nixia. Acta Ecol Sin. 2011;31(18):5225–5234. (In Chinese).
- Chen Y, Lü GH, Li Y. Soil microbial functional diversity of rhizosphere and non-rhizosphere of three dominant herbaceous plants in the Dushanzi district. Acta Ecol Sin. 2018;38(9):122–129. (In Chinese).
- Du YX, Xie BM, Cai HS, et al. Structural and functional diversity of rhizosphere microbial community of nine plant species in the daqing saline-alkali soil region. Acta Ecologica Sinica. 2016;36(3):740–747. (In Chinese)
- Nicolitch O, Colin Y, Turpault MP, et al. Soil type determines the distribution of nutrient mobilizing bacterial communities in the rhizosphere of beech trees. Soil Biol Biochem. 2016;103:429–445.
- Feng W, Guan T, Wang XY, et al. Effects of combined application of biogas slurry and chemical fertilizer on winter wheat rhizosphere soil microorganisms and enzyme activities. Chin J Appl Ecol . 2011;22(4):1007–1012. (In Chinese).
- Ma K, Yang JL, Ma L, et al. Effects of intercropping on soil microbial communities after long-term potato monoculture. Acta Ecol Sin. 2016;36(10):2987–2995. (In Chinese).
- Wei J, Liu X, Zhang X, et al. Rhizosphere effect of Scirpus triqueter on soil microbial structure during phytoremediation of diesel-contaminated wetland. Environ Technol. 2014;35(4):514–520.
- Xu WN, Wang TQ. Greening additives for use in concrete. Chinese Patent Publication No. CN 1358685A. 21 December 2001; 2001. (In Chinese).
- Ding Y, Hu WJ, Xia ZY, et al. Soil fertility dynamics of substrate used for ecological slope protection. Journal of Hydroecology. 2017;38(2):31–37. (In Chinese).
- Zhao BQ, Xia ZY, Xu WN, et al. Review on research of slope eco-restoration technique for engineering disturbed area. Water Resourc Hydropower Engin. 2017;48(2):130–137.
- Berg G, Smalla K. Plant species and soil type cooperatively shape the structure and function of microbial communities in the rhizosphere. FEMS Microbiol Ecol. 2009;68(1):1–13.
- Lal R, Mokma D, Lowery B. Relation between soil quality and erosion. In: Lal R, editor. Soil quality and soil erosion. Boca Raton (FL): CRC Press; 2018. pp.237–258.
- Zhou J, Lei T. Review and prospects on methodology and affecting factors of soil microbial diversity. Biodiversity Sci. 2007;15(3):306–311. (In Chinese).
- Riley D, Barber SA. Bocarbonate accumulation and pH changes at the soybean (Glycine max (L.) Merr.) root-soil interface. Soil Sci Soc Am J. 1969;33(6):905–908.
- Zhang JE. Usual experimental methods and technology in ecology. Beijing: Chemical Industry Press; 2007. (In Chinese).
- Tokeshi M, Kitamori T. Advances in flow analysis. Hoboken (NJ): John Wiley & Sons; 2008.
- Brookes PC, Landman A, Pruden G, et al. Chloroform fumigation and the release of soil nitrogen: a rapid direct extraction method to measure microbial biomass nitrogen in soil. Soil Biol Biochem. 1985;17(6):837–842.
- Brookes PC, Powlson DS, Jenkinson DS. Phosphorus in the soil microbial biomass. Soil Biol Biochem. 1984;16(2):169–175.
- Vance ED, Brookes PC, Jenkinson DS. An extracted method for measuring soil microbial biomass C. Soil Biol Biochem. 1987;19(6):703–707.
- Schutter M, Dick R. Shifts in substrate utilization potential and structure of soil microbial communities in response to carbon substrates. Soil Biol Biochem. 2001;33(11):1481–1491.
- Shao YY, Wang ZY, Zou L. Effect of chlorothalonil on soil microbial communities of larix artificial shelter-Forest. Acta Ecol Sin. 2011;31(3):818–829. (In Chinese).
- Cao HJ, Wang LM, Xu MY, et al. Effect of vegetation type on the diversity of soil microbial communities at the new stage volcanic lava platform, Wudalianchi area, Northeast China. Acta Ecol Sin. 2019;39(21):7927–7937. (In Chinese).
- Li F, Kong Q, Zhang Q, et al. Spent mushroom substrates affect soil humus composition, microbial biomass and functional diversity in paddy fields. Appl Soil Ecol. 2020;149(1192):103489.
- Zhang C, Liu GB, Xue S, et al. Functional diversity of rhizosphere microbial community of different vegetation types in the hilly-gully region of loess Plateau. Acta Agrestia Sin. 2015;23(4):710–717. (In Chinese).
- Fisk MC, Ruether KF, Yavitt JB. Microbial activity and functional composition among Northern peatland ecosystems. Soil Biol Biochem. 2003;35(4):591–602.
- Kaiser O, Puhler A, Selbitschka W. Phylogenetic analysis of microbial diversity in the rhizoplane of oilseed rape (Brassica napus cv. Westar) employing cultivation-dependent and cultivation-independent approaches. Microb Ecol. 2001;42(2):136–149.
- Dardanelli MS, Manyani H, González-Barroso S, et al. Effect of the presence of the plant growth promoting rhizobacterium (PGPR) Chryseobacterium balustinum Aur9 and salt stress in the pattern of flavonoids exuded by soybean roots. Plant Soil. 2010;328(1–2):483–493.
- Wang CY, Cao Z, Wang L. Eological effects of leguminous plants on microorganism community in rhizosphere soils. Ecol Environ Sci. 2013;22(1):85–89. (In Chinese).
- Li Z, Zhao YJ, Song HY, et al. The effects of soil thickness heterogeneity on grassland plant community structure and growth of dominant species in karst area. Pratacultural Sci. 2017;34(10):2023–2032. (In Chinese).
- Sun S, Zhang XJ, Liu JP, et al. Synergistic effects of shade and drought on the physiological metabolism and resistance system of Arthraxon hispidus. Acta Ecol Sin. 2018;38(5):1770–1779. (In Chinese).
- Qiu Q, Li JY, Wang JH, et al. Microbes, enzyme activities and nutrient characteristics of rhizosphere and non-rhizosphere soils under four shrubs in Xining Nanshan, Prefecture, China. Acta Ecol Sin. 2014;34(24):7411–7420. (In Chinese).
- Gong S, Guo R, Zhang T, et al. Warming and nitrogen addition increase litter decomposition in a temperate meadow ecosystem. PLoS ONE. 2015;10(3):e0116013.
- Vieira S, Sikorski J, Gebala A, et al. Bacterial colonization of minerals in grassland soils is selective and highly dynamic. Environ Microbiol. 2020;22(3):917–933.
- Li CJ, Li Y, Ma J, et al. Nutrition in the rhizosphere of five xerophy pants. Arid Land Geography. 2011;34(2):222–228. (In Chinese).
- Rouatt JW, Katznelson H, Payne TMB. Statistical evaluation of the rhizosphere effect. Soil Sci Soc Am J. 1960;24(4):271–273.
- Cao YC, Yang R, Liu S, et al. Characteristics of microbial community in Forest soil between rhizosphere and non-rhizosphere in summer and autumn in Qinling mountains, China. Acta Ecol Sin. 2017;37(5):1667–1676. (In Chinese).
- Dai YT, Hou XY, Yan ZJ, et al. Rhizosphere microbial functional diversity affected by vegetation restoration in the Hobq sand land, Inner Mongolia, China. Acta Pratacultural Sin. 2016;25(10):56–65.
- Kowalchuk GA, Buma DS, Boer DW, et al. Effects of above-ground plant species composition and diversity on the diversity of soil-borne microorganisms. Antonie Van Leeuwenhoek. 2002;81(1-4):509–520.
- Song XC, Wang HL, Qin WD, et al. Effects of stand type of artificial forests on soil microbial functional diversity. Chin J Appl Ecol . 2019;30(3):841–848. (In Chinese).
- Ren ML, Wang SM, Zhang X, et al. Rhizosheath soil microbial functional diversity of two typical Gramineae plants in the Southern margin of the Junggar basin. Acta Ecol Sin. 2017;37(17):5630–5639. (In Chinese).
- Shen C, Xiong J, Zhang H, et al. Soil pH drives the spatial distribution of bacterial communities along elevation on Changbai Mountain. Soil Biol Biochem. 2013;57:204–211.
- Cheng H, Xu W, Luo T, et al. Microbial functional diversity as affected by different engineered eco-restoration methods at Xiangjiaba hydropower station. J Environ Eng. 2020;146(3):04019125.
- Shao L, Gu J, Zhang SQ, et al. Effects of bio-compound fertilizer on corn soil microbial community and enzyme activities. J Agro-Environ Sci. 2012;31(6):1153–1159. (In Chinese).