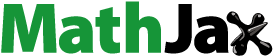
Abstract
Drought stress is a major limiting factor for soybean production. In this study, protein elicitor AMEP412 was applied on soybean seedlings to promote drought tolerance, and its correlation with alterations in the reactive oxygen species (ROS) in leaves was studied. The plants of soybean cv. Suinong 26 were sprayed with AMEP412 at the V1 stage and then treated with drought stress and rehydration. The alterations in phenotypes, physiological indices, ROS levels and antioxidant system as well as lipid peroxidation were monitored. The results showed that spraying AMEP412 significantly promoted the drought tolerance of soybean seedlings. Soybean seedlings pre-treated with AMEP412 performed better in phenotypes and physiological indexes, like leaf relative water content (RWC), leaf relative conductivity (RC) and osmolytes (free proline, soluble sugar and protein). In addition, the in situ and quantification detection of ROS revealed that AMEP412 pretreatment led to higher accumulation of ROS at the early stage and faster scavenging of ROS at the late stage. Moreover, AMEP412 pretreatment increased the activity of superoxide dismutase (SOD), guaiacol peroxidase (POD), catalase (CAT), ascorbate peroxidase (APX), monodehydroascorbate reductase (MDHAR), dehydroascorbate reductase (DHAR), glutathione reductase (GR), glutathione peroxidase (GPX), and the levels of ascorbate (AsA) and glutathione (GSH), but decreased the level of malondialdehyde (MDA) as compared with only drought treatment. Overall, the results indicated that AMEP412 was effective in alleviating the adverse effects of drought stress, which was partially attributable to the ROS accumulation and scavenging triggered by AMEP412.
Introduction
As a major agricultural crop, soybean (Glycine max) provides large amounts of vegetable protein and edible oil for humans and animals. However, the growth and yield of soybean are highly affected by drought stress [Citation1]. Many references have reported the yield reduction caused by drought stress [Citation2–4]. Thus there is a great need of applicable means to improve the drought tolerance of soybean.
As a kind of eco-friendly exogenous bio-stimulants, protein elicitors could induce plant biotic and abiotic stress resistance via signal recognition, signal transduction and defense gene regulation [Citation5–7]. Many protein elicitors have been used to improve the drought resistance of plants [Citation8–11]. AMEP412 is a newly identified protein elicitor from Bacillus subtilis, which could trigger plant defense response and promote plant resistance [Citation12]. In our previous field application, AMEP412 treated soybean plants showed obvious drought tolerance, and resulted in less loss of the yield (unpublished data). However, the underlying mechanism still needed investigation.
Reactive oxygen species (ROS) are an unavoidable chemical entity of aerobic life [Citation13]. Under normal conditions, ROS are continuously produced in plants at a relatively low level. When plants are exposed to environmental stress, the level of ROS increases significantly to activate stress related signaling pathways. However, further accumulation of ROS will result in oxidative damage and even programmed cell death [Citation14–17]. Both drought stress and protein elicitors could trigger ROS production [Citation18,Citation19], indicating that the crosstalk between the two different external stimulants at ROS level might play a key role in the drought resistance induced by protein elicitors.
In this study, we performed AMEP412 treatment on soybean at the seedling stage, followed by the drought stress and rehydration. The effects of AMEP412 application on phenotypes, physiological indexes, ROS production and scavenging were monitored, and the influences of the altered ROS levels on antioxidant and lipid peroxidation system were also investigated. Our goal was to explore the soybean drought tolerance improved by AMEP412 at ROS level, and to provide experience for field application.
Materials and methods
Plant materials and treatments
A pot experiment was conducted in the greenhouse at Heilongjiang Bayi Agricultural University, Daqing, Heilongjiang Province, China (45°50´N, 124°15´E) in the summer of 2021. The soybean cultivar Suinong 26 was used in the experiment. Soybean seeds were planted in plastic pots (7.5 cm in height and 9.3 cm in diameter) containing 0.4 kg soil, and six seeds were planted per pot. After one week, they were thinned to four seedlings of uniform size per pot. At the V1 stage, combinations of four different treatments were initiated: (i) water control (CK); (ii) drought stress (DS); (iii) water control treated with AMEP412 (ACK); (iv) drought stress pre-treated with AMEP412 (ADS). AMEP412 treatment was executed by spraying 100 μg/mL purified protein [Citation12] on leaves (treatments iii and iv), whereas the others were sprayed with distilled water (treatments i and ii). The spray treatment was done only once, with 2 mL of protein solution or water per pot. On the same day, the drought treatments were set up by withholding water for 5 days and, subsequently, resupplying water at 10% (w/w) for another 5 days for the stressed plants to recover. In parallel, the control pots were watered daily at 10% (w/w) for 10 days. At the 1st, 3rd and 5th days of drought (DAD), and the 1st, 3rd and 5th days after resupplying water (DRW), leaf samples of each treatment were collected in triplicate for direct determination, or frozen in liquid nitrogen and stored at −80 °C for extraction.
Phenotype and physiological indexes
The phenotypes of the soybean plants in each treatment were recorded under control, drought stressed conditions and water resupplied conditions.
The leaf relative water content (RWC) was calculated following Smart and Bingham’s method [Citation20]. Once the leaf fresh weight (LFW) was determined, the samples were soaked in distilled water for 24 h. Then the leaf turgid weight (LTW) of each sample was recorded. To determine the leaf dry weight (LDW), the leaf tissues were oven-dried at 70 °C for 72 h before weight was recorded. Finally, the RWC was calculated based on the following equation: RWC (%) = [(LFW-LDW)/(LTW-LDW)] × 100.
The leaf relative conductivity (RC) was measured according to the method of Blum and Ebercon [Citation21] with modifications. Leaves were excised and immersed in distilled water in test tubes overnight at room temperature. The conductivities of the water before boiling (R1) and after boiling (R2) were determined, and the electrolyte leakage in leaves was calculated based on the formula: RC (%) = (R1/R2)×100.
Proline content was measured according to a method previously published by Bates et al. [Citation22]. Free proline was extracted from 0.5 g leaf sample with a 5 mL extraction solution of 3% aqueous sulfosalicylic acid. The homogenate was centrifuged at 12 000×g for 15 min. Then 2 mL of supernatant was incubated with 2 mL of glacial acetic acid and 2 mL acid ninhydrin at 100 °C for 1 h. After cooling the samples at room temperature, 5 mL toluene was added and mixed by vortexing. The absorbance was recorded at 520 nm.
The soluble sugar content was determined by spectrophotometer at 640 nm using an anthrone reagent [Citation23], and the calibration curve was prepared using glucose as the standard. The soluble protein content was determined by the Brilliant Blue G-250 regent [Citation24], using bovine serum albumin (BSA) as a standard.
In situ detection of ROS
Accumulation of hydrogen peroxide was detected by a peroxidase-dependent in situ histochemical staining procedure using 3,3-diaminobenzidine (DAB) [Citation25] and superoxide ion using a superoxide-dependent reduction of nitro blue tetrazolium (NBT) [Citation26]. Leaves were cut and then vacuum-infiltrated with 1 mg/mL DAB (pH 3.8) or 1 mg/mL NBT for 2 h. The treated leaves were incubated for more than 24 h in 70% ethanol and 5% glycerol to eliminate chlorophyll, observed for DAB and NBT deposits, and photographed.
Quantification of ROS production
The production of H2O2 was determined using the method described by Tiedemann [Citation27]. Leaf squares were washed using distilled water and incubated in 2 mL of a reagent mixture consisting of 50 mmol/L phosphate buffer pH 7.0, 0.05% guaiacol and 2500 U/mL peroxidase (EC 1.11.1.7, Sigma Aldrich, P8375) for 2 h at room temperature in the dark. The release of H2O2 was followed by measurement of absorbance at 450 nm.
The O2 generation was quantified based on NBT reduction activity, as described by El Hadrami et al. [Citation28]. Leaf squares were washed using distilled water and immersed in 10 mmol/L potassium phosphate buffer, pH 7.8, containing 0.05% NBT and 10 mmol/L NaN3 for 1 h. This mixture was heated at 85 °C for 15 min and then cooled quickly. The reduction activity of the soybean leaf was followed by measuring the absorbance at 580 nm.
Measurement of SOD, POD and CAT activities
Frozen leaves (0.5 g) were ground to a powder in liquid nitrogen and homogenized with 5 mL of 50 mmol/L sodium phosphate buffer (pH 7.8 for SOD and POD, and pH 7.0 for CAT) containing 0.1 mmol/L ethylene diamine tetraacetic acid (EDTA) and 1% (w/v) polyvinylpyrrolidone (PVP). The homogenate was centrifuged at 15 000×g for 30 min at 4 °C and the supernatant was used for measuring enzyme activities.
SOD activity was assayed by measuring the inhibition of NBT reduction according to the inhibition of nitro blue tetrazolium reduction at 560 nm [Citation29]. POD activity was measured according to the changes in absorption at 470 nm due to guaiacol oxidation [Citation30]. CAT activity was determined by measurement of the differential values between original and residual H2O2 in the reaction solution at 240 nm [Citation31]. All spectrophotometric analyses were performed on a UV-VIS spectrophotometer (ONLAB, EU-2000A).
Measurement of APX, MDHAR, DHAR, GR and GPX activities
Frozen leaves (0.5 g) were ground to a powder in liquid nitrogen and homogenized with 5 mL of 50 mmol/L sodium phosphate buffer (pH 7.5) containing 0.1 mmol/L Na2EDTA, 0.3% Triton X-100 and 4% (w/v) PVP. The homogenate was centrifuged at 15 000×g for 30 min at 4 °C. The supernatant were used for measurement of enzyme activities.
APX activity was assayed by monitoring the rate of reduced ascorbate acid (AsA) oxidation at 290 nm according to Nakano and Asada’s method [Citation32]. MDHAR activity was measured by recording the decrease in absorbance at 340 nm due to oxidation of reduced nicotinamide adenine dinucleotide phosphate (NADPH), as described by Murshed et al. [Citation33]. The DHAR activity assay was carried out by measuring the increase in absorbance at 265 nm due to AsA formation, according to Nakano and Asada’s method [Citation32].
GR activity was measured by monitoring the oxidized glutathione (GSSG)-dependent oxidation of NADPH at 340 nm for 3 min, according to the method of Schaedle and Bassham [Citation34]. GPX activity was assayed by measuring the rate of GSH-dependent oxidation of NADPH at 340 nm, as described by Navari-Izzo et al. [Citation35].
Measurement of antioxidants (AsA and GSH)
Frozen leaves (0.5 g) were ground to a powder in liquid nitrogen and homogenized with 5 mL of 5% sulfosalicylic acid and centrifuged at 15 000×g for 30 min, and the supernatant was used for AsA and GSH assays. The AsA concentration was determined based on the reduction of ferric to ferrous ion with AsA in acid solution followed by the formation of the red chelate between ferrous ion and 2,2′-dipyridyl [Citation36]. The total GSH was determined by the 5,5′-dithio-bis-nitrobenzoic acid (DTNB)-GR recycling procedure [Citation37].
Measurement of MDA
Malondialdehyde (MDA) content was measured by the thiobarbituric acid (TBA) reaction as described by Heath and Packer [Citation38], and was used to estimate lipid peroxidation. Frozen leaves (0.5 g) were ground to a powder in liquid nitrogen and homogenized with 5 mL of 5% trichloroacetic acid (TCA). The homogenate was centrifuged at 15 000×g for 10 min. The supernatants were mixed with the same volume of 20% TCA containing 0.5% TBA and kept at 95 °C for 30 min. The mixture was cooled rapidly and centrifuged again. The optical density of the supernatant was measured at 532 and 600 nm.
Data analysis
SPSS 17.0 and Origin 8.0 were used for data collation and statistical analysis. The results presented are the mean values of three replicates with standard error of the means (±SEM). Duncan’s multiple range tests compared the treatment means, with differences considered statistically significant at the p ≤ 0.05 level.
Results
Phenotype and physiological indexes
Phenotype can directly reflect the drought resistance of soybean plants. As shown in , at 5DAD, the growth status of soybean plants (DS) was seriously suppressed, with most leaves wilted. In contrast, there were no visible wilting symptoms in AMEP412-pretreated soybean plants (ADS). At 5DRW, the soybean plants in DS and ADS treatments generally recovered from the drought stress (). However, in DS treatment, permanent wilting was found on leaves at bottom layers. Compared with water controls, drought stress apparently affected the growth status of soybean plants, while AMEP412 pretreatment reduced the damage caused by drought stress. There was no significant difference in phenotypes between CK and ACK treatments.
Figure 1. Phenotypic appearance of soybean plants under different treatments. (A) The phenotypes at 5 days after drought (DAD). (B) The phenotypes at 5 days after resupplying water (DRW). CK, water control; DS, drought stress; ACK, water control treated with AMEP412; ADS, drought stress pre-treated with AMEP412. Same below.
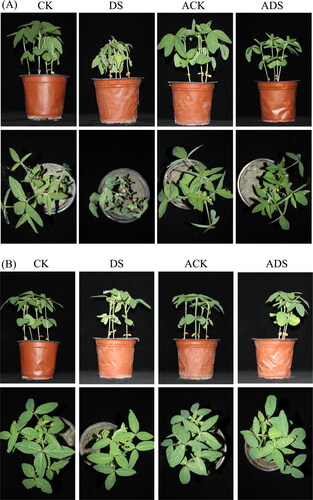
Variations in RWC and RC were used as parameters to determine the stress status of plants [Citation39]. The free proline, soluble sugar and soluble protein contents play important roles in osmoregulation and dehydration prevention under drought stress [Citation40]. In the present study, drought stress significantly decreased the leaf RWC, but increased the leaf RC and osmolytes (free proline, soluble sugar and soluble protein) contents. Then all the indexes tended to recover gradually after rehydration (). Most of the peak and valley values appeared at 5DAD except for those of soluble sugar and soluble protein (peaked at 1DRW). Compared with DS treatment, ADS treatment significantly changed the values of RWC, RC, free proline, soluble sugar and soluble protein by 13.3%, 27.3%, 28.2%, 27.3%, and 7.6% at the peak and valley points, respectively (p < 0.05). In the non-drought stressed treatments (CK and ACK), AMEP412 also resulted in a similar but minor trend of the above indexes.
Figure 2. Alterations in the physiological indexes of different treatments throughout the experimental period. (A) RWC; (B) RC; (C) free proline; (D) soluble sugar; (E) soluble protein. Means denoted by the same letters did not differ significantly at p ≤ 0.05 according to Duncan’s multiple range test. Error bars show ± SEM. Same below.
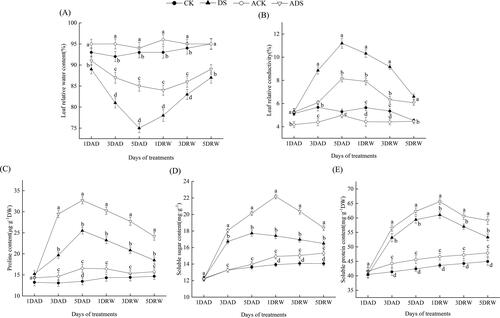
ROS determination
The in situ detection of ROS (H2O2 and O2,) was executed by DAB and NBT staining, respectively. The straining degrees showed positive correlations with the ROS levels. As shown in , in drought stress treatments (DS and ADS), significant ROS accumulations were observed at 5DAD and 5DRW. The difference probably lay in that the cleanup of ROS in AMEP412 pretreatment (ADS) was more effective, as compared with DS treatment. Certain amounts of staining spots were monitored in ACK treatment at 5DAD and 5DRW, indicating AMEP412 application could also trigger ROS accumulation throughout the experimental process.
Figure 3. DAB and NBT staining to assess H2O2 (A) and O2 (B) production in soybean leaves in the different treatments.
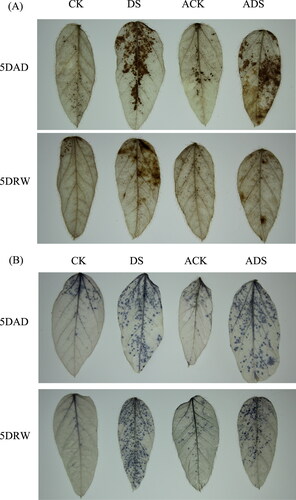
The quantification determination () showed that drought stress obviously elevated both H2O2 and O2 levels (DS and ADS), which decreased gradually after rehydration. H2O2 () and O2
() contents showed early accumulation in ADS treatment at 3 DAD, which increased by 8.7 and 17.2%, as compared with the DS treatment, respectively (p < 0.05). From 5DAD to 3DRW, the H2O2 and O2
parameters had no significant differences between DS and ADS treatments. However, they showed a significant decline in ADS treatment at 5DRW, which decreased by 4.6 and 14.3%, as compared with the DS treatment, respectively (p < 0.05). In the non-drought stressed treatments (CK and ACK), AMEP412 led to a slight but non-significant increase in ROS levels.
Antioxidant enzyme activities
Plants employ many different types of antioxidant enzymes to work together to remove excessive ROS effectively [Citation41]. SOD is a major scavenger of O2, catalyzing its dismutation into H2O2 and O2. Both CAT and POD scavenge the accumulated H2O2 to non-toxic levels by converting it into H2O and O2. In the AsA-GSH cycle [Citation42], APX utilizes AsA as a specific electron donor to reduce H2O2 to water, and AsA is then regenerated by the action of MDHAR and DHAR. GPX catalyzes the reduction of H2O2 to water by GSH, and GR catalyzes the NADPH-dependent regeneration of GSH.
In this experiment (), drought stress showed significant effect on the activities of the antioxidant enzymes, and peak values were obtained at 5DAD (GR and GPX) and 1DRW (SOD, POD, CAT, APX, MDHAR and DHAR). Then the recovered plants showed a gradual decline in enzyme activities. Notably, AMEP412 pretreatment further enhanced the alterations of antioxidant enzyme activities. ADS treatment significantly increased the SOD, POD, CAT, APX, MDHAR, DHAR, GR and GPX activities by 17.3%, 23.7%, 9.2%, 15.8%, 23.2%, 19.0%, 36.4% and 10.7% at the peak points, respectively, over DS treatment (p < 0.05). Furthermore, even at 5DRW, most of the enzyme activities (except for MDHAR and GPX) of the ADS treatment still kept at significantly higher levels, as compared with the DS treatment (p < 0.05). In water controls (CK and ACK), AMEP412 application also induced a significant but minor increase in some antioxidant enzyme activities (POD, APX and GPX).
Antioxidants
Antioxidants (AsA and GSH) play important roles in scavenging ROS through the AsA-GSH cycle [Citation43]. Drought stress treatments (DS and ADS) significantly increased the AsA content () and GSH cotntent (), which peaked at 1DRW and then decreased. In comparison with the drought-only treatment (DS), the AsA and GSH levels in the AMEP412 pretreatment (ADS) were generally elevated, increasing by 38.5% and 16.2% at 1DRW, respectively (p < 0.05). In water controls, AMEP412 treatment (ACK) also led to a significant increase in GSH content, when compared with CK treatment.
Lipid peroxidation
The degree of membrane lipid peroxidation in soybean leaves can be reflected by MDA content, which reflects the degree of cell membrane damage [Citation44]. As shown in , marked enhancement in MDA content was found under drought stress conditions (DS and ADS), with a peak at 1DRW. ADS treatment continuously decreased the MDA content, which was 27.4% lower than that of DS treatment at 1DRW (p < 0.05). Under water conditions (CK and ACK), the lipid peroxidation did not show significant changes.
Discussion
AMEP412, a protein elicitor produced by B. subtilis, is recently known as a biological agent for improving plant health by triggering plant immunity, inhibiting plant pathogens and killing harmful insects [Citation12,Citation45,Citation46]. In this study, for the first time, the drought tolerance of soybean plants induced by AMEP412 was reported. During the process of drought stress and rehydration, no obvious wilting leaves were observed with the application of AMEP412, while the physiological parameters also agree with the symptoms. Compared with drought stress control, AMEP412 increased the ROS burst degree at the early stage, which induced higher production of antioxidant enzymes, antioxidants and osmolytes. Then the enhanced antioxidant system fastened the cleanup of ROS and alleviated the lipid peroxidation, which protected soybean plants from the damage caused by drought stress. This result enriched our knowledge of the function of AMEP412 and broadened its potential application scale.
Under drought stress, a rapid ROS accumulation would occur, leading to negative impact on antioxidant metabolism, and consequently cell peroxidation damage [Citation47,Citation48]. Plants have evolved enzymatic and non-enzymatic antioxidant systems to scavenge excessive ROS, which keep the cellular ROS level at a steady state. Once this balance is broken by continuous drought stress, oxidative damage and wilting symptoms appear. In this study, the soybean seedlings in DS treatment showed obvious wilting symptoms at 5DAD, indicating that the balance of ROS generation and scavenging was disturbed. The soybean seedlings in ADS treatment did not show wilting symptoms, implying the scavenging of ROS could still keep pace with the accumulation of ROS. Following rehydration, the production of ROS was restrained and the balance shifted to ROS scavenging, resulting in the recovery of the soybean plants from drought stress.
Apart from the destructive role of ROS, an early rise in ROS level could act as a signal for acclimation or defense response, which serves positive roles in plant growth and development [Citation49]. By applying protein elicitors on plants, the signaling role of ROS has been understood well in the case of defense against pathogens [Citation50], promotion in growth [Citation51] and tolerance to drought stress [Citation52]. In our previous study, we found that AMEP412 could trigger ROS burst and defensive enzymes expression, and then led to the defense response against disease [Citation12]. In this study, the pretreatment with AMEP412 elevated the production of ROS at the early stage, and then led to an enhanced response of the ROS-scavenging system throughout the whole experimental process, resulting in the drought tolerance of soybean seedlings. This result suggested that the ROS accumulation trigged by AMEP412 activated certain downstream signaling cascades, and finally facilitated the ROS scavenging, which might partially be the mechanism underlying the drought tolerance induced by AMEP412.
It is now well established that both drought stress and protein elicitors could induce ROS to some degree [Citation53]. In drought stress related references, the downstream signaling of ROS is likely to occur via calcium and reversible protein phosphorylation [Citation54,Citation55], which refer to Ca2+ channels and mitogen-activated protein kinases (MAPKs), respectively. Furthermore, the levels of phytohormones like abscisic acid (ABA) and ethylene could also be modulated by ROS [Citation56–58]. In plant immunity related reports, Ca2+ signaling and MAPK cascades could also be activated by ROS burst [Citation59–61], as well as downstream pathways mediated by hormones like salicylic acid (SA), jasmonic acid (JA) and ethylene [Citation62,Citation63]. Taken together, these observations suggest that ROS signaling induced by protein elicitors could evoke downstream responses that contribute to drought tolerance. The present study further indicated the existence of crosstalk between the two different external stimulants at ROS level.
In the present research, the drought tolerance of soybean improved by AMEP412 was only investigated at the seedling stage. However, drought stress at flowering and pod filling stages affects the soybean yield more directly [Citation64]. So the influence of AMEP412 on soybean plants at these other stages will be studied in subsequent research. Furthermore, besides ROS, more aspects like photosynthesis, hormone balance and signaling transduction will be involved for better understanding of the underlying mechanism.
Conclusions
Drought stress remarkably affected the phenotypes and physiological indexes of soybean seedlings. As a response to drought stress, ROS were generated dramatically, followed by great alterations in the antioxidant and lipid peroxidation system. By application of AMEP412 before drought stress, the phenotypes of soybean plants gained notable improvements. Improvements were seen in the physiological indexes, like RWC, RC and osmolytes as well. Besides, higher accumulation of ROS was observed at the early stage, which led to enhanced antioxidative protection and resulted in accelerated ROS scavenging and decreased lipid peroxidation. Altogether the results suggested that AMEP412 obviously promoted the drought tolerance of soybean seedlings, which was at least partially attributed to the improvement at the ROS level.
Author contributions
Quan Liu conceived and designed the experiments. Quan Liu and Siwen Wang performed the experiments. Quan Liu wrote the manuscript under the guidance of Yanli Du and Kuide Yin. All authors reviewed and approved the manuscript.
Acknowledgements
The authors thank the reviewers, whose comments and suggestions helped us to improve this manuscript.
Disclosure statement
No potential conflict of interest was reported by the authors.
Data availability statement
All data that support the findings of this study are available from the corresponding author upon reasonable request.
Additional information
Funding
References
- Brown EA, Caviness CE, Brown DA. Response of selected soybean cultivars to soil moisture deficit. Agron J. 1985;77(2):274–278.
- Eck HV, Mathers AC, Musick JT. Plant water stress at various growth stages and growth and yield of soybeans. Field Crop Res. 1987;17(1):1–16.
- Desclaux D, Huynh TT, Roumet P. Identification of soybean plant characteristics that indicate the timing of drought stress. Crop Sci. 2000;40(3):716–722.
- Liu F, Anderse MN, Jensen CR. Loss of pod set caused by drought stress is associated with water status and ABA content of reproductive structures in soybean. Funct Plant Biol. 2003;30(3):271–280.
- Durrant WE, Dong X. Systemic acquired resistance. Annu Rev Phytopathol. 2004;42:185–209.
- Dodds PN, Rathjen JP. Plant immunity: towards an integrated view of plant-pathogen interactions. Nat Rev Genet. 2010;11(8):539–548.
- Mishra AK, Sharma K, Misra RS. Elicitor recognition, signal transduction and induced resistance in plants. J Plant Interact. 2012;7(2):95–120.
- Zhang Y, Yang X, Liu Q, et al. Purification of novel protein elicitor from Botrytis cinerea that induces disease resistance and drought tolerance in plants. Microbiol Res. 2010;165(2):142–151.
- Wang B, Yang X, Zeng H, et al. The purification and characterization of a novel hypersensitive-like response-inducing elicitor from Verticillium dahliae that induces resistance responses in tobacco. Appl Microbiol Biotechnol. 2012;93(1):191–201.
- Shi F, Dong Y, Zhang Y, et al. Overexpression of the PeaT1 elicitor gene from alternaria tenuissima improves drought tolerance in rice plants via interaction with a myo-inositol oxygenase. Front Plant Sci. 2017;8(970):970.
- Zhou X, Chen Y, Zhao Y, et al. The application of exogenous PopW increases the tolerance of solanum lycopersicum L. to drought stress through multiple mechanisms. Physiol Mol Biol Plants. 2020;26(12):2521–2535.
- Shen Y, Li J, Xiang J, et al. Isolation and identification of a novel protein elicitor from a Bacillus subtilis strain BU412. AMB Express. 2019;9(1):117.
- Halliwell B. Reactive species and antioxidants. Redox biology is a fundamental theme of aerobic life. Plant Physiol. 2006;141(2):312–322.
- Cruz de Carvalho MH. Drought stress and reactive oxygen species: production, scavenging and signaling. Plant Signal Behav. 2008;3(3):156–165.
- Yasar F, Ellialtioglu S, Yildiz K. Effect of salt stress on antioxidant defense systems, lipid peroxidation, and chlorophyll content in green bean. Russ J Plant Physiol. 2008;55(6):782–786.
- Gill SS, Tuteja N. Reactive oxygen species and antioxidant machinery in abiotic stress tolerance in crop plants. Plant Physiol Biochem. 2010;48(12):909–930.
- Miller G, Suzuki N, Ciftci-Yilmaz S, et al. Reactive oxygen species homeostasis and signalling during drought and salinity stresses. Plant Cell Environ. 2010;33(4):453–467.
- Smirnoff N. The role of active oxygen in the response of plants to water deficit and desiccation. New Phytol. 1993;125(1):27–58.
- Torres MA, Jones JD, Dangl JL. Reactive oxygen species signaling in response to pathogens. Plant Physiol. 2006;141(2):373–378.
- Smart RE, Bingham GE. Rapid estimates of relative water content. Plant Physiol. 1974;53(2):258–260.
- Blum A, Ebercon A. Cell membrane stability as measurement of drought and heat tolerance in wheat. Crop Sci. 1981;21(1):43–47.
- Bates LS, Waldren RP, Teare ID. Rapid determination of free proline for water-stress studies. Plant Soil. 1973;39(1):205–207.
- Yemm EW, Willis AJ. The estimation of carbohydrates in plant extracts by anthrone. Biochem J. 1954;57(3):508–514.
- Bradford MM. A rapid and sensitive method for the quantification of microgram quantities of protein utilizing the principle of protein-dye binding. Anal Biochem. 1976;72(1–2):248–256.
- Thordal-Christensen H, Zhang Z, Wei Y, et al. Subcellular localization of H2O2 in plants. H2O2 accumulation in papillae and hypersensitive response during the barley-powdery mildew interaction. Plant J. 1997;11(6):1187–1194.
- Doke N. Generation of superoxide anion by potato tuber protoplasts during the hypersensitive response to hyphal wall components of phytophthora infestans and specific inhibition of the reaction by suppressors of hypersensitivity. Physiol Plant Pathol. 1983;23(3):359–367.
- Tiedemann AV. Evidence for a primary role of active oxygen species in induction of host cell death during infection of bean leaves with Botrytis cinerea. Physiol Plant Pathol. 1997;50(3):151–166.
- El Hadrami A, Kone D, Lepoivre P. Effect of juglone on active oxygen species and antioxidant enzymes in susceptible and partially resistant banana cultivars to black leaf streak disease. Eur J Plant Pathol. 2005;113(3):241–254.
- Dhindsa RS, Plumb-Dhindsa P, Thorpe TA. Leaf senescence: correlated with increased levels of membrane permeability and lipid peroxidation and decreased levels of superoxide dismutase and catalase. J Exp Bot. 1981;32(1):93–101.
- Chance B, Maehly AC. Assay of catalases and peroxidases. Meth Enzymol. 1955;2:764–775. [Database][Mismatch
- Havir EA, Mchale NA. Biochemical and developmental characterization of multiple forms of catalase in tobacco leaves. Plant Physiol. 1987;84(2):450–455.
- Nakano Y, Asada K. Hydrogen peroxide is scavenged by ascorbate-specific peroxidase in spinach chloroplasts. Plant Cell Physiol. 1981;22(5):867–880.
- Murshed R, Lopez-Lauri F, Sallanon H. Microplate quantification of enzymes of the plant ascorbate-glutathione cycle. Anal Biochem. 2008;383(2):320–322.
- Schaedle M, Bassham JA. Chloroplast glutathione reductase. Plant Physiol. 1977;59(5):1011–1012.
- Navari-Izzo F, Meneguzzo S, Loggini B, et al. The role of the glutathione system during dehydration of boea hygroscopica. Physiol Plant. 1997;99(1):23–30.
- Law MY, Charles SA, Halliwell B. Glutathione and ascorbic acid in spinach (spinacia oleracea) chloroplasts. The effect of hydrogen peroxide and of paraquat. Biochem J. 1983;210(3):899–903.
- Griffith OW. Determination of glutathione and glutathione disulfide using glutathione reductase and 2-vinylpyridine. Anal Biochem. 1980;106(1):207–212.
- Heath RL, Packer L. Photoperoxidation in isolated chloroplasts. I. Kinetics and stoichiometry of fatty acid peroxidation. Arch Biochem Biophys. 1968;125(1):189–198.
- Lobato AKS, Neto CFO, Filho BGS, et al. Physiological and biochemical behavior in soybean (Glycine max cv. Sambaiba) plants under water deficit. Aust J Crop Sci. 2008;2:25–32.
- Sharma P, Jha AB, Dubey RS, et al. Reactive oxygen species, oxidative damage, and antioxidative defense mechanism in plants under stressful conditions. J Bot. 2012;2012:1–26.
- Dong S, Jiang Y, Dong Y, et al. A study on soybean responses to drought stress and rehydration. Saudi J Biol Sci. 2019;26(8):2006–2017.
- Singh VP, Singh S, Kumar J, et al. Hydrogen sulfide alleviates toxic effects of arsenate in pea seedlings through up-regulation of the ascorbate-glutathione cycle: possible involvement of nitric oxide. J Plant Physiol. 2015;181:20–29.
- Apel K, Hirt H. Reactive oxygen species: metabolism, oxidative stress, and signal transduction. Annu Rev Plant Biol. 2004;55:373–399.
- Moller IM, Jensen PE, Hansson A. Oxidative modifications to cellular components in plants. Annu Rev Plant Biol. 2007;58:459–481.
- Liu Q, Shen Y, Yin K. The antimicrobial activity of protein elicitor AMEP412 against Streptomyces scabiei. World J Microbiol Biotechnol. 2020;36(1):18.
- Liu Q, Zhang B, Shen Y, et al. Effect of the protein elicitor AMEP412 from Bacillus subtilis artificially fed to adults of the whitefly, bemisiatabaci (genn.) (Hemiptera: Aleyrodidae). Egypt J Biol Pest Control. 2020;30(3):1–5.
- Foyer CH, Noctor G. Oxidant and antioxidant signaling in plants: a revaluation the concept of oxidative stress in a physiological context. Plant Cell Environ. 2005;28(8):1056–1071.
- Bian S, Jiang Y. Reactive oxygen species, antioxidant enzyme activities and gene expression patterns and recovery. Sci Hortic. 2009;120(2):264–270.
- Lamb C, Dixon RA. The oxidative burst in plant disease resistance. Annu Rev Plant Physiol Plant Mol Biol. 1997;48(1):251–275.
- Ruiz C, Nadal A, Montesinos E, et al. Novel rosaceae plant elicitor peptides as sustainable tools to control Xanthomonas arboricola pv. pruni in prunus spp. Mol Plant Pathol. 2018;19(2):418–431.
- Yu WQ, Wang X, Tang YC, et al. Cloning and expression of the EsxA gene and the growth-promoting effects of the encoded protein on rice seedlings. AMB Express. 2021;11(1):72.
- Demirkol G. PopW enhances drought stress tolerance of alfalfa via activating antioxidative enzymes, endogenous hormones, drought related genes and inhibiting senescence genes. Plant Physiol Biochem. 2021;166:540–548.
- Cheeseman JM. Hydrogen peroxide and plant stress: a challenging relationship. Plant Stress. 2007;1:4–15.
- Neill SJ, Desikan R, Clarke A, et al. Hydrogen peroxide and nitric oxide as signaling molecules in plants. J Exp Bot. 2002;53(372):1237–1247.
- Hung SH, Yu CW, Lin CH. Hydrogen peroxide functions as a stress signal in plants. Bot Bull Acad Sin. 2005;46:1–10.
- Zhang X, Zhang L, Dong F, et al. Hydrogen peroxide is involved in abscisic acid-induced stomatal closure in vicia faba. Plant Physiol. 2001;126(4):1438–1448.
- Ke D, Sun G. The effect of reactive oxygen species on ethylene production induced by osmotic stress in etiolated mungbean seedling. Plant Growth Regul. 2004;44(3):199–206.
- Kwak JM, Nguyen V, Schroeder JI. The role of reactive oxygen species in hormonal responses. Plant Physiol. 2006;141(2):323–329.
- Li F, Wang J, Ma C, et al. Glutamate receptor-like channel3.3 is involved in mediating glutathione-triggered cytosolic calcium transients, transcriptional changes, and innate immunity responses in arabidopsis. Plant Physiol. 2013;162(3):1497–1509.
- Bredow M, Monaghan J. Regulation of plant immune signaling by calcium-dependent protein kinases. Mol Plant Microbe Interact. 2019;32(1):6–19.
- Kudla J, Becker D, Grill E, et al. Advances and current challenges in calcium signaling. New Phytol. 2018;218(2):414–431.
- Ludwig AA, Saitoh H, Felix G, et al. Ethylene-mediated cross-talk between calcium-dependent protein kinase and MAPK signaling controls stress responses in plants. Proc Natl Acad Sci U S A. 2005;102(30):10736–10741.
- Yang YX, Ahammed GJ, Wu C, et al. Crosstalk among jasmonate, salicylate and ethylene signaling pathways in plant disease and immune responses. Curr Protein Pept Sci. 2015;16(5):450–461.
- Ishibashi Y, Yamaguchi H, Yuasa T, et al. Hydrogen peroxide spraying alleviates drought stress in soybean plants. J Plant Physiol. 2011;168(13):1562–1567.