Abstract
The MADS-box transcription factor family plays an important role in regulating growth and signal transduction in animals and plants. TaMADS32 encodes a type II MADS-box transcription factor which is mainly expressed in wheat root system. In this study, we used bioinformatics methods to analyse TaMADS32, and investigate its function in plant root development and response to various abiotic stresses. We mapped TaMADS32 on chromosome 2B of wheat. Both TaMADS32 and its orthologs contained 7 exons and 6 introns and all the orthologous proteins have the MADS domains. Prokaryotic experiments showed that TaMADS32 could be expressed in prokaryotic cells. The predicted subcellular localization of TaMADS32 in plant cells was in the nucleus and in the cell membrane. Abiotic stresses (drought, heat and cold) can alter the expression of TaMADS32 in transgenic Arabidopsis. Phenotypic analysis showed that overexpression of TaMADS32 enhanced the tolerance of Arabidopsis to abiotic stresses. These results indicate that TaMADS32 is probably involved in the adaptation of plants to abiotic stress environments.
Introduction
Plants must adapt to or resist various adverse environments through physiological and biochemical adjustments during their growth and development [Citation1]. Transcription factors play an irreplaceable role in the plants’ response to the changing external environment [Citation2]. The MADS-box gene family encodes transcription factors that regulate the growth and reproduction of eukaryotes and play important roles in organ development, signal transduction and material transport in animals and plants [Citation3–5]. The MADS-box genes are divided into two groups: type I and type II. Plant type II MADS domain proteins have a characteristic domain structure that includes MADS, I, K and C-terminal domains. Thus type II groups are also known as MIKC-type MADS-box genes [Citation6,Citation7]. To date, most reports about the function of MIKC-type MADS-box genes have focussed on flower development in plants [Citation8,Citation9]. For example, in wheat, TaVRT2 and TaVRN1 are flowering promoters in the vernalization pathway and jointly regulate the photosynthesis-induced flowering [Citation10].
In addition to its extensively reported roles in floral organ development, the MADS-box gene family has been increasingly reported to be involved in the regulation of abiotic stresses [Citation11,Citation12]. For example, the rice MADS-box protein OsMADS23 was up-regulated after dehydration and involved in Ca2+ dependent signal transduction further proving the role of MADS-box proteins in the cross-pathway between development and stress response [Citation13]. Many other studies have also shown that some members of the MADS-box family regulated the appearance and development of roots, and played an important role in plant response to abiotic stresses [Citation14,Citation15].
TaMADS32 (also named TraesCS2B01G344000.1) is a MIKC type MADS-box gene. Our previous study on TaMADS32 showed that it was highly expressed in the roots of ‘Chinese Spring’ and its expression level varied under different abiotic stresses [Citation16]. In this study, we characterized TaMADS32 using bioinformatics tools, prokaryotic expression, subcellular localization prediction, and expression analysis of TaMADS32 overexpressed in Arabidopsis. This study provided a foundation for understanding the function of TaMADS32 in plant growth and response to abiotic stresses.
Materials and methods
Plant materials
Seeds of Triticum aestiveum L. ‘Chinese Spring’ were sown and cultivated in Wenjiang experimental base, Chengdu, Sichuan, China. Field management was carried out according to the common practices for wheat production [Citation17]. Onion was used for subcellular localization and wild-type Arabidopsis thaliana ecotype Columbia-0 (Col-0) was used for functional analysis. Arabidopsis was grown in a growth chamber at 22 °C with 16 h light (220 µmol m−2 s−1)/8 h dark photoperiod with a relative humidity of 70%. Onions were bought from a local market.
Sequence data collection of orthologous genes
Pseudomolecules and coding sequence (CDS) of TaMADS32 in wheat genotype ‘Chinese Spring’ were downloaded from http://wheat-urgi.versailles.inra.fr/ (IWGSC RefSeq v1.0). The whole genome sequences of rice (Oryza sativa) and Brachpodium distachyon v2.0 were obtained from Phytozome v9.0 and v10 (http://www.phytozome.org/), respectively. The genome sequences and CDS of Aegilops tauschii (AetV4.0) were downloaded from https://www.ncbi.nlm.nih.gov/assembly/GCA_002575655.1/#/def_asm_Primary_Assembly. The assembled Triticum urartu genome sequences and CDS were downloaded from GIGA_DB (http://gigadb.org/) National Center for Biotechnology Information (NCBI, http://www.ncbi.nlm.nih.gov/) and GIGA_DB (http://gigadb.org/), respectively. Pseudomolecules and CDS of wild emmer (T. turgidum) were downloaded from http://202.194.139.32/.
Chromosomal locations of TaMADS32 genes
We blasted the CDS against a public database to determine the full-length genome sequence using the BLAST++ BLASTN algorithm with an E value cut-off of 10−5 [Citation18]. The gDNA sequences in each species were further blasted against their genomes to determine their positions on the chromosome.
Protein structure analysis and phylogenetic analysis
The intron-exon structures of the orthologs in different species were determined by GSDS 2.0 and DNAman 7.0 (Lynnon Biosoft, San Ramon, CA, USA) was used to evaluate the similarity between the sequences of each species. SMART (http://smart.embl-heidelberg.de/smart/set_mode.cgi?GENOMIC=1) was used for protein motif analysis [Citation19,Citation20]. Multiple alignments and drawing of a dendrogram for the evolutionary relationship in different species were performed using MEGA X [Citation21].
Analysis of cis-acting elements and transcription factor binding sites in promoters
The 1000 bp upstream sequences of these genes from wheat (T. aestiveum L.), rice (Oryza sativa L.), wild emmer (T. turgidum L.), Brachypodium distachyon L., Aegilops tauschii L. and barley (Hordeum vulgare L.) were used for searching cis-acting elements in PlantCARE database [Citation22]. We further compared the cis-acting elements of these species. In order to predict the transcription factor binding sites, we scanned the sequence in JASPAR http://jaspar.genereg.net/search?q=&collection=CORE&tax_group=plants [Citation23]. We further retrieved motifs from the footprintDB (http://floresta.eead.csic.es/footprintdb) to confirm the transcription factors [Citation24]. Correlation analysis between predictive transcription factors and expression patterns in rice, barley and wheat were calculated by SPSS Version 22.0 (SPSS Inc., Chicago, IL).
Public database-based analysis of expression pattern of TaMADS32 in wheat
The expression level of TaMADS32 (TPM, transcripts per million) in wheat was obtained from the expVIP database (http://www.wheat-expression.com/). To further verify the results, we also retrieved expression values (FPKM, fragments per kilobase of exon per million mapped reads) from WheatExp database (http://wheat.pw.usda.gov/WheatExp/).
Bacterial expression of CDS of TaMADS32
The restriction enzyme sites of vector and gene were analysed using CE design v1.04 software. BamHI and SalI restriction enzyme sites were selected and introduced into the CDS of TaMADS32. The modified CDS was cloned into the prokaryotic expression vector pET-30a (Novagen) (). The fusion vector was transformed into BL21 Escherichia coli which was purchased from Shanghai Weidi Biotechnology Co., Ltd. To induce bacterial expression, the cells were treated with 0.5 mmol/L IPTG (isopropyl β- d-1-thiogalactopyranoside) for 3-5 h, and then run on 15% sodium dodecyl-sulfate polyacrylamide gel electrophoresis (SDS-PAGE) gel [Citation25].
Subcellular localization of TaMADS32
The confirmed PCR products excluding stop codon were inserted into the pCAMBIA2300-GFP vector digested with BamHI and SalI () upstream of the green fluorescent protein (GFP) sequence [Citation26]. The recombinant plasmid (35S: GFP-TaMADS32) and empty vector (35S: GFP) were transiently introduced into the onion epidermis. PDS-1000/He particle transport system (Bio-Rad, Hercules, CA, USA) was used for the transient expression experiment. GFP signals were detected using confocal laser scanning microscopy (LSM 710; Karl Zeiss, Jena, Germany) [Citation27].
Overexpression vector construction and Arabidopsis transformation
The amplicon of TaMADS32 CDS was cloned into the plant expression vector pHB (). Recombinant plasmids were inserted into Agrobacterium tumefaciens GV3101. Transformation of Arabidopsis was performed by the floral dip method [Citation28]. Transgenic plants were screened on 1/2 Murashige and Skoog medium containing 50 mg/L hygromycin. Putative transformants were verified by real-time quantitative PCR (RT-qPCR) using qTaMADS32-F/R primers (). Overexpressing plants were screened repeatedly, and only homozygous T3 generation plants were used for stress tolerance analysis [Citation29].
Table 1. Details of primers used in this study.
Abiotic stress treatments of overexpressing Arabidopsis
The overexpressing Arabidopsis were transferred to soil in a growth chamber. Planting conditions refer to the above method. After 3 weeks, 18 Arabidopsis seedlings were used in each abiotic stress treatment. For salt stress treatment, seedlings were irrigated with 400 mmol/L sodium chloride (NaCl) solution (200 mL per pot) every 3 days for 2 weeks. For drought stress treatment, seedlings were irrigated with sufficient water for 1 week and then were kept without watering for 14 days. Seedlings for heat and cold stresses were cultured in an incubator at 42 °C and 4 °C for three days, respectively. For ABA (abscisic acid) stress, each plant was sprayed with 2 mL of 25 mg/L ABA on the leaves for 3 days.
RNA isolation and RT-qPCR analysis
We collected leaves, roots, stems, inflorescence and seeds in various stages of the bread wheat (T. aestivum L.) genotype ‘Chinese Spring’. Abiotic stress treatments including cold, ABA, NaCl, drought and cold referred to Li [Citation30]. The roots, stems and leaves of Arabidopsis seedlings in each abiotic stress treatment were collected separately for total RNA extraction. The Plant RNA Kit R6827 (Omega Bio-Tek, American) was used to extract total RNA of the collected samples. Qualified RNA was stored at −80 °C and used for reverse transcription. cDNA synthesis was performed using Takara PrimeScript TMRT reagent Kit with gDNA Eraser (Perfect Real Time, TaKaRa, Shiga, Japan). SYBR Premix Ex TaqTM II (TaKaRa, Shiga, Japan) was used for RT-qPCR. The RT-qPCR conditions were: 95 °C for 10 min, 40 cycles at 95 °C for 15 s, 60 °C for 30 s. The β-actin1 from wheat [Citation31] and β-actin2 from Arabidopsis (GenBank Accession No. NM_001338359.1) were, respectively, used as the internal reference genes in wheat and Arabidopsis RT-qPCR analysis. The relative transcription abundance was calculated using the 2 - Δ Δ CT method [Citation32]. The mean value of the three biological experiments was used for the analysis. The primer sequences are shown in .
Results
Chromosomal locations and structures analysis of TaMADS32 and orthologs
The full-length sequence of TaMADS32 was 13,999 bp (TraesCS2B01G344000.1), and its homologs were 13,218 bp (TraesCS2A01G337900.1), and 14,820 bp (TraesCS2D01G325000.1), respectively. Blasting results showed that TaMADS32 was a single-copy gene, and its orthologs in rice, T. urartu, wild emmer, B. distachyon, Ae, tauschii, and barley were also single-copy genes. The three homologous sequences of TaMADS32 were located on 2AL, 2BL and 2DL, respectively (). The ortholog in rice (Os02g36924.1) was mapped to the chromosome arm 2 L, that in Ae. tauschii (AET2Gv20737400.2) on 2DL, those of wild emmer (TRIDC2AG047600.1, TRIDC2BG05100.4) on 2AL and 2BL. Due to the incomplete sequence of TRIDC2BG05100.4 in wild emmer wheat, only TRIDC2AG047600.1 was analysed in the subsequent analysis. The gene and protein structure analysis showed that all orthologs of TaMADS32 from different species contained 7 exons and 6 introns () and contained a highly conserved MADS domain in all the studied species. Two coiled helix configurations were detected in wheat, wild emmer and Ae. tauschii, while only one existed in other species (). The phylogenetic analysis showed that the orthologs of T. aestivum, Ae. tauschii and wild emmer were clustered into a single group. Among these orthologs, TaMADS32 exhibited the closest genetic relationship with TraesCS2A01G337900.1 in T. aestivum, and TRIDC2AG047600.1 in wild emmer, followed by Ae. tauschii, and the farthest relationship with T. urartu ().
Promoter analyses
Based on the prediction of transcription factor binding sites, we found three common matrixes, ABI3, abi4 and AG () in wheat (TraesCS2A01G337900.1, TraesCS2B01G344000.1 and TraesCS2D01G325000.1), barley (HORVU2Hr1G080490.1) and rice (Os02g36924.1). These detected motifs were verified on footprintdb (http://floresta.eead.csic.es/footprintdb).
Table 2. Putative transcription factors of promoters in wheat, barley and rice.
Expression pattern of TaMADS32 in ‘Chinese Spring’
The results from a public database showed that TaMADS32 was expressed in various tissues of wheat at different growth stages. Data from expVIP showed that TaMADS32 was mainly expressed in the root (root_ z10, root_ z13, root_ z39), and less abundantly expressed in leaves and spikes (spike_ z39, spike_ Z65) (). Data from WheatExp also showed that its expression level was mainly in the root (root _ z13, root_ z39) followed by the spikes (). RT-qPCR results also showed that the expression of TaMADS32 was mainly expressed in roots ().
Figure 3. Expression patterns of TaMADS32 in wheat. Gene expression pattern at different periods in the exp VIP database, Zadok’s wheat growth phases (a). The gene expression pattern in the Wheat exp database (b). RT-qPCR validation results from different tissues at different periods (c). Three biological replicates were used.
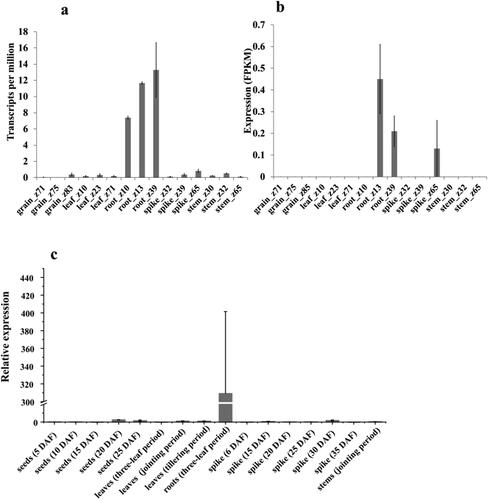
We analysed the TaMADS32 expression under stress conditions on the expression database (expVIP and WheatExp). In wheat, polyethylene glycol (2 h and 12 h) treatment increased the expression of TaMADS32. After cold treatment for two weeks, the expression of TaMADS32 was decreased (). A combination of drought and heat treatment for 1 h decreased TaMADS32 gene expression but increased at 6 h. Heat treatment for 1 h decreased significantly TaMADS32 expression but increased it at 6 h. Under drought treatment, the expression of TaMADS32 was slightly decreased at 1 h but was up-regulated at 6 h (). We used RT-qPCR to verify the results obtained from the databases. The results showed that the expression of TaMADS32 was significantly decreased in roots under 4 h and 12 h of cold treatment, but was firstly decreased and then up-regulated in leaves. The expression of TaMADS32 in leaves and roots was both significantly down-regulated at 4 h and 12 h of ABA treatment. Under NaCl treatment (NaCl 4 h and 10 h), the expression of TaMADS32 in leaves and roots was also significantly down-regulated. Under heat treatment, the expression of TaMADS32 was significantly decreased in leaves only at 4 h, inversely only decreased in roots at 12 h ().
Figure 4. Expression patterns of the TaMADS32 gene under various abiotic stresses. Gene expression patterns under stresses (PEG, cold, drought and heat stresses) in the expVIP database, the seedlings of 9 days were treated with drought and three-leaf seedlings were treated with cold (a,b). RT-qPCR validation results; DAF, days after flowering (c). Three biological replicates. Note: * Significant at the 0.05 probability level, **Significant at the 0.01 probability level.
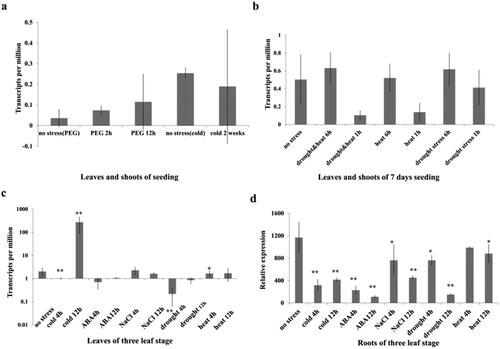
Expression of TaMADS32 in E. coli
To verify whether TaMADS32 can be expressed in other species, we recombined its CDS into pET-30a and transferred it into E. coli, and then induced its expression with IPTG. SDS-PAGE protein analysis showed that compared with the E. coli with empty vectors, a new protein band appeared at about 37 kD in the E. coli with the recombinant vectors. Among the protein bands, the 10-kD protein was expressed by the pET-30a, and a protein of about 27-kD was expressed by the TaMADS32 recombinant cells, and the result was consistent with the target protein size encoded by TaMADS32. pET30a-TaMADS32, which was not induced by IPTG, showed a small amount of expression, and no expression was shown in the E. coli cells with empty vectors (). These results indicate that TaMADS32 can express the corresponding protein in prokaryotic cells. Thus we could further carry out subcellular localization and overexpression experiments.
TaMADS32 is localized in the cell membrane and nucleus
TaMADS32 was predicted to be a nuclear protein with a MADS-box domain. To confirm the subcellular localization of TaMADS32, we constructed pCAMBIA2300-GFP-TaMADS32 fusion protein and empty vector (pCAMBIA2300-GFP). They were then transiently co-transformed into onion cells. Green fluorescence from pCAMBIA2300-GFP was localized in the cell membrane, cytoplasm and nucleus. However, the green fluorescence signal of the fusion protein was mostly merged with the cell membrane and nucleus further indicating that TaMADS32 was located in the nucleus and cell membrane ().
Overexpression of TaMADS32 improves the tolerance of Arabidopsis to stresses
The overexpressing T1 Arabidopsis seedlings were selected using the medium containing 50 mg/L hygromycin. The results of RT-qPCR and DNA gel blot analysis showed that TaMADS32 was transferred into Arabidopsis and could be expressed successfully (). To further clarify the function of TaMADS32, we studied the overexpressing Arabidopsis in different abiotic stresses including cold, ABA, NaCl, drought and heat (). RT-qPCR results showed that under normal growth conditions, TaMADS32 was primarily detected in roots and leaves. Besides, we found that the transcriptional level of TaMADS32 in the overexpressing lines under different abiotic stresses was different. Under heat stress, the expression of TaMADS32 in the roots was significantly increased, but the expression in the stems and leaves was reduced significantly compared to that under normal conditions. Under drought treatment, the expression of TaMADS32 in the root was similar with that under normal growth conditions, but its expression in the stems and leaves was dramatically reduced. Under NaCl treatment, the expression of TaMADS32 in roots, stems and leaves was decreased significantly compared to that under normal conditions. Under ABA treatment, the expression of TaMADS32 in the roots, stems and leaves of the overexpressing plants was significantly inhibited. Under cold treatment, the expression of TaMADS32 in the roots was significantly inhibited, but there was no significant difference in the stems and leaves ().
Figure 7. Expression analysis of TaMADS32 in wild-type and transgenic Arabidopsis plants. Relative expression in Arabidopsis plants (a); WT, the wild-type plant; L, the transgenic Arabidopsis. RT-PCR analysis of TaMADS32 gene and Actin in Arabidopsis. M, Marker, L1, L2, transgenic Arabidopsis lines, WT, wild-type Arabidopsis (b); expression patterns of the gene in transgenic Arabidopsis by RT-qPCR in normal conditions, cold, drought, ABA, heat and NaCl stress (c). The expression levels are normalized to Actin with three biological replicates. Note: CK means no stress, * Significance at the 0.05 probability level, **Significance at the 0.01 probability level.
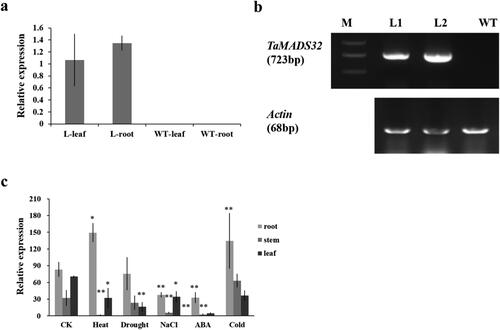
Discussion
MIKC-type MADS-box genes have been proved to play important roles in the formation and regulation of protein complexes, and are becoming important targets in studying the process of plant breeding and improvement [Citation33–35]. There are 180 MADS-box genes in wheat, including 138 MIKC-type MADS-box genes [Citation16]. Our study showed that TaMADS32 gene is a MIKC-type MADS-box gene, and it has all the characteristics of the MIKC-type MADS-box genes. These conserved structural regions of the MIKC-type MADS-box genes suggest that they may have new functions in response to a variety of biotic and abiotic stresses [Citation34]. Most MADS-box proteins can bind to DNA elements in SRE type CArG-boxes (CC[A/T]6GG)’ and regulate their own expression [Citation7]. The results of protein structure analysis showed that all the investigated genes have a highly conserved MADS-box domain (). The MADS-box domain regulates the spatiotemporal expression of genes and mediates many important biological processes in plants. For example, WFUL1 and WFUL2 were expressed in the base of the spikelet meristem, while WFUL3 was expressed in both the spikelet primordium and the whole spikelet meristem [Citation36,Citation37]. Overexpression of WFUL1 and WFUL2 leads to an early flowering phenotype. WFUL1 has the function of providing flowering capacity, while WFUL3 plays a role in flower meristem development together with WFUL2 [Citation37–39]. Therefore, as a member of MIKC type MADS-box genes, whether TaMADS32 can regulate the specific expression of other genes and participate in biological functions remains to be studied.
According to the current research progress about the MADS-box family in wheat, it is necessary to further study how the expression of MADS-box genes respond to various environmental stresses in plants [Citation34]. Based on previous studies [Citation16], we found that the transcription level of TaMADS32 was down-regulated significantly under ABA, NaCl, drought and heat treatment, indicating that it may be involved in the response to these stresses (). We thus transferred it into Arabidopsis and treated the wild-type and overexpressing Arabidopsis with abiotic stresses. We found that under NaCl and ABA treatments, the expression of TaMADS32 was inhibited in the overexpressing Arabidopsis suggesting that the exogenous NaCl and ABA may inhibit the expression of TAMADS32 in the roots of overexpressing Arabidopsis and thus form effective response mechanisms to resist or adapt to NaCl and ABA stresses. The expression of TaMADS32 in ‘Chinese Spring’ and overexpressing Arabidopsis was similar, indicating that TaMADS32 has a certain conserved function in different species. TaMADS32 was up-regulated in the roots of Arabidopsis under cold and heat stress. We speculated that increasing the transcription level of TAMADS32 could improve the resistance of plants to these abiotic stresses. Overall, these results suggested that TAMADS32 may have some important roles in regulation of stresses response and has breeding potentials in wheat.
Conclusions
In this study, we characterized the root-related gene TaMADS32 (TraesCS2B01G344000.1) using bioinformatics methods and molecular biology techniques. TaMADS32 can be expressed in prokaryotic bacteria and was localized in the cell membrane and nucleus of plant cells. The expression of TaMADS32 was up-regulated in the roots of overexpressing Arabidopsis plants under heat and cold stresses, compared to that under normal conditions. The expression of TaMADS32 was decreased under NaCl and ABA stresses. These results can contribute to our understanding of the regulation mechanism of the TaMADS32 gene under different abiotic stresses.
Authors’ contributions
XMZ and TL finished the study and wrote this manuscript. HXC participated in field work and analysed data. HPT and YM did field work and helped with informatics analysis. LLG and AH helped with data collection, data analysis and discussion revision. XJL analysed data, discussed results and revised the manuscript. JM designed the experiments, guided the entire study, participated in data analysis and extensively revised this manuscript. All authors participated in the research and approved the final manuscript.
Data availability statement
All data generated or analysed during this study are included in this article; further inquiries can be directed to the corresponding author upon reasonable request.
Disclosure statement
No potential conflict of interest was reported by the authors.
Correction statement
This article has been republished with minor changes. These changes do not impact the academic content of the article.
Additional information
Funding
References
- Wang M, Zhuang J, Zou Z, et al. Overexpression of a Camellia sinensis DREB transcription factor gene (CsDREB) increases salt and drought tolerance in transgenic Arabidopsis thaliana. J Plant Biol. 2017;60(5):452–461.
- Agarwal P, Jha B. Transcription factors in plants and ABA dependent and independent abiotic stress signalling. Biologia Plant. 2010;54(2):201–212.
- Bai G, Yang DH, Cao P, et al. Genome-wide identification, gene structure and expression analysis of the MADS-Box gene family indicate their function in the development of tobacco (Nicotiana tabacum L.). Int J Mol Sci. 2019;20(20):5043.
- Messenguy F, Dubois E. Role of MADS box proteins and their cofactors in combinatorial control of gene expression and cell development. Gene. 2003;316:1–21.
- Ming X, Tao YB, Fu Q, et al. Flower-specific overproduction of cytokinins altered flower development and sex expression in the perennial woody plant Jatropha curcas L. Int J Mol Sci. 2020;21(2):640.
- Alvarez-Buylla ER, Pelaz S, Liljegren SJ, et al. An ancestral MADS-box gene duplication occurred before the divergence of plants and animals. Proc Natl Acad Sci U S A. 2000;97(10):5328–5333.
- Kaufmann K, Melzer R, Theissen G. MIKC-type MADS-domain proteins: structural modularity, protein interactions and network evolution in land plants. Gene. 2005;347(2):183–198.
- Becker A, Theissen G. The major clades of MADS-box genes and their role in the development and evolution of flowering plants. Mol Phylogenet Evol. 2003;29(3):464–489.
- Hugouvieux V, Silva CS, Jourdain A, et al. Tetramerization of MADS family transcription factors SEPALLATA3 and AGAMOUS is required for floral meristem determinacy in arabidopsis. Nucleic Acids Res. 2018;46(10):4966–4977.
- Xie L, Zhang Y, Wang K, et al. TaVrt2, an SVP-like gene, cooperates with TaVrn1 to regulate vernalization-induced flowering in wheat. New Phytol. 2021;231(2):834–848.
- Chen L, Zhao Y, Xu S, et al. OsMADS57 together with OsTB1 coordinates transcription of its target OsWRKY94 and D14 to switch its organogenesis to defense for cold adaptation in rice. New Phytol. 2018;218(1):219–231.
- Dong TT, Song WH, Tan CT, et al. Molecular characterization of nine sweet potato (Ipomoea batatas Lam.) MADS-box transcription factors during storage root development and following abiotic stress. Plant Breed. 2018;137(5):790–804.
- Rai Y, Wardhan V, Gupta DB, et al. Calcium-dependent changes in physicochemical properties and the proteome dynamics influence dehydration responses in rice. Env Exp Bot. 2020;172:103965.
- Montiel G, Gaudet M, Laurans F, et al. Overexpression of MADS-box gene AGAMOUS-LIKE 12 activates root development in Juglans sp. and Arabidopsis thaliana. Plants (Basel. 2020;9(4):444.
- Yu C, Liu Y, Zhang A, et al. MADS-box transcription factor OsMADS25 regulates root development through affection of nitrate accumulation in rice. PLoS One. 2015;10(8):e0135196.
- Ma J, Yang Y, Luo W, et al. Genome-wide identification and analysis of the MADS-box gene family in bread wheat (Triticum aestivum L.). PLoS One. 2017;12(7):e0181443.
- Liu J, Luo W, Qin N, et al. A 55 K SNP array-based genetic map and its utilization in QTL mapping for productive tiller number in common wheat. Theor Appl Genet. 2018;131(11):2439–2450.
- Ma J, Stiller J, Berkman PJ, et al. Sequence-based analysis of translocations and inversions in bread wheat (Triticum aestivum L.). PLoS One. 2013;8(11):e79329.
- Letunic I, Doerks T, Bork P. SMART: recent updates, new developments and status in 2015. Nucleic Acids Res. 2015;43:D257–D260.
- Tamura K, Stecher G, Peterson D, et al. MEGA6: Molecular evolutionary genetics analysis version 6.0. Mol Biol Evol. 2013;30(12):2725–2729.
- Kumar S, Stecher G, Li M, et al. MEGA X: Molecular evolutionary genetics analysis across computing platforms. Mol Biol Evol. 2018;35(6):1547–1549.
- Lescot M, Déhais P, Thijs G, et al. PlantCARE, a database of plant cis-acting regulatory elements and a portal to tools for in silico analysis of promoter sequences. Nucleic Acids Res. 2002;30(1):325–327.
- Khan A, Fornes O, Stigliani A, et al. JASPAR 2018: update of the open-access database of transcription factor binding profiles and its web framework. Nucleic Acids Res. 2018;46(D1):D260–D266.
- Contreras-Moreira B, Sebastian A. FootprintDB: analysis of plant cis-regulatory elements, transcription factors, and binding interfaces. Methods Mol Biol. 2016;1482:259–277.
- Santambrogio P, Levi S, Cozzi A, et al. Production and characterization of recombinant heteropolymers of human ferritin H and L chains. J Biol Chem. 1993;268(17):12744–12748.
- Wang W, Wang Y, Du Y, et al. Overexpression of Camellia sinensis H1 histone gene confers abiotic stress tolerance in transgenic tobacco. Plant Cell Rep. 2014;33(11):1829–1841.
- Li P, Song A, Gao C, et al. Chrysanthemum WRKY gene CmWRKY17 negatively regulates salt stress tolerance in transgenic chrysanthemum and arabidopsis plants. Plant Cell Rep. 2015;34(8):1365–1378.
- Clough SJ, Bent AF. Floral dip: a simplified method for agrobacterium-mediated transformation of Arabidopsis thaliana. Plant J. 1998;16(6):735–743.
- Gao Z, He X, Zhao B, et al. Overexpressing a putative aquaporin gene from wheat, TaNIP, enhances salt tolerance in transgenic arabidopsis. Plant Cell Physiol. 2010;51(5):767–775.
- Li M. Isolation and functional analysis of drought stress responsive gene TaWNK in wheat [D]. Yangling, China: Northwest A&F University; 2018.
- Zhang H, Ma J, Liu J, et al. Molecular characterization of the TaWTG1 in bread wheat (Triticum aestivum L.). Gene. 2018;678:23–632.
- Zhang CC, Yuan WY, Zhang QF. RPL1, a gene involved in epigenetic processes regulates phenotypic plasticity in rice. Mol Plant. 2012;5(2):482–493.
- Gramzow L, Theissen G. A hitchhiker’s guide to the MADS world of plants. Genome Biol. 2010;11(6):214.
- Schilling S, Kennedy A, Pan S, et al. Genome-wide analysis of MIKC-type MADS-box genes in wheat: pervasive duplications, functional conservation and putative neofunctionalization. New Phytol. 2020;225(1):511–529.
- Schilling S, Pan S, Kennedy A, et al. MADS-box genes and crop domestication: the jack of all traits. J Exp Bot. 2018;69(7):1447–1469.
- Kinjo H, Shitsukawa N, Takumi S, et al. Diversification of three APETALA1/FRUITFULL-like genes in wheat. Mol Genet Genomics. 2012;287(4):283–294.
- Murai K. Homeotic genes and the ABCDE model for floral organ formation in wheat. Plants (Basel). 2013;2(3):379–395.
- Adam H, Ouellet F, Kane NA, et al. Overexpression of TaVRN1 in arabidopsis promotes early flowering and alters development. Plant Cell Physiol. 2007;48(8):1192–1206.
- Callens C, Tucker MR, Zhang D, et al. Dissecting the role of MADS-box genes in monocot floral development and diversity. J Exp Bot. 2018;69(10):2435–2459.