Abstract
Sucrose transporters (SUCs/SUTs) play crucial roles in apoplast transport and long-distance distribution of sucrose throughout the whole plant. However, whether and how the Arabidopsis AtSUC4 modulates sucrose import from apoplast to cytosol remains unclear. In the present study, we found that AtSUC4 protein was localized to the plasma membrane in the root. Expression of AtSUC4 in roots was gradually induced with the increasing sucrose concentrations (0%, 2%, 4% and 6%). When feeding high concentrations (4% and 6%) of sucrose, the primary root growth of seedling was inhibited. Interestingly, atsuc4 mutants exhibited longer primary root than the wild type under these conditions, indicating that atsuc4 mutants were less sensitive to excess sucrose. Moreover, the root of atsuc4 mutants accumulated less sucrose and abscisic acid (ABA) and more indole-3-acetic acid (IAA) on 4% and 6% sucrose supplementation. Transcriptomic analysis revealed that numerous genes associated with sugar transport and metabolism, as well as ABA signalling were down-regulated, whereas many IAA signaling-related genes were up-regulated in mutant plants relative to the wild type under 6% sucrose treatment. Collectively, our finding demonstrated that the deficiency of AtSUC4 reduced the inhibition of primary root growth under high sucrose condition, probably through reducing the sucrose transportation and metabolism, and subsequent alteration in IAA and ABA signalling.
Keywords:
Introduction
Sucrose, functioning as a nutritional substance, osmolyte and signal molecule, plays a central role in the response and adaptation to environmental stress in most plants [Citation1]. Most sucrose is derived from the photosynthetic source organ, the leaves, and transported into sink organs, including flowers, stems, roots and seeds. This involves the uptake or release of sucrose from cells or subcellular compartments via transport proteins. Thus, not surprisingly, sucrose transporters (SUCs or SUTs) are critical for plant growth and development [Citation2]. Sucrose is synthesized in the source cells and exported to the apoplasm probably by the SWEET efflux proteins, then imported into the sieve elements and companion cell complexes (SE-CCCs) via sucrose transporters (SUTs or SUCs) in most plants [Citation3]. Studies have detected that the concentration of sucrose could reach 10−1 mol L−1 to 1 mol L−1 in the conducting vascular cells, while extracellular sucrose concentrations are in the 10−3 mol L−1 range [Citation4,Citation5]. However, the mechanism of how these cells respond to such higher sucrose concentrations to maintain the sucrose equilibrium in extra- and intra-cellular components is still unknown.
SUCs are H+/sucrose symporters that utilize the proton motive force present across the plasma membrane (PM) of the SE-CCCs to load sucrose against its concentration gradient into the phloem [Citation6]. Multiple SUT genes have been identified and initially classified into three subgroups (SUT1, SUT2 and SUT4 subfamily) but later into four distinct groups (Group I, II, III and IV) according to phylogenetic analysis [Citation7,Citation8]. Among them, most members of Group II are high-affinity/low-capacity sucrose transporters with Km values in the range of 139 μmol L−1−1.5 mmol L−1 and described as PM-localized transporters, whereas members of the Group IV (with the exception of OsSUT2 from rice) are low-affinity/high-capacity sucrose transporters with Km values ranging from 5 mmol L−1 to 6 mmol L−1 and suggested as the tonoplast-localized transporters [Citation9]. There are nine SUCs in Arabidopsis (Arabidopsis thaliana), among which only AtSUC4 belong to Group IV. Numerous studies showed that SUTs in Group IV have distinct functions in different plant species, indicating a versatile role of the members in this group in plant growth and development [Citation10,Citation11]. AtSUC4 can also catalyse the transport of sucrose from the vacuolar lumen into the cytoplasm in the cells [Citation12]. Due to the low expression level of AtSUC4, the aerial part of atsuc4.1 (cs856419) mutant behaved essentially as the wild type (WT) in sucrose content, germination, development and response to cold treatment when cotyledons were fully unfolded. The AtSUC4-overexpressing lines showed a 30% reduction in sucrose content in aerial parts compared with WT under 3% sucrose treatment [Citation13], implying that AtSUC4 releases sucrose from the vacuole for cellular metabolism. However, little is known about whether and how AtSUC4 participate in the sucrose import under higher sucrose status.
In Arabidopsis, the primary root elongation rate is basically dependent on the sugar concentration in the medium, and a good correlation between the elongation rate and sucrose content (0–2%) in primary roots was found [Citation14]. In addition, sugars transported into the root also transmit as signals. In young developing seedling root of Arabidopsis, sucrose transported into the root can act as a necessary and sufficient signal regulating root elongation together with light [Citation15]. The expression patterns of AtSUC1 and AtSUC2 displayed contrasting roles during the night, showing increased transcript accumulation of AtSUC2 (sucrose loading in phloem) in leaves and AtSUC1 (sucrose unloading) in roots [Citation16,Citation17]. However, the function of AtSUC4 in root elongation was uncertain, and a clear linking on the interaction between sucrose transport and root growth remains to be elucidated.
Apart from sucrose, multiple plant hormones also regulate root elongation, among which auxin stands out as a key instructive signal [Citation18]. Exogenous sucrose could promote the root growth through sugar-hormone crosstalk in Arabidopsis and peach [Citation19,Citation20]. Specifically, exogenous sucrose increased the expression of auxin synthesis- and transport-related genes in roots, resulting in auxin accumulation in the root system [Citation20]. Abscisic acid (ABA) signalling also plays a critical role in regulating root elongation and root system architecture [Citation21]. Yu et al. [Citation22] revealed that OsERF2 was required for the root architecture and ABA-response by regulating the expression of some genes involved in sugar metabolism and hormone signalling pathways. Additionally, excessive exogenous glucose inhibits primary root growth via ABI5, which represses PIN1 accumulation and auxin activity in Arabidopsis [Citation23]. To date, however, the molecular mechanism of crosstalk among sucrose transporters, indole-3-acetic acid (IAA) and ABA regulating root growth remains unclear.
To gain more information about sucrose transport regulation in root with emphasis on AtSUC4 while extracellular sucrose concentration is high, expression patterns and subcellular localization of AtSUC4 were performed first. Several atsuc4 mutant lines were generated and showed longer root length under high concentrations (4% and 6%) of exogenous sucrose. The contents of sugars and hormones (IAA and ABA) in the roots in WT and mutant lines were analysed. Transcriptome analysis was carried out and indicated that the expression of genes involved in sucrose transport and metabolism, IAA- and ABA signalling were changed in mutant lines. This study confirmed the novel function of AtSUC4 and provided insights into the regulation of AtSUC4 on root elongation under higher sucrose.
Materials and methods
Strains materials and growth conditions
Arabidopsis thaliana (ecotype Col-0) and atsuc4 mutants were grown in a growth chamber in soil under long-day (LD) conditions (16 h light/8 h dark) at 22 °C and 70% relative humidity, light was present at 100–120 μmol.m−2.s−1. Before germination, plates were incubated at 4 °C for 2 d in the dark and subsequently transferred to the growth chamber. Escherichia coli strain DH5α was used for all cloning steps. Transformation of Arabidopsis was performed using Agrobacterium tumefaciens strain GV3101 [Citation24].
Genes expression level detection
For gene expression analysis, total RNA was isolated from roots of different plants using the Plant RNA Extraction Kit (Omega, Norcross, GA, USA), and cDNA was synthesized using HiScript II 1st Strand cDNA Synthesis Kit (+gDNA wiper) (Vazyme). Reverse transcription-quantitative real time polymerase chain reaction (RT-qPCR) was conducted using FastStart DNA Master SYBR Green I (Roche) on the Roche LightCycler 480 System (Roche Applied Science), with ACTIN2 as the internal control [Citation25]. Three independent biological replicates and four technical replicates were performed. The primer sequences are listed in Supplemental Data SCitation1.
Subcellular localization assay of AtSUC4
The subcellular localization of AtSUC4 was detected by three methods. For the method of Arabidopsis stable transformation system, the promoter (2000 bp upstream of ATG) and gDNA sequence of AtSUC4, named gAtSUC4, were amplified and cloned into a pCAMBIA1300 vector. As a result, the construct pCAMBIA1300-pSUC4::gAtSUC4-GFP was generated and subsequently transformed into WT plants. The localization of AtSUC4 in root was detected. The second method used the transient expression system of Arabidopsis protoplast to check the subcellular localization of AtSUC4. Protoplast isolation and transformation of a rosette leaf were performed according to Robert et al. [Citation26]. The 35S promoter and cDNA sequence of AtSUC4 were amplified and cloned into pEarley101 vector, the vector pEarley101-35S::cAtSUC4-GFP was generated and used for protoplast transient transformation. All the fluorescence was detected using a Leica TCS SP5 confocal microscope. The excitation wavelength was 488 nm and the emitted fluorescence was recorded from 500 to 550 nm for green fluorescent protein (GFP). The third method was Immunogold labelling of AtSUC4, which was essentially performed as described previously [Citation27] using the mouse anti-AtSUC4 monoclonal antibody (Beijing Protein Innovation, Beijing, China). We selected ‘ASLASEAHGQTSGTDEAFL’ as the synthesized target peptide. Four SPF Balb/c female mice were immunized for the first time with ‘AtSUC4-KLH’. Before fusion, we used ‘AtSUC4-KLH’ to shock immunize a mouse with the best physiological state. The fused cells were transferred to semi-solid medium for culture. Then, the single clones grown on the semi-solid medium were picked into a 96-well culture plate for cultivation and subsequent screening. Subsequently, the plates were coated with ‘AtSUC4-BSA’, and the selected clones were screened by enzyme-linked immunosorbent assay (ELISA) for the first time, yielding 24 positive hybridoma cell lines. These positive cell lines were screened for the second time by ELISA, and the positive cell lines were screened for subclass identification. As a result, nine IgG-type positive hybridoma cell lines were obtained and frozen. Finally, the monoclonal antibody was used to detect the total protein of Arabidopsis by Western blot.
Verification of expression pattern of AtSUC4 at tissue level
To verify the expression pattern of AtSUC4 during Arabidopsis development at the tissue level, we created pAtSUC4::GUS transgenic plants under the control of the endogenous AtSUC4 promoter. A 998-bp fragment before the coding region of AtSUC4 was amplified from total DNA of leaf, subcloned into the entry vector pDONR207 and inserted upstream of the GUS into the destination vector pHGWFS7 [Citation28], yielding construct pHGWFS7-pAtSUC4::GUS, which was used for A. tumefaciens transformation. Primer pairs used are shown in Supplemental Data SCitation1. Samples including the whole seedlings (grown for 4 d, 14 d and 25 d period), flowers (grown for 35 d period), siliques and seeds (grown for 50 d period) were stained according to a standard protocol [Citation13]. For each sample, we analysed 10–15 independent lines, and representative results are presented. Photographs were captured by a stereomicroscope (Leica MZFL III; Leica Microsystems, Bensheim, Germany).
Generation of Arabidopsis atsuc4 mutant lines
Three types of atsuc4 mutant lines were used in this study. The atsuc4 mutant (cs93419) was obtained from the Nottingham Stock Centre (http://arabidopsis.info/) and was screened for the AtSUC4 point mutation homozygous with no ERECTA gene mutation, which was named as suc4-1. To generate an AtSUC4 knockout line suc4-2, we adopted the CRISPR/Cas9 genome editing system provided by Ma et al. [Citation29]. Two gene-specific gRNA sequences (TCACAGAGTCACTCGCAACC and CTCGTTGGGCATAGTAGCGA) were designed using the tools at http://skl.scau.edu.cn/. The AtSUC4 T-DNA insertion line named suc4-3 (WiscDsLox450E10) was obtained from the Nottingham Stock Centre (http://arabidopsis.info/). The position of the T-DNA insertion was determined by sequencing a PCR product obtained from suc4-3 genomic DNA [Citation13]. For the AtSUC4 complement-expressors (marked as AtSUC4/suc4-1), the AtSUC4 promoter and gDNA sequence fragments were amplified from the total DNA of leaves, and inserted into the BamH I- and EcoR I-digested plant transformation vector pCAMBIA1300 [Citation30] by ClonExpress MultiS One Step Cloning Kit (Vazyme). The resulting plasmid pCAMBIA1300-pSUC4::gSUC4-GFP was used for suc4-1 mutant transformation. Primer pairs for vector construction and identification of atsuc4 mutants are shown in Supplemental Data SCitation1.
Measurement of root length, plant height and the number of rosette leaves
For sugars treatment, the seeds of WT and atsuc4 mutant lines were sown on Murashige and Skoog (MS) solid media containing different concentrations of sucrose, glucose and mannitol after sterilizing the seeds (0%, 2%, 4% and 6%). Bright-field images of different plants were captured at 6 d after sowing using a Canon 60 D camera. Root length was measured by Image J software. For the phenotype comparison between WT and mutants, plants were grown in soil under LD condition. The number of rosette leaves was recorded at 25 d, and the plant height was measured at 50 d. Three biological replicates and more than 30 plants for each replicate were used for analysis.
Analysis of carbohydrate content
Preparation of plant materials and determination of carbohydrate content via ion chromatography was performed as previously described [Citation25]. WT, atsuc4 mutants and AtSUC4/suc4-1 seeds were sown on MS solid media containing different concentrations of sucrose (0%, 2%, 4% and 6%). Roots were collected and quickly grinded in liquid nitrogen. An aliquot of each individual sample was precisely weighed (20 mg) and transferred to an Eppendorf tube. After adding the addition of 500 μL of extract solvent (ethanol–water, 8:1) and including the isotope as internal stand with the fix concentration. The samples were vortexed sonicated. The homogenate and sonicate circle were repeated three times, followed by incubation at 90 °C for 30 min and centrifugation at 18,500 g for 15 min. The supernatant was transferred to an auto-sampler vial for ultra-high-performance liquid chromatography-tandem mass spectrometry (UHPLC-MS/MS) analysis to measure glucose and fructose. Another aliquot was further diluted 10 times for UPLC-MS/MS analysis to quantitate the sucrose. When the concentrations of metabolites were beyond the standard curve range, the samples were diluted accordingly to place their concentrations within the range. The final concentration was in μg mg−1. FW equals the calculated concentration multiplied by the dilution factor.
Determination of IAA and ABA content in roots
Roots were collected from WT and suc4-1 mutant grown on MS solid media with 2% and 6% sucrose supplementation under LD condition at 6 d after sowing, with three biological replicates (approximately 0.5 g fresh weight per sample). And the contents of endogenous IAA and ABA were determined according to a previously reported method with modification [Citation31]. The samples (50 mg) were analysed using Thermo Scientific Ultimate 3000 UHPLC System equipped with a Thermo Scientific TSQ Quantiva-Stage Quandrupole Mass Spectrometer (http://www.greenswordcreation.com).
Analysis of the RNA-seq data
Total RNA was isolated from the 6-d-old seedling roots of WT and suc4-1 plants grown on MS solid media containing different concentrations of sucrose (2%, 4% and 6%) in three biological replicates. As previously described [Citation32], RNA samples were treated with DNase before being quality checked using an Agilent 2100 Bioanalyzer. Total RNA was prepared for a strand-specific TruSeq™ RNA-seq library, and all 27 samples were sequenced on an Illumina HiSeq 4000, with 150 bp paired end reads. Differential expression analysis was performed using the DEGSeq R package (1.12.0). P-values were adjusted using the Benjamini & Hochberg method. A corrected P-value of 0.05 and log2 (Fold Change) of 1 were set as the threshold for significantly differential expression. Gene ontology enrichment analysis of differentially expressed genes (DEGs) was implemented by the GOseq R package, in which gene length bias was corrected. Gene ontology terms with a corrected P-value less than 0.05 were considered significantly enriched by DEGs. All sequences generated in this study have been deposited in the National Center for Biotechnology Information Sequence Read Archive (https://www.ncbi.nlm.nih.gov/sra/) with project number PRJNA666009.
Statistical analyses
The overall data were statistically analysed using SPSS 20 software (IBM China Company Ltd., Beijing, China). One-way or two-way analysis of variance with Duncan test at the 5% level was used to test differences between multiple samples. Data were presented as means and SEs or SDs using Microsoft Excel.
Results
Expression pattern and subcellular localization of AtSUC4
To investigate whether the AtSUC4 expression was induced by sucrose, we generated a construct (pAtSUC4::GUS) harbouring the GUS reporter gene driven by the endogenous AtSUC4 promoter and transformed into Col-0. pAtSUC4::GUS seedlings were grown on the medium with different concentrations of sucrose (0%, 2%, 4% and 6%) for 7 d and then stained for GUS activity detection. As shown in , with the increase in sucrose concentration in the medium, the activity of GUS became gradually higher, suggesting that AtSUC4 expression was significantly induced by sucrose. This result was further supported by GUS expression analysis using RT-qPCR (). We also detected the expression level of AtSUC4 in the root of 7-d-old WT seedlings. The result showed that the expression level of AtSUC4 increased significantly as the concentration of external sucrose increased (). These results indicated that AtSUC4 expression was induced by sucrose.
Figure 1. Expression level of AtSUC4 in response to different concentrations of sucrose. (A) 7 d-old pAtSUC4::GUS seedling on MS solid media with different concentrations of sucrose (0%, 2%, 4% and 6%). (B) The relative expression level of GUS in roots of pAtSUC4::GUS plants. (C) The relative expression level of AtSUC4 in roots of 7-d-old WT seedlings under different concentrations of sucrose treatment (0%, 2%, 4% and 6%). Data are mean values ± SE of three biological replicates. Bars with different letters indicate significant differences among treatments for the same stage according to Duncan’s test (at p < 0.05).
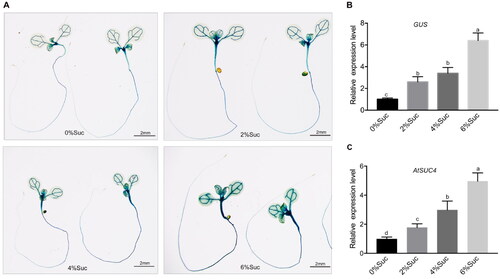
We next detected the spatio-temporal expression of AtSUC4 in Arabidopsis using pAtSUC4::GUS seedlings. As shown in Supplemental Figures S1A and B, AtSUC4 was mainly expressed in vasculature cells of leaves and mature portion of roots in 4-d- and 14-d-old seedlings. In 25-d-old plants, GUS staining covered the entire root and juvenile leaves but not mature leaves (rosette) (Supplemental Figure S1C). The inflorescence, petals, styles and the developing flower buds showed the presence of GUS staining. Specifically, we detected the GUS activity in vasculature of petals and styles in mature flower (Supplemental Figure S1D). In addition, it showed that strong GUS activity was detected in vascular tissues of siliques at 1 DAF (days after flowering) and embryo at 15 DAF (Supplemental Figures S1E and F).
It has been known that the Group IV sucrose transporters are tonoplast- or/and PM-localized. Since the tonoplast localization of AtSUC4 was found only by protoplasts transient system [Citation13], we wanted to re-check whether AtSUC4 localized to the PM using three different approaches. The AtSUC4-GFP fusions protein was stably expressed in the root of Arabidopsis, showing clear and strong fluorescence in the PM (). Immune colloidal gold assay was also conducted for subcellular localization detection. The identification of IgG-type positive hybridoma cell lines by Western Blot are shown in Supplemental Figure S2, and a monoclonal antibody of AtSUC4, which belongs to subclass G2b, was successfully obtained. Both immune colloidal gold assay and transient transformation of Arabidopsis protoplasts with 35S::AtSUC4-GFP vector showed that AtSUC4 was localized to the PM (Supplemental Figures 2B and C). These results indicated that AtSUC4 was mainly located in the PM in root cells.
Figure 2. Subcellular localization of AtSUC4. (A) Root image of GFP fusion to the C-terminus of AtSUC4 by stable transformation. (B) Detection of AtSUC4 localization by immune colloidal gold. SE: sieve element, CC: companion cell, PM: plasma membrane. The black arrow represents the gold particles. The right image served as a negative control. (C) The subcellular localization of a GFP fusion to the C-terminus of AtSUC4 by transient transformation of Arabidopsis protoplasts. GFP and RFP indicated the fluorescence of AtSUC4-GFP fusion protein and chlorophyll auto-fluorescence, respectively.
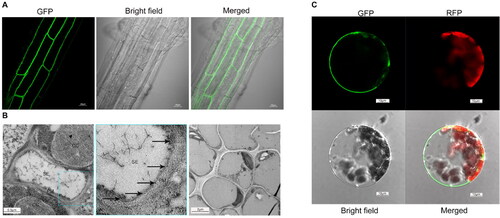
Analysis of root length in atsuc4 mutants under higher sucrose condition
In order to determine the effect of AtSUC4 mutation on plant growth, three mutant lines were generated, including suc4-1 (generated by EMS mutagenesis), suc4-2 (generated by CRISPR/Cas9 editing) and suc4-3 (T-DNA insertion) (Supplemental Figure S3A). The suc4-1 point mutation (C502A) and suc4-2 inserted mutation (A was inserted between nucleotides 29 and 30) introduced stop codon (TAA), resulting an early termination of AtSUC4 protein translation (Supplemental Figure S3B). The AtSUC4 transcript in all the three mutants was significantly lower than in the WT, whereas it was similar to that of the WT in the AtSUC4/suc4-1 (Supplemental Figures S3C and D). Under the LD conditions, both plant height and leaf number were similar in all genotypes (Supplemental Figures S3E and F, S4A and B). The root length of WT was similar to all the three atsuc4 mutants on medium supplemented with low sucrose concentration, including 0%, 0.5%, 1%, 1.5% or 2% sucrose (Supplemental Figure S4C). These data demonstrated that there was no difference in phenotype between atsuc4 mutants and WT plants on the sucrose-free or low concentration of sucrose condition.
Figure 3. The phenotypic and statistics of primary root length under different concentrations (0%, 2%, 4% and 6%) of sugar treatments: sucrose (A, B); glucose (C, D); mannitol (E, F). The first row plants in (A), (C) and (E) are the shared control group. Data in (B), (D) and (F) are mean values ± SE of three biological replicates. All the plants were grown on MS solid media for 6 days after sowing. Bars with different letters indicate significant differences among genotypes for the same treatment according to Duncan’s test (at p < 0.05), n > 50.
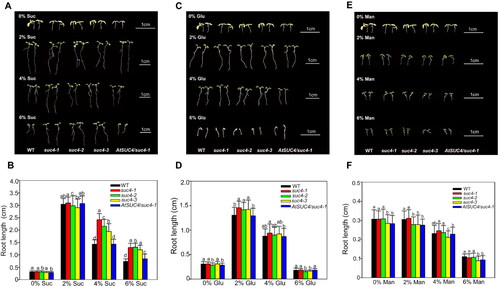
Figure 4. Expression level of genes related to sucrose transport and metabolism, IAA and ABA signalling pathway. (A), (C), (E) Heatmap of representative genes expression related to sucrose, IAA and ABA signalling pathway. (B), (D), (F) Gene expression changes as assessed by RT-qPCR. The relative expression detected by RT-qPCR is represented by a bar graph, and the left ordinate indicates the expression level. FPKM of genes, according to RNA-Seq data, is represented by a line graph, and the right ordinate indicates the FPKM level. Asterisks represent a significant difference compared with WT. The log2 fold change value of the gene expressions from transcriptome is displayed in different colours. Yellow colour means high expression and blue colour means low expression. Each sample was assayed in three biological replicates. The experimental materials were selected from the roots of different plants which were grown on MS solid media with 6% sucrose for 6 days.
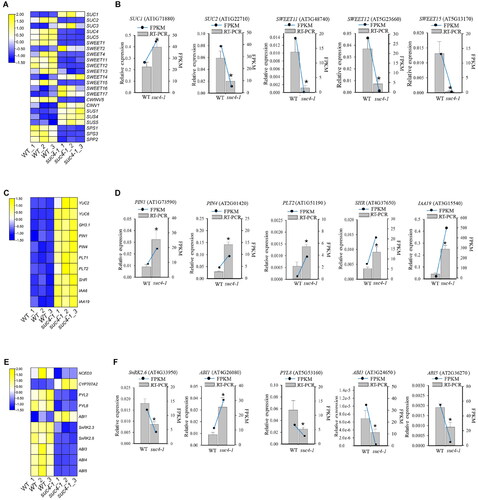
To test whether the phenotypes of atsuc4 mutants were altered in response to high content of sucrose, atsuc4 mutants, WT and the complementary line AtSUC4/suc4-1 were cultured under different concentrations (0%, 2%, 4% and 6%) of three different sugars. When feeding sucrose to the medium, the root length was the longest in all plants at 2% sucrose concentration. As the sucrose concentration became higher, the root length in all lines gradually decreased (), indicating that higher concentration of sucrose would inhibit the root growth. Interestingly, the primary root of the atsuc4 mutants was significantly longer than that of WT and AtSUC4/suc4-1 plants when the sucrose concentrations in the medium was raised to 4% and 6% (). We next explored whether the high concentration of glucose or mannitol (served as osmotic stress) would cause the similar difference of primary root length among WT, atsuc4 mutants and AtSUC4/suc4-1. As shown in , no differences were found in the root length among these three genotypes of plants under the same treatment. Taken together, these results suggested that disruption of AtSUC4 in atsuc4 mutants repressed the inhibition of high concentration sucrose on root growth, which displayed a sucrose-specific response.
Accumulation of sucrose, ABA and IAA in roots of atsuc4 mutants under higher sucrose condition
To investigate whether the difference in root length was caused by the sucrose content, we then compared the sucrose content in roots between mutants and WT. The results revealed that when no exogenous sucrose was added, the sucrose content in all lines showed no significant difference. After adding exogenous sucrose (2%, 4% and 6%), the sucrose content in the roots of all lines increased as the exogenous sucrose concentration became higher. However, an obviously reduced sucrose content in root was shown in atsuc4 mutants compared with WT and AtSUC4/suc4-1 on medium with 2%, 4% and 6% sucrose supplementation, especially when 6% sucrose was added, the difference of sucrose content between atsuc4 mutants and WT was conspicuous (). To a lesser extent, the glucose content of suc4-1 and suc4-3 roots were decreased compared with WT on medium supplemented with 4% and 6% sucrose, whereas no significant difference between suc4-2 and WT (Supplemental Figure S5A). The content of fructose in three atsuc4 mutants was significantly lower than that of WT and AtSUC4/suc4-1 on medium without sucrose. As the concentration of sucrose increased to 6%, markedly reduced fructose content was detected in suc4-2 compared with WT, but no significant differences in WT, suc4-1 and suc4-3 (Supplemental Figure S5B). These results showed that atsuc4 mutants accumulated less sucrose under higher sucrose condition, which was probably contributed to longer root length compared with WT.
Table 1. Sucrose content in roots of different types of plants.
All the plants were grown on MS solid media with different concentration of sucrose (0%, 2%, 4% and 6%) for 6 days after sowing. Data are mean values ± SD of three biological replicates. ‘*’ and ‘**’ indicate significant differences among genotypes for the same treatments according to Duncan’s test (at p < 0.05 and p < 0.01, respectively), n > 50.
In order to verify whether the longer root length of the atsuc4 mutants crossed with the IAA and ABA signal pathway under high sucrose condition, we detected the IAA and ABA contents in roots of WT and suc4-1 (). The result showed that the accumulation of IAA in both suc4-1 and WT was decreased in the 6% sucrose supplementation compared with 2% sucrose. However, it was significantly higher in suc4-1 than WT, suggesting that the less reduction of IAA might alleviate the inhibition of root growth under high sucrose. On the contrary, 6% sucrose stimulated the accumulation of ABA in both suc4-1 and WT, and less ABA was accumulated in the suc4-1 compared with WT.
Table 2. ABA and IAA content in roots of different plants.
All the plants were grown on MS solid media with different concentration of sucrose (2% and 6%) for 6 days after sowing. Data are mean values ± SD of three biological replicates. ‘*’ indicates significant differences among genotypes for the same treatments according to Duncan’s test (at p < 0.05), n > 50.
Analysis of global gene expression change in atsuc4 mutants under different sucrose condition
To better understand the role of AtSUC4 at the molecular level under high sucrose, comparative transcriptome analysis was performed between suc4-1 and WT using roots grown for 6 d under 2%, 4% and 6% sucrose treatments. A total of 1,259 (581 up, 678 down) DEGs were identified in suc4-1 compared with WT on the medium supplemented with 2% sucrose, respectively. At 4% sucrose condition, 452 (182 up, 270 down) DEGs were found in suc4-1. Under high concentration of sucrose condition (6% sucrose), the number of DEGs increased, among which 6,485 (2,837 up, 3,648 down) DEGs were identified in suc4-1. Besides, only 26 DEGs overlapped under these three conditions (Supplemental Figure S6). KEGG enrichment analysis among the DEGs revealed that three pathways, including carbon metabolism, glyoxylate and dicarboxylate metabolism and pyruvate metabolism, were enriched under 4% sucrose treatment (Supplemental Figure S7A). Additionally, plant hormone signal transduction, starch and sucrose metabolism and phenylpropanoid biosynthesis were the most enriched pathways in the suc4-1 plants at 6% sucrose condition (Supplemental Figure S7B).
Atsuc4 mutants response to higher sucrose through sucrose, IAA and ABA signalling pathway
Our study showed that the sucrose accumulation was much lower in atsuc4 mutants than in WT under 6% sucrose. We therefore checked the expression change of AtSUCs and AtSWEETs, two main kinds of carriers for sucrose transport, in suc4-1 in RNA-seq data. As shown in , AtSUC2, 4 and 5, AtSWEET1, 4, 11, 12, 13 and 15 were significantly down-regulated in suc4-1 compared with WT at 6% sucrose condition, whereas a reverse expression pattern was found for AtSWEET2, 14, 16 and 17, as well as AtSUC1 and 3. We further investigated the expression of AtSUC2, AtSWEET11, 12 and 15 by qRT-PCR, showing consistence with RNA-seq data (). These results revealed that more sucrose transporters tend to be down-regulated in suc4-1, which might contribute to lower level of sucrose in the mutants under high sucrose. Except for sucrose transport, sucrose accumulation is also affected by sucrose synthesis and degradation. Hence, we explored the expression of genes related to sucrose synthesis and degradation in suc4-1 under high sucrose conditions. As shown in , three genes that encode Sucrose Synthase (SUS1, 4, 5), which are important for sucrose degradation, were significantly up-regulated in suc4-1 lines compared with WT under high sucrose. On the contrary, the transcript levels of the genes encoding Sucrose-Phosphate Synthase (SPS), which is involved in sucrose synthesis, were reduced significantly.
The content of ABA and IAA were significantly different between suc4-1 and WT under 6% sucrose treatment (). Therefore, the expression levels of IAA- and ABA-related genes were investigated by RNA-seq. As shown in , two IAA biosynthesis genes (YUC2, 6), two IAA transporter genes (PIN1, 4) and five IAA signal transduction genes (PLT1, 2, SHR, IAA6 and 19) were up-regulated in suc4-1 under 6% sucrose condition. On the contrary, one ABA biosynthesis gene (NCED3), seven ABA signal transduction genes (PYL2, 8, SnRK2.3, 2.6, ABI3, 4 and 5) were down-regulated in suc4-1 (). These results were in agreement with the increased accumulation of IAA and reduction in ABA in atsuc4 mutant plants under 6% sucrose treatment. To test the reliability of the transcriptome data, five sucrose metabolism genes, five IAA signalling pathway genes and five ABA signalling pathway genes were chosen for RT-qPCR assay. Gene expression as elucidated by RT-qPCR exhibited similar trends with that of the transcriptome data, with some variation in the magnitude (). Taken together, these results suggested that deficiency of AtSUC4 had directly or indirectly stimulated the IAA signalling pathway but repressed the ABA signalling pathway under high sucrose.
Discussion
Although various studies have focussed on AtSUCs in Arabidopsis, relatively few have been performed on the effect of AtSUC4, a unique high-affinity/low-capacity sucrose transporter in Group IV in Arabidopsis, on root development in recent years. Based on our findings in this study, the possible function and the regulation mechanism of AtSUC4 in the root under high sucrose would be discussed.
Long-distance transport of sucrose from source to sink organs occurs through vascular system, which is mediated mainly by sucrose transporters. In this regard, many SUTs were targeted to PM [Citation33]. It is well known that the members in the SUT4 clade were reported to be either PM- or/and tonoplast-localized [Citation34,Citation35]. To date, dual target to the PM and tonoplast have been characterized in several members, including HvSUT2 (Hordeum vulgare), LjSUT4 (Lotus japonicas), NtSUT4 (Nicotiana tabacum), GeSUT4 and OsSUT2 (Oryza sativa L.) [Citation36,Citation37]. In addition, two SUT4 homologs of Solanaceae, SlSUT4 from tomato and StSUT4 from potato, were found in the endoplasmic reticulum except for PM and vacuole [Citation36], indicating heterogeneity of SUT4 clade localization in plants. It has been characterized that AtSUC4 localized to vacuole using the protoplast transient expression system and PM by functional characterization in heterogenous yeast [Citation13, Citation34]. Only one proteomic report indicated that AtSUC4 residue was detectable in the chloroplast envelope [Citation38], but this point of view was fully challenged by lack of more direct evidence [Citation39]. In the present study, using stably transformed plants with an AtSUC4-GFP construct under a native promoter, we demonstrated that AtSUC4 was PM-localized, which was confirmed by two other additional approaches of transient expression and Immunogold label systems (). Moreover, the deficiency of AtSUC4 led to less sucrose accumulation in the root of atsuc4 mutants under high sucrose concentration (), implying the sucrose import mediated by AtSUC4 from the extracellular space to the cytoplasm. These observations provided clear evidence that AtSUC4 physiologically functions at the PM in root, the primary site for apoplastic sucrose import. Therefore, the alleviation of sucrose toxicity in root under high sucrose treatment in atsuc4 mutant was supported by the sucrose import activity of AtSUC4.
Sucrose metabolism plays pivotal roles in development and stress response, mainly by generating a range of sugars as metabolites to fuel growth and as signals to regulate gene expression for crosstalk with hormonal signalling. This is achieved by the generation of sugar signalling molecules such as sucrose itself, glucose and trehalose-6-phosphate or perhaps by the signalling role exerted by the metabolic process itself [Citation40,Citation41]. Therefore, sucrose transport, synthesis and degradation are very important in regulating plant growth. To date, SUCs and SWEETs have been characterized as the two main types of proteins responsible for sucrose transport in plants. Arabidopsis genome contains nine SUCs, and five of them were found to be differentially expressed between the atsuc4 mutant and WT under 6% sucrose supplementation, which AtSUC2, 4 and 5 were down-regulated, whereas AtSUC1 and 3 were up-regulated in suc4-1 (), suggesting that more AtSUCs were decreased in suc4-1. The clade III member of SWEET family in Arabidopsis AtSWEET9-15 and one member of clade V AtSWEET16 have been identified to have sucrose transport activity [Citation3, Citation42]. As SWEETs facilitate the sugar transport along the sugar gradient, 6% sucrose treatment inhibited the expression of AtSWEET11, 12 and 15, but only induced AtSWEET14 and 16 expression in suc4-1 (), indicating that a larger number of AtSWEETs which was responsible for sucrose transport tend to be repressed in suc4-1. Accordingly, we proposed that the notably decreased sucrose accumulation in the atsuc4 mutants could be a result of the more down-regulated expression of AtSUCs and AtSWEETs, which are responsible for sucrose transport. It was well known that AtSUC2, functions as a high-affinity, low-capacity transporter, is essential for phloem loading for long-distance sucrose transport from source to sink [Citation43]. The expression of AtSUC2 was decreased in atsuc4 mutant (), and was induced by increasing sucrose treatment (Supplemental Figure S8), showing the co-expression pattern of AtSUC2 and AtSUC4 under high sucrose condition. Furthermore, the interaction between AtSUC2 and AtSUC4 was found [Citation43], and overexpression of AtSUC4 and inclusion of adg1, tmt1, tmt2 in atsuc2 mutant could partially rescue the dwarf phenotype [Citation44]. Accordingly, probably AtSUC2 and AtSUC4 might be in a complex network to regulate root growth under high sucrose condition. Computational analysis of cis-acting regulatory elements in promoters of AtSUCs showed that ABA-responsive element (ABRE) was distributed in most of AtSUCs, including AtSUC1 and AtSUC3 [Citation45]. Furthermore, a bZIP transcriptional factor ABI5, involved in the ABA signalling pathway, was found to bind to ABRE in the context of the AtSUC1 promoter using yeast-one hybrid assay, and negatively regulating the AtSUC1 expression level [Citation46]. In the present study, ABI5 was repressed in the atsuc4 mutant compared with WT under 6% sucrose treatment (), which thus contributes to the promotion of AtSUC1 accumulation. Although there is no direct evidence that ABI5 could bind to ABRE in the AtSUC3 promoter, we speculated that ABI5 might affect the AtSUC3 expression in a similar way to that in AtSUC1.
Sucrose can be degraded by sucrose synthase (SUS; EC 2.4.1.13) into hexose or their derivatives, which are then used in diverse ways. The transcript levels of AtSUS1, 4 and 5 and AtCINV1 were increased, whereas sucrose synthesis-related genes, including two AtSPSs (AtSPS1 and AtSPS3) and AtSPP2, were decreased in the suc4-1 plants compared with WT (), indicating that the pathway of sucrose degradation was active, but the sucrose synthesis was blocked by the deficiency of AtSUC4. Taken together, these data suggest that AtSUC4 regulates root growth by affecting the expression of many sucrose metabolic enzymes, which reduce sucrose inflow and biosynthesis and increase sucrose degradation in intracellular.
Evidence from some studies highlights the central role of auxin in generation and maintenance of primary root meristems [Citation17, Citation47,Citation48]. Thus, sucrose and auxin could operate coordinately during root growth. Interestingly, the root length and IAA content of atsuc4 mutants were higher than that of WT under 6% sucrose treatment ( and ). Consistent with this, RNA-seq data showed that the expression of the genes related to IAA biosynthesis (YUC2, 6) and transporters (PIN1, 4) were significantly up-regulated in suc4-1 under high-sucrose (). Since lower sucrose content was measured in atsuc4 mutants, and sucrose has been identified as a signal molecule, it is therefore speculated that more sucrose could active expression of genes responsible for IAA biosynthesis and transport. The PLT, SCR and SHR genes are required to define the root–stem cell niche [Citation49,Citation50]. Meanwhile, PLT in turn regulates the auxin accumulation in the quiescent centre both through transport (PIN4) and biosynthesis (YUCs) [Citation51,Citation52]. According to RNA-seq data, AtPLT1, 2 and AtSHR were significantly up-regulated in suc4-1 compared with WT at 6% sucrose application (). Based on the results presented here, we proposed that the higher content of IAA provoked the expression of AtPLT1, 2 and AtSHR in suc4-1 compared with WT, which contribute to display the longer primary root under high sucrose. The aux/iaa mutants, including iaa1, 2, 13, 6, 17, 19 and 28, had reduced multiple auxin responses, with shorter roots than WT [Citation53]. In our study, the expression levels of two Aux/IAAs (IAA6, 19) in the atsuc4 mutant were significantly higher than in the WT in the treatment with 6% sucrose (), contributing to the longer root length in the atsuc4 mutant accordingly. The interaction between sucrose and IAA has been revealed, in which auxin signalling factor significantly affected the accumulation of sucrose, while sucrose could regulate the auxin signalling [Citation54,Citation55]. Our results indicated that AtSUC4 influenced the expression of IAA-related genes, and we tested whether IAA has an impact on AtSUC4 accumulation in turn. However, the result showed that no obvious change occurred in GUS activity under native AtSUC4 promoter and AtSUC4 expression under IAA treatment (Figure S9A and B), indicating that AtSUC4 expression was not affected by IAA during seedling growth. It has been reported that sucrose not only provides the energy for plant growth and development, but also acts as a signal factor to regulate gene expression [Citation56]. Hence, the RNA profiling studies revealed that the deficiency of AtSUC4 decreased the sucrose content in atsuc4 mutants root compared with WT under 6% sucrose treatment, with sucrose acting as a signal factor to increase the expression of IAA-related genes, therefore activated the IAA signalling transduction.
Studies have suggested that sucrose and ABA are provital molecular signals that participate in regulating diverse developmental processes in plant [Citation57,Citation58]. It has been reported that manipulation of strawberry FaSUT1 expression is positively correlated with sucrose and ABA content [Citation57]. Similarly, as shown in and Supplemental Figure S5, the deficiency of AtSUC4 led to a strong decrease in sucrose content relative to WT under all the concentrations of exogenous sucrose, but had little effects on the content of glucose and fructose. Moreover, suc4-1 had significantly lower of ABA content under both low (2%) and high (6%) sucrose concentrations, suggesting positive relationship between AtSUC4 expression and ABA synthesis. In addition, NCED3, a key gene that controls the synthesis of ABA, was significantly decreased in suc4-1 compared with WT (). These results strongly indicated that AtSUC4 probably participates in the sucrose signalling, subsequently regulating the ABA synthesis in the root. ABA signal transduction is highly dependent on its synthesis [Citation59,Citation60]. Consistent with the less ABA in suc4-1, we found that the expression levels of seven ABA signal transduction genes (PYL2, 8, SnRK2.3, 2.6, ABI3, 4, 5) were significantly decreased in suc4-1 with 6% sucrose supplementation, while ABI1, a member of group PP2C which has a negative regulatory effect on ABA signal transduction, was significantly up-regulated (). Similar to IAA, ABA could not affect the expression of AtSUC4 during seedling growth (Figure S9C and D), suggesting that AtSUC4 accumulation could not be regulated by ABA. Collectively, our data may indicate that the deficiency of AtSUC4 reduced the inhibition of root elongation via ABA through repressing the ABA synthesis and signalling mediated by reducing sucrose content.
Conclusions
This study demonstrated a novel role of AtSUC4 involved in high sucrose mediated-inhabitation in root growth. We found that AtSUC4 was induced by sucrose, and the deficiency of AtSUC4 resulted in alleviation of sucrose toxicity in root under high sucrose condition, which might be ascribed to reduced sucrose and ABA accumulation and increased IAA levels. The gene expression of sucrose-related, ABA and IAA signalling pathways were altered in suc4-1 mutant plants under high sucrose. These results provide new insights into the mechanism in which AtSUC4 regulates sucrose translocation when the extracellular sucrose concentration is high. However, how AtSUC4 coordinates with sucrose, IAA and ABA pathways remains to be elucidated so that we can fully understand the AtSUC4-mediated modulation of root growth under high sucrose condition.
Authors’ contributions
Conceptualization, J.L. and C.P.; Formal analysis, S.L., L.Z., J.G. and T.D.; Investigation, S.L., L.Z., J.G. T.D. and Y.W.; Methodology, S.L.; Writing – original draft, S.L. and J.L.; Writing – review & editing, J.L. and C.P.
Supplemental Material
Download MS Excel (14.7 KB)Supplemental Material
Download PDF (1.1 MB)Disclosure statement
No potential conflict of interest was reported by the authors.
Data availability statement
The data that support the findings of this study are openly available in [National Center for Biotechnology Information Sequence Read Archive] at [https://www.ncbi.nlm.nih.gov/sra/], reference number [PRJNA666009].
Additional information
Funding
References
- Ruan YL, Jin Y, Yang YJ, et al. Sugar input, metabolism, and signaling mediated by invertase: roles in development, yield potential, and response to drought and heat. Mol Plant. 2010;3(6):942–955.
- Wormit A, Trentmann O, Feifer I, et al. Molecular identification and physiological characterization of a novel monosaccharide transporter from Arabidopsis involved in vacuolar sugar transport. Plant Cell. 2006;18(12):3476–3490.
- Braun DM, Wang L, Ruan YL. Understanding and manipulating sucrose phloem loading, unloading, metabolism, and signalling to enhance crop yield and food security. J Exp Bot. 2014;65(7):1713–1735.
- Lohaus G, Hussmann M, Pennewiss K, et al. Solute balance of a maize (Zea mays L.) source leaf as affected by salt treatment with special emphasis on phloem retranslocation and ion leaching. J. Exp. Bot. 2000;5:1721–1732.
- Geiger D. Plant sucrose transporters from a biophysical point of view. Mol Plant. 2011;4(3):395–406.
- Julius BT, Leach KA, Tran TM, et al. Sugar transporters in plants: new insights and discoveries. Plant Cell Physiol. 2017;58(9):1442–1460.
- Kühn C. A comparison of the sucrose transporter systems of different plant species. Plant Biol. 2003;5(3):215–232.
- Sauer N. Molecular physiology of higher plant sucrose transporters. FEBS Lett. 2007;581(12):2309–2317.
- Eom JS, Cho JI, Reinders A, et al. Impaired function of the tonoplast-localized sucrose transporter in rice, OsSUT2, limits the transport of vacuolar reserve sucrose and affects plant growth. Plant Physiol. 2011;157(1):109–119.
- Sivitz AB, Reinders A, Ward JM. Arabidopsis sucrose transporter AtSUC1 is important for pollen germination and sucrose-induced anthocyanin accumulation. Plant Physiol. 2008;147(1):92–100.
- Gottwald JR, Krysan PJ, Young JC, et al. Genetic evidence for the in planta role of phloem-specific plasma membrane sucrose transporters. Proc Natl Acad Sci U S A. 2000;97(25):13979–13984.
- Schulz A, Beyhl D, Marten I, et al. Proton-driven sucrose symport and antiport are provided by the vacuolar transporters SUC4 and TMT1/2. Plant J. 2011;68(1):129–136.
- Schneider S, Hulpke S, Schulz A, et al. Vacuoles release sucrose via tonoplast-localised SUC4-type transporters. Plant Biol (Stuttg). 2012;14(2):325–336.
- Freixes S, Thibaud M-C, Tardieu F, et al. Root elongation and branching is related to local hexose concentration in Arabidopsis thaliana seedlings. Plant Cell Environ. 2002;25(10):1357–1366.
- Kircher S, Schopfer P. Photosynthetic sucrose acts as cotyledon-derived long-distance signal to control root growth during early seedling development in Arabidopsis. Proc Natl Acad Sci U S A. 2012;109(28):11217–11221.
- Durand M, Mainson D, Porcheron B, et al. Carbon source-sink relationship in Arabidopsis thaliana: the role of sucrose transporters. Planta. 2018;247(3):587–611.
- Hoth S, Niedermeier M, Feuerstein A, et al. An ABA-responsive element in the AtSUC1 promoter is involved in the regulation of AtSUC1 expression. Planta. 2010;232(4):911–923.
- Chaiwanon J, Wang W, Zhu JY, et al. Information integration and communication in plant growth regulation. Cell. 2016;164(6):1257–1268.
- Macgregor DR, Deak KI, Ingram PA, et al. Root system architecture in Arabidopsis grown in culture is regulated by sucrose uptake in the aerial tissues. Plant Cell. 2008;20(10):2643–2660.
- Zhang S, Peng F, Xiao Y, et al. Peach PpSnRK1 participates in sucrose-mediated root growth through auxin signaling. Front Plant Sci. 2020;11:409.
- Antoni R, Gonzalez-Guzman M, Rodriguez L, et al. PYRABACTIN RESISTANCE1-LIKE8 plays an important role for the regulation of abscisic acid signaling in root. Plant Physiol. 2013;161(2):931–941.
- Yu B, Wang Y, Zhou H, et al. Genome-wide binding analysis reveals that ANAC060 directly represses sugar-induced transcription of ABI5 in Arabidopsis. Plant J. 2020;103(3):965–979.
- Yuan TT, Xu HH, Zhang KX, et al. Glucose inhibits root meristem growth via ABA INSENSITIVE 5, which represses PIN1 accumulation and auxin activity in Arabidopsis. Plant Cell Environ. 2014;37(6):1338–1350.
- Clough SJ, Bent AF. Floral dip: a simplified method for Agrobacterium-mediated transformation of Arabidopsis thaliana. Plant J. 1998;16(6):735–743.
- Schneider S, Beyhl D, Hedrich R, et al. Functional and physiological characterization of Arabidopsis INOSITOL TRANSPORTER1, a novel tonoplast-localized transporter for myo-inositol. Plant Cell. 2008;20(4):1073–1087.
- Robert S, Zouhar J, Carter C, et al. Isolation of intact vacuoles from Arabidopsis rosette leaf-derived protoplasts. Nat Protoc. 2007;2(2):259–262.
- Fan RC, Peng CC, Xu YH, et al. Apple sucrose transporter SUT1 and sorbitol transporter SOT6 interact with cytochrome b5 to regulate their affinity for substrate sugars. Plant Physiol. 2009;150(4):1880–1901.
- Karimi M, Inze D, Depicker A. GATEWAY vectors for Agrobacterium-mediated plant transformation. Trends Plant Sci. 2002;7(5):193–195.
- Ma X, Zhang Q, Zhu Q, et al. A robust CRISPR/Cas9 system for convenient, high-efficiency multiplex genome editing in monocot and dicot plants. Mol Plant. 2015;8(8):1274–1284.
- Jiang SY, Vanitha J, Bai Y, et al. A novel binary T-vector with the GFP reporter gene for promoter characterization. Plos One. 2014;9(9):e107328.
- Chen ML, Fu XM, Liu JQ, et al. Highly sensitive and quantitative profiling of acidic phytohormones using derivatization approach coupled with nano-LC-ESI-Q-TOF-MS analysis. J Chromatogr B Analyt Technol Biomed Life Sci. 2012;905:67–74.
- Deng GM, Zhang S, Yang QS, et al. MaMYB4, an R2R3-MYB repressor transcription factor, negatively regulates the biosynthesis of anthocyanin in banana. Front Plant Sci. 2021;11:600704.
- Kühn C, Grof CP. Sucrose transporters of higher plants. Curr Opin Plant Biol. 2010;13(3):287–297.
- Weise A, Barker L, Kühn C, et al. A new subfamily of sucrose transporters, SUT4, with low affinity/high capacity localized in enucleate sieve elements of plants. Plant Cell. 2000;12(8):1345–1355.
- Reinders A, Sivitz AB, Starker CG, et al. Functional analysis of LjSUT4, a vascular sucrose transporter from Lotus japonicas. Plant Mol Biol. 2008;68(3):289–299.
- Chincinska I, Gier K, Krugel U, et al. Photoperiodic regulation of the sucrose transporter StSUT4 affects the expression of circadian-regulated genes and ethylene production. Front Plant Sci. 2013;4:26.
- Ho LH, Lee YI, Hsieh SY, et al. GeSUT4 mediates sucrose import at the symbiotic interface for carbon allocation of heterotrophic Gastrodia elata (Orchidaceae). Plant Cell Environ. 2020;44(1):20–33.
- Ferro M, Salvi D, Riviere-Rolland H, et al. Integral membrane proteins of the chloroplast envelope: identification and subcellular localization of new transporters. Proc Natl Acad Sci U S A. 2002;99(17):11487–11492.
- Doidy J, Grace E, Kühn C, et al. Sugar transporters in plants and in their interactions with fungi. Trends Plant Sci. 2012;17(7):413–422.
- O’Hara LE, Paul MJ, Wingler A. How do sugars regulate plant growth and development? New insight into the role of trehalose-6-phosphate. Mol Plant. 2013;6(2):261–274.
- Ruan Y, Patrick JW, Bouzayen M, et al. Molecular regulation of seed and fruit set. Trends Plant Sci. 2012;17(11):656–665.
- Chen LQ, Cheung LS, Feng L, et al. Transport of sugars. Annu Rev Biochem. 2015;84:865–894.
- Srivastava AC, Ganesan S, Ismail IO, et al. Functional characterization of the Arabidopsis AtSUC2 sucrose/H + symporter by tissue-specific complementation reveals an essential role in phloem loading but not in long-distance transport. Plant Physiol. 2008;148(1):200–211.
- Schulze WX, Reinders A, Ward J, et al. Interactions between co-expressed Arabidopsis sucrose transporters in the split-ubiquitin system. BMC Biochem. 2003;4(1):3–10.
- Anaokar S, Liu H, Keereetaweep J, et al. Mobilizing vacuolar sugar increases vegetative triacylglycerol accumulation. Front Plant Sci. 2021;12:1664.
- Ibraheem O, Botha CE, Bradley G. In silico analysis of cis-acting regulatory elements in 5′ regulatory regions of sucrose transporter gene families in rice (Oryza sativa Japonica) and Arabidopsis thaliana. Comput Biol Chem. 2010;34(5-6):268–283.
- Dello IR, Nakamura K, Moubayidin L, et al. A genetic framework for the control of cell division and differentiation in the root meristem. Science. 2008;322(5906):1380–1384.
- Overvoorde P, Fukaki H, Beeckman T. Auxin control of root development. Cold Spring Harb Perspect Biol. 2010;2(6):a1537.
- Aida M, Beis D, Heidstra R, et al. The PLETHORA genes mediate patterning of the Arabidopsis root stem cell niche. Cell. 2004;119(1):109–120.
- Moreno-Risueno MA, Sozzani R, Yardimci GG, et al. Transcriptional control of tissue formation throughout root development. Science. 2015;350(6259):426–430.
- Galinha C, Hofhuis H, Luijten M, et al. PLETHORA proteins as dose-dependent master regulators of arabidopsis root development. Nature. 2007;449(7165):1053–1057.
- Santuari L, Sanchez-Perez GF, Luijten M, et al. The PLETHORA gene regulatory network guides growth and cell differentiation in Arabidopsis roots. Plant Cell. 2016;28(12):2937–2951.
- Mockaitis K, Estelle M. Auxin receptors and plant development: a new signaling paradigm. Annu Rev Cell Dev Biol. 2008;24:55–80.
- Yuan Y, Mei L, Wu M, et al. SlARF10, an auxin response factor, is involved in chlorophyll and sugar accumulation during tomato fruit development. J Exp Bot. 2018;69(22):5507–5518.
- Stokes ME, Chattopadhyay A, Wilkins O, et al. Interplay between sucrose and folate modulates auxin signaling in Arabidopsis. Plant Physiol. 2013;162(3):1552–1565.
- Ruan YL. Sucrose metabolism: gateway to diverse carbon use and sugar signaling. Annu Rev Plant Biol. 2014;65(4):33–67.
- Jia H, Wang Y, Sun M, et al. Sucrose functions as a signal involved in the regulation of strawberry fruit development and ripening. New Phytol. 2013;198(2):453–465.
- Yang Z, Zhang L, Diao F, et al. Sucrose regulates elongation of carrot somatic embryo radicles as a signal molecule. Plant Mol Biol. 2004;54(3):441–459.
- Zhu GH, Liu YG, Ye NH, et al. Involvement of the abscisic acid catabolic gene CYP707A2 in the glucose-induced delay in seed germination and post-germination growth of Arabidopsis. Physiol Plant. 2011;143(4):375–384.
- Dong H, Ma XN, Zhang P, et al. Characterization of Arabidopsis thaliana root-related mutants reveals ABA regulation of plant development and drought resistance. J Plant Growth Regul. 2020;39(3):1393–1401.