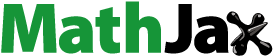
Abstract
Previous studies show that 1-aminocyclopropane-1-carboxylate (ACC) deaminase can facilitate the growth and stress tolerance of hosts by reducing ethylene levels. In this study, the acdS gene encoding ACC deaminase from Bacillus cereus (HK012) was cloned and transformed into tobacco (Nicotiana tabacum L.) by the leaf disc method using Agrobacterium. Molecular detection and physiological analysis of the transgenic tobacco plants were performed. Our results showed the acdS gene was integrated into the tobacco genome and fluorescence microscopy showed that the fusion protein was located on the cell membrane of tobacco root. Compared with control, the transgenic plants showed increases in plant height, root length, dry weight, fresh weight and chlorophyll content; and significant increases in the concentration of proline of 55.15% and 42.7% under salt stress conditions (150 mmol L−1 and 300 mmol L−1 NaCl, respectively). The superoxide dismutase, peroxidase, catalase and ACC deaminase activities of transgenic tobacco were higher than those of control tobacco at 150 and 300 mmol L−1 salt concentrations. Transgenic tobacco seedlings expressing the acdS gene of B. cereus HK012 showed higher salt tolerance than the control plants. The obtained results suggest that the acdS gene of B. cereus can be used to promote salt tolerance in glycophytes by using biotechnology strategies.
Introduction
Salinity is an enormous and growing problem for worldwide agriculture [Citation1]. In particular, hot and dry regions of the world frequently have high salinity soil with low agricultural potential [Citation2]. The physiological consequences of soil salinity are inhibition of plant growth and development, including inhibition of seed germination, flowering and fruit set [Citation3]. The development of crops better adapted to abiotic stresses is crucial in many parts of the world [Citation4]. Transgenic technology has revealed the function of certain heterogenes in crops such as rice, wheat and corn in improving tolerance under biotic and abiotic stress [Citation5–8]. Tobacco (Nicotiana tabacum L.) is a commercial crop and an important model plant of great significance to Chinese farmers and the agricultural industry [Citation9]. In tobacco, high salt stress leads to water loss and loss of enzyme activity, thus hindering seed growth and metabolism and inhibiting tobacco seed germination and reducing the germination rate. Therefore, developing new high-yielding cultivars tolerant to salt stresses will substantially influence tobacco production [Citation10,Citation11].
The biosynthetic pathway of ethylene has been extensively studied [Citation12,Citation13]. As a plant hormone, ethylene modulates normal plant growth and development and acts in the response of plants to various stress factors [Citation14]. Ethylene promotes root hair formation, flowering, fruit ripening and abscission, and leaf and petal senescence and abscission [Citation13,Citation15].
The significance of ethylene as a plant growth regulator has been demonstrated in many crops including Arabidopsis, maize and tomato [Citation16,Citation17]. In the ethylene biosynthesis, methionine is the biosynthetic precursor of ethylene. S-adenosyl methionine (SAM) is synthetized by converting methionine to s-adenosyl l-methionine. Then, 1-aminocyclopropane-1-carboxylate synthase (ACS), the pyridoxal enzyme, converts SAM into 1-aminocyclopropane-1-carboxylate (ACC) and methyl-thioadenosine. ACC oxidase (ACO) finally catalyzes the conversion of ACC to ethylene, HCN, CO2 and water [Citation18,Citation19]. Transduction of the ethylene signal is involved in response to plant adaptation to abiotic stress. Under abiotic stress, root formation, stem and petiole elongation, stomatal opening and closing are regulated by ethylene to reduce salt and metal toxicity [Citation20]. Two ethylene peaks appear in plants after exposure to stress [Citation21]. The first peak is very small and activates the plant’s protective response, and then leads to the second slightly larger ethylene peak, which may lead to plant senescence, yellowing and abscission [Citation22]. Because the second ethylene peak is actually harmful to plants [Citation23], reducing the level of the second ethylene peak by transferring the acdS gene can improve the stress resistance of plants.
ACC is a precursor of ethylene in all higher plants, and both ACC and ethylene typically increase in plants under stresses [Citation24]. Understanding in molecular detail the role of ACC deaminase in ethylene metabolism, its mode of action and regulation is the basis of technological approaches to improve crop abiotic tolerance. ACC deaminase can prevent excessive increase in ethylene biosynthesis by catalyzing the production of α-ketobutyrate and ammonia, thereby reducing the ACC concentration in plants [Citation20,Citation25]. The acdS gene encoding ACC deaminase of a few microorganisms was used for inducing salt tolerance in Arabidopsis, poplar and tomato [Citation26,Citation27]. Thus, transferring acdS into plants can improve their resistance to stress.
Plants can be protected from abiotic stresses such as salt stress, drought stress and heavy metal stress using plant growth-promoting (rhizo)bacteria (PGPB or PGPR), which produce ACC deaminase, or by genetic engineering methods. For example, transgenic rapeseed expressing acdS performed better than non-transgenic plants under salt stress [Citation28]. In addition, the transformation of acdS gene into plants can also be used to enhance plant freshness and reduce the damage of pathogenic microorganisms to plants. Studies show that the inoculation of tomato plants with CHB 1107 wild type (WT) carrying acdS is effective in reducing the production of ethylene and proline content in tomato plants under salt stress, consequently improving their growth and nutrient uptake [Citation29]. In addition, ACC deaminase-containing PGPR can be used as biological control agents against attacking phytopathogens such as Fusarium wilt (Fusarium oxysporum), leaf infection (Botrytis cinerea), bacterial leaf blight (Xanthomonas oryzae), damping off (Rhizoctonia solani), southern blight (Sclerotium rolfsii), root and stem rot (Phytophthora sp), damping off and root rot disease (Pythium ultimum) etc. [Citation30].
AcdS genes were isolated from different strains and plants. The introduction of these genes into commercially important plants by metabolic genetic engineering provides benefits for reducing stress-induced ethylene [Citation31]. In our previous studies, Bacillus cereus HK012 was isolated from halophytic plant seashore mallow (Kosteletzkya virginica (L.)), which is native to the salt marsh in America’s eastern coastal areas. The growth of its host K. virginica seedlings and Arabidopsis plants were improved when inoculated with B. cereus HK012 under salt stress. B. cereus HK012 has the highest activity of ACC deaminase among all the isolated endophytic bacteria. The sequence of acdS gene coding for ACC deaminase HK012 was different from that of other B. cereus strains (all data above unpublished). However, the ability of the acdS gene carried by B. cereus to mitigate salt stress in tobacco has not been well studied. The above findings provide the basis for our current research work. In this study, tobacco plants were genetically modified to express the acdS gene isolated from B. cereus HK012 in K. virginica and were tested for its ability to alleviate salt stress.
Materials and methods
Cloning and sequence analysis of B. cereus acdS
B. cereus (HK012) was isolated from the root tuber of K. virginica, which were introduced from the United States by Nanjing University. It was identified as B. cereus by the combination of morphological characteristics and 16S rDNA sequence. B. cereus (HK012) was inoculated in liquid medium containing 30 ml LB (Luria-Bertani) and incubated for 16–18 h at 30 °C 150 r min−1 to reach an optical density (OD600) of 1.0–1.6, and B. cereus genomic DNA was extracted. The B. cereus acdS gene-specific forward and reverse primers were 5′-cacctttagctaaattcccgag-3′ and 5′-aagatgtattcgcatataaagc-3′, respectively. PCR was conducted using standard conditions consisting of initial denaturation at 94 °C for 4 min followed by 35 cycles of 94 °C for 30 s, 56 °C for 30 s and 72 °C for 2 min, followed by a final extension of 72 °C for 5 min using a Mastercycler® nexus - PCR Thermal Cycler (Eppendorf, Germany). The PCR products of B. cereus acdS were inserted into a T-vector and the resulting construct was transformed into Escherichia coli DH5α competent cells (Thermo Scientific™). Single positive colonies were sequenced by Sangon Biotech (Shanghai) Co., Ltd. and the sequencing results were compared with the acdS gene of B. cereus (AAU17328.1) downloaded from GenBank (https://www.ncbi.nlm.nih.gov/genbank/).
Transgenic plant vector construction and acquisition
To generate the p35S::GFP-acdS construct, the B. cereus acdS gene without a stop codon was cloned by polymerase chain reaction (PCR) to insert into the entry plasmid. The expression vector construction was performed by Gateway technology with the ClonaseTM II kit (Invitrogen, USA) according to the manufacturer’s protocol. Finally, the acdS gene was inserted into the pMDC45 destination vector. The recombinant p2 × 35S::GFP-acdS plasmid was introduced into Agrobacterium tumefaciens GV3101 (Gold Biotechnology®).
Tobacco seeds (cultivar K326, Northrup King Seed Company, USA) were sterilized sequentially with 75% alcohol for 1 min and 10% NaClO for 8 min, then rinsed three times with sterile distilled water. Surface-sterilized seeds were placed on Murashige and Skoog (MS) medium and germinated in an illumination incubator for three to four weeks with a light intensity of 80–200 μmol·m−2·s−1, and the light cycle temperature was 24 °C in the dark for 8 h and 28 °C in the light for 16 h. The tobacco leaves were collected and cut into small pieces (2–4 cm) using a razor blade. Then, A. tumefaciens containing the p35S::GFP-acdS plasmid was transformed into tobacco by the leaf disc technique [Citation32]. The leaf discs were then placed on selective medium and were cultured in the dark at 25 °C. After 30 days, the obtained callus was incubated under 16/8 h light conditions and cultivated until plantlet regeneration. Seeds of transformed tobacco plants were harvested from the transformed plants (T0) and were grown on Murashige and Skoog (MS) medium containing 10 mg L−1 hygromycin and 500 mg L−1 cefotaxime. The hygromycin-tolerant lines were selected for confirmation by PCR. Homozygous T3 progeny derived from T2 population were selected for further verification by western blot and salt tolerance analysis.
Identification of transgenic tobacco plants
T3 transgenic tobacco plants were grown on MS solid medium with hygromycin for six weeks. Then leaves from these plants were collected for genomic DNA extraction using the CTAB method. PCR was performed with GFP gene-specific forward primer (5′-atgatgaaaggagaacttttcactgga-3′, starting at the GFP fragment) and acdS gene-specific reverse primer (5′-aagatgtattcgcatataaagc-3′, starting at the position of termination in the CDS fragment). The amplification program was 94 °C for 2 min, followed by 35 amplification cycles (94 °C for 30 s, 58 °C for 30 s, and 72 °C for 2 min), ending with a 5-minute extension at 72 °C. The PCR products were detected by 1.5% agarose gel electrophoresis.
Western blot analysis of transgenic tobacco plants
After 10 days of culture on MS medium, total protein was extracted from WT and T3 transgenic tobacco seedlings using RIPA Lysis and Extraction Buffer (Thermo Scientific™) and quantified using the BCA Protein Assay Kit. Protein samples (200 μg) were electrophoresed by 8% sodium dodecyl sulfate polyacrylamide gel electrophoresis (SDS-PAGE) and gels were transferred to nitrocellulose membranes. TBST buffer supplemented with 5% skimmed milk was used as a blocking agent. Primary antibodies (anti-GFP antibody, Ab290 (Abcam); GAPDH Monoclonal Antibody, Ab8245(Abcam)) and the secondary antibodies (Goat Anti-Rabbit IgG H&L (HRP): Ab6721 (Abcam); Goat Anti-Mouse IgG H&L (HRP), Ab6789 (Abcam)) were used for western blotting analysis performed on eZwest™ Pro (GeneScript Biotech Co., LTD).
Subcellular localization assay
Transgenic and WT tobacco roots cultured on MS medium for four weeks were used for the preparation of slides. The fluorescence of the ACDS-GFP fusion protein in the roots of 4-week-old transgenic tobacco plants was monitored using confocal laser scanning microscope (ZEISS LSM 880). GFP was visible at 488 nm excitation and emitted between 515 and 565 nm. Imaging of samples was done under bright field and dark field.
Salt stress treatments WT and transgenic tobacco plant
The seeds were cultured under sterile conditions on MS medium containing 10 mg L−1 hygromycin and cultured under light for 25 d. The WT and 35S:: acdS seeds of the same size were transferred to the hydroponic nutrient solution for culture for two weeks and then transferred to the culture solutions with different salt concentrations: 0 mmol L−1 NaCl; 150 mmol L−1; 300 mmol L−1 NaCl. Physiological indicators such as dry weight and fresh weight in the hydroponic nutrient solution were measured after six days.
Determination of chlorophyll content
Leaves (0.1 g) were soaked in 25 mL of 90% ethanol for extraction overnight (16 h). Then, the supernatant was measured at 663 nm and 645 nm with a spectrophotometer (UH5700, HITACHI) [Citation33]. The calculation formula for the chlorophyll content (mg/g) was as follows: chlorophyll (A + B) = 6.63 A665+18.16A649.
Determination of proline content
The powdered tissue was homogenized in 5 mL of 3% aqueous sulfosalicylic acid and centrifuged at 12,000 g for 10 min at 4 °C. The supernatant (2 mL) was collected in a new tube and mixed with 2 mL of acid-ninhydrin and glacial acetic acid, then incubated for 30 min at 100 °C. The cooled mixture was extracted with 4 mL of toluene. Absorbance was measured at 520 nm using toluene as a blank. The proline content (μg g−1) was calculated according to a regression equation [Citation8].
Assay for antioxidant enzymes of transgenic tobacco
Three leaf samples were collected under salt stress treatment, and 0.05 g of each sample was placed into a 2 mL centrifuge tube. They were then quick-frozen in liquid nitrogen for 30 s, shaken vigorously for 20 s, then 1 mL crude enzyme extract was added. The homogenate was centrifuged at 15,000 g for 15 min at 4 °C. The supernatant was used as a crude extract to determine the enzyme activities of superoxide dismutase (SOD), peroxidase (POD) and catalase (CAT). All enzyme assays were performed at 4 °C.
SOD activity
SOD activity was determined based on its effect in inhibiting the photoreduction of nitroblue tetrazolium (NBT) [Citation34]. Upon the addition of riboflavin into a test-tube containing a 3.3-mL reaction mixture (1.5 mL of 50-mmol L−1 phosphate buffer, 0.3 mL of 0.02 mmol L−1 EDTA (pH 7.8), 0.3 mL of 130 mmol L−1 methionine, 0.3 mL of 0.75 mmol L−1 NBT, 0.3 mL of 0.1 mmol L−1 riboflavin, 0.1 mL crude enzyme extract and 0.5 mL ddH2O), the tube was illuminated with two 20-W fluorescent lamps (4000 lux) for 30 min. Non-illuminated and illuminated reactions without the enzyme extract served as calibration standards. The absorbance values of the reaction mixture and the blank control were measured at 560 nm. SOD activity was calculated using the following formula (U g−1):
(1)
(1)
where A0 is the light absorption value of light control, AS is the sample light absorption value, VT is the total sample volume (ml), V1 is the sample dosage during determination (mL) and W is the sample fresh weight (g).
POD activity
POD activity was determined based on the ability of H2O2 to oxidize guaiacol to guaiacinone (amber-coloured product with a maximum absorption peak at 470 nm) in the presence of peroxidase [Citation35]. The reaction mixture (3.55 mL) contained 2.9 mL of 50 mmol L−1 phosphate buffer (pH 7.0), 0.1 mL of 2% guaiacol solution, 0.5 mL of 2% hydrogen peroxide solution and 0.05 mL of enzyme extract. After mixing, the absorbance was measured at 470 nm from the beginning of the reaction to 5 min. Enzyme activity was quantified according to the change in absorbance per minute. Without blank control, the absorbance value of each sample at 470 nm was measured by spectrophotometer (UH5700, HITACHI) at room temperature. The POD activity was calculated according to the following formula (U· g−1 min−1). One unit (1 U) of POD activity was defined as the amount of enzyme that can change A470 by 0.01 per minute.
(2)
(2)
where ΔA470 is the absorbance change value at 470 nm in the reaction solution, W is the fresh weight of leaves (g), t is the reaction time (min), VS is the volume of crude enzyme extract taken during determination (mL) and VT is the total volume of sample (mL).
CAT activity
The CAT activity was measured based on the decrease of H2O2 levels [Citation36]. The reaction mixture (2.55 mL) contained 50 mmol L−1 phosphate buffer (pH 7.0) 2 mL, 0.2% H2O2 0.5 mL and 0.05 mL enzyme extract. The reaction began when the enzyme extract was added to the reaction mixture. The reduction in H2O2 levels was determined by measuring absorbance at 240 nm. One unit of CAT activity was defined as the amount of H2O2 (mmol L−1) degraded per minute. Except for the blank control, the change in the absorbance value at 240 nm of each sample was measured by spectrophotometer (UH5700, HITACHI) at room temperature. A total of 5 recordings were made every 1 min until the light absorption value remained constant. The following formula was used:
(3)
(3)
where E240 is the mean density decrease per minute at 240 nm, VT is the total sample volume (mL), VS is the measured sample volume (mL) and W is the fresh weight of leaves (g).
Determination of ACC deaminase activity
ACC deaminase activity was assayed according to a method that measures the amount of α-ketobutyrate produced upon the hydrolysis of ACC [Citation37]. After the reaction of different concentrations of α-ketobutyric acid with 2,4-dinitrophenylhydrazine, the standard curve of ACC consumption was drawn according to its absorbance value at 570 nm wavelength. After obtaining the crude enzyme extract of transgenic tobacco and WT plants at different salt concentrations and reacting with sufficient amount of ACC, the absorbance value was measured at 540 nm, and the ACC consumption was calculated according to the formula Y = 1.560X–0.0403, R2=0.9914. Under the condition of sufficient substrate reaction time, ACC deaminase activity was positively correlated with substrate ACC consumption, thus reflecting ACC deaminase activity in the sample.
ACC deaminase activity was determined by calculating the α-ketobutyrate concentration of samples according to the standard curve and the following formula.
Data analysis
Data were statistically analyzed using SPSS® (version 22.0). The values are presented as means with standard deviation (± SD). Differences were considered statistically significant at the p < 0.05 and p < 0.01 level.
Results
Molecular characterization of acdS and identification of T3 generation transgenic tobacco plants
Specific forward and reverse primers were used to amplify the full-length B. cereus acdS fragment without a stop codon. Sequencing of the fragment revealed that it is 996 bp in length (), which is similar to that in other microorganisms. Using gateway technology, a pMDC45 destination vector carrying GFP fused with the acdS gene and the hygromycin phosphotransferase (hptII) gene under the regulation of CaMV 2 × 35S promoter was transformed into A. tumefaciens strain GV3101, which were transferred into tobacco by leaf disc technique.
Young leaves of the T3 transgenic tobacco plants and WT seedlings were collected and genomic DNA was extracted. Based on fusion fragment of GFP gene and acdS gene, forward and reverse primers were designed. The integration of acdS gene in different transgenic lines was detected by PCR. Because the GFP and acdS genes corresponded to fragments of 735 bp and 996 bp, respectively, the expected size of the GFP-acdS fusion gene was approximately 1731 bp. The obtained bands of the expected size () indicated that a total of 23 tobacco plants had integrated the GFP-acdS fusion gene in their genomes.
Western blot analysis of transgenic tobacco plant
Western blot analysis of the GFP-AcdS recombinant protein was performed with GFP-specific binding antibody. Six single seedlings from 23 T3 transgenic tobacco lines showed the expected fused protein band with a molecular weight of 63 kDa (). The bands corresponded to the expected band size, indicating that transgenic tobacco plants were successfully obtained.
Subcellular localization of the AcdS protein
As shown (), signals of acdS-GFP were displayed in organelles including in the cell wall or cell membrane. Our result indicated that acdS was predominantly localized in the cell membrane or cell wall.
Overexpression of acdS gene enhances salt tolerance in tobacco
As shown in , under salt stress, transgenic lines with 35S:acdS displayed a salt tolerance phenotype. Transgenic tobacco plants showed a significant increase in plant height, root length and root fresh/dry weight in both non-salt and salt treatments compared with control. Plant height, root length, dry weight and fresh weight of both transgenic and non-transgenic tobacco decreased as the concentration of NaCl in the hydroponic nutrient solution increased. Compared with the control, the transgenic plants showed a better growth advantage, which was most pronounced at 300 mmol L−1 NaCl. This indicates that the introduction of acdS gene of B. cereus into tobacco could improve the salt tolerance of tobacco ().
Chlorophyll content
The chlorophyll content decreased with the increase in NaCl concentration (). However, the chlorophyll content of transgenic tobacco was higher than that of control plants (), under the same concentration of 0 mmol L−1 and 300 mmol L−1 NaCl stress. There was significant difference at 150 mmol L−1 NaCl.
Effect of overexpression of ACC deaminase on proline
Proline is considered to be one of the most effective osmoprotectants because of its circular structure and secondary amino groups, which are different from those of protein-derived amino acids [Citation38]. There is evidence that proline accumulation is positively correlated with stress resistance in plants [Citation39]. In our study, the proline content of transgenic tobacco was significantly increased respectively by 55.15% and 42.27% compared with the control in the variants that received treatment with 150 mmol L−1 and 300 mmol L−1 NaCl ().
SOD, POD and CAT in transgenic tobacco under salt stress
The amount of reactive oxygen species (ROS) in plants increases under salt stress, which leads to relatively inadequate antioxidant scavenging and damage by oxidative stress. Antioxidant systems are responsible for scavenging ROS in plants. Changes in the activity of antioxidant enzymes are important indicators of plant deterioration under environmental stresses [Citation39]. Increased activity of antioxidant enzymes can counteract the oxidative stress induced by salt stress, thus contributing to the ability of plants to tolerate salt. Our results showed that the baseline activities of SOD、CAT and POD in transgenic tobacco were significantly higher than those in the controls both in the absence of NaCl and under treatment with 150 mmol L−1 and 300 mmol L−1 NaCl (). Under 150 and 300 mmol L−1 NaCl treatment, the SOD activity of transgenic tobacco was increased by 28.87% and 31.83%, respectively, compared with the control. Overall, the activities of the three assayed enzymes in transgenic tobacco were significantly higher than those in control plants under salt stress.
ACC deaminase activity
The enzyme activity of ACC deaminase leads to the production of α-ketobutyrate and ammonia. It is one of the most effective mechanisms to induce tolerance of plants to salt stress by reducing the ACC level and preventing the excessive increase of ethylene synthesis under salt stress. The ACC consumption of transgenic tobacco was significantly higher than that of control plants (). At 0, 150 and 300 mmol L−1 NaCl, the ACC consumption increased significantly by 8.5%, 15.3% and 58.1%, respectively. The results showed that the ACC deaminase activity of transgenic tobacco was significantly higher than that of wild-type tobacco, indicating successful expression of acdS.
Discussion
Salt stress can adversely influence plant growth and development. Abiotic stress has severe toxic effects on plant growth, including chlorophyll degradation and destruction of membrane fluidity through membrane lipid peroxidation caused by oxidative damage due to excessive ROS [Citation40]. The regulation of ROS homeostasis is crucial for plant stress resistance. Antioxidant enzymes in plants such as SOD, POD and CAT can control excessive ROS and protect plants from damage [Citation41,Citation42]. SOD is the main enzyme that converts O2.- to H2O2, while CAT and POD comprise the second most important antioxidant system that converts H2O2 and other hydroperoxides to water [Citation43,Citation44]. To investigate the different responses between transgenic tobacco and the nontransgenic counterpart, we monitored the changes in some physiological processes associated with accumulation of antioxidant enzymes. The SOD, POD and CAT activity in this study were higher in transgenic tobacco, thus allowing the transgenic plants to combat the accumulation of ROS and thus decrease the oxidative damage (). This study supports previous reports [Citation18–20,Citation24–29] that the integration of ACC deaminase genes reduces ROS production and thus stress ethylene production, thereby reducing oxidative damage.
Some studies have shown that proline accumulation occurs in tomato plants under salt stress [Citation45]. Our study also showed that the proline content of transgenic tobacco increased significantly under salt stress. In addition, the reduction of total chlorophyll in plants is usually an important effect of salt stress [Citation46]. In this study, compared with the control, the total chlorophyll content of transgenic tobacco under salt treatment was significantly higher. These results suggest that transgenic tobacco mitigated (at least in part) the ethylene-mediated growth inhibition. Ethylene, a hormone that exists in all higher plants, is an important regulator of normal growth and development of plants, as well as a key characteristic of plant response to various stresses [Citation14,Citation47,Citation48]. High concentrations of ethylene can cause plant death. In plants, S-adenosine methionine (SAM) can be used to synthesize ethylene by ACC synthase and ACC oxidase [Citation49]. ACC as a precursor of ethylene synthesis in plants, is secreted from plant roots, and then metabolized by endophytes that produce acdS [Citation50], which catalyzes the conversion of ACC to ammonia and α-ketobutyrate, inhibits the production of vegetable ethylene, thereby promoting plant growth [Citation51]. PGPR containing the acdS gene, such as Pseudomonas veronii, Azoarcus sp. and Rahnella aquatilis, can mitigate the harmful effects of flooding (in Sesamum indicum L.) [Citation52], cadmium stress (in rice) [Citation53] and salt stress (in maize) [Citation54]. Introduction of the acdS gene into Petunia hybrida by transgenic approach can improve the tolerance of petunia to cold, drought and salt stress [Citation55]. The molecular mechanism is attributed, at least partly, to the role of acdS in controlling ethylene synthesis in plants. Transgenic plants grow better, have better physiological parameters and are more tolerant than wild-type plants, mainly because the former produce less ethylene than the latter. These results indicate that ethylene production and the expression of the ethylene biosynthesis gene that encodes ACC oxidase (ACO1) may be significantly lower in transgenic plants than in wild-type plants under stress conditions. Therefore, the production of ethylene as well as the expression ethylene-related genes under different salt concentrations needs to be further investigated in this study.
Here, the B. cereus acdS gene was successfully transformed into tobacco and the GFP-acdS fusion protein was detected in the T3 transgenic tobacco lines. The growth and development of seedlings in transgenic tobacco lines was promoted compared with that of control plants at the same concentration of NaCl solutions. The length of shoots and roots in transgenic tobacco lines were significantly improved as compared to wild-type plants under the same treatment conditions ().
The consumption of ACC decreases with the increase in salt concentration, indicating that, when plants are subjected to high salt stress, ACC is oxidized by ACC oxidase to produce ethylene, causing programmed cell death (PCD) in plants [Citation56]. Compared with the controls, in transgenic tobacco, ACC deaminase increased the consumption of substrate ACC, thereby reducing the level of ethylene produced by ACC oxidase and enhancing the salt tolerance of tobacco.
Conclusions
In this study, the acdS gene encoding ACC deaminase from Bacillus cereus (HK012) was cloned and transformed into tobacco successfully by the leaf disc method. The ACC deaminase activity of transgenic tobacco was significantly higher than that of wild-type tobacco. Heterogeneous expression of acdS gene of B. cereus (HK012) in transgenic tobacco lines promoted the activities of protective enzymes, such as POD, SOD and CAT under normal and salt stress conditions, thus enhancing the tolerance to salinity of transgenic plants. Our results suggest that acdS gene of B. cereus (HK012) could be used to promote salt tolerance in glycophytes by using biotechnology strategies.
Authors’ contributions
Yuqi Guo designed the research. Zengyuan Tian, Yange Chen performed most of the experiments. ZengYuan Tian wrote and revised the manuscript. All authors performed data analyses and took part in writing the manuscript.
Research involving human and animal participants
This article does not contain any studies with human participants or animals performed by any of the authors.
Data availability statement
All data that support the findings reported in this study are available from the corresponding author upon reasonable request.
Disclosure statement
No potential conflict of interest was reported by the authors.
Correction Statement
This article has been republished with minor changes. These changes do not impact the academic content of the article.
Additional information
Funding
References
- Glick BR. Bacteria with ACC deaminase can promote plant growth and help to feed the world. Microbiol Res. 2014;169(1):30–39.
- Glick BR, Cheng ZY, Czarny J, et al. Promotion of plant growth by ACC deaminase-producing soil bacteria. Eur J Plant Pathol. 2007;119(3):329–339.
- Sairam RK, Tyagi A. Physiology and molecular biology of salinity stress tolerance in plants. Curr Sci. 2004;86(3):407–421.
- Liu XQ, Liu CY, Guo Q, et al. Mulberry transcription factor MnDREB4A confers tolerance to multiple abiotic stresses in transgenic tobacco. PLoS One. 2015;10(12):e0145619.
- Wang W, Vinocur B, Altman A. Plant responses to drought, salinity and extreme temperatures: towards genetic engineering for stress tolerance. Planta. 2003;218(1):1–14.
- Dunwell JM. Transgenic approaches to crop improvement. J Exp Bot. 2000;51(suppl_1):487–496.
- Oh SJ, Song SI, Kim YS, et al. Arabidopsis CBF3/DREB1A and ABF3 in transgenic rice increased tolerance to abiotic stress without stunting growth. Plant Physiol. 2005;138(1):341–351.
- Zhang PY, Yuan Z, Wei L, et al. Overexpression of ZmPP2C55 positively enhances tolerance to drought stress in transgenic maize plants. Plant Sci. 2022;314:111127.
- Sun H, Sun X, Wang H, et al. Advances in salt tolerance molecular mechanism in tobacco plants. Hereditas. 2020;157(1):5.
- Li J, Chen C, Wei J, et al. SpPKE1, a multiple stress-responsive gene confers salt tolerance in tomato and tobacco. IJMS. 2019;20(10):2478.
- Park HJ, Kim WY, Yun DJ. A role for GIGANTEA: keeping the balance between flowering and salinity stress tolerance. Plant Signal Behav. 2013;8(7):e24820.
- Yang SF, Hoffman NE. Ethylene biosynthesis and its regulation in higher plants. Annu. Rev. Plant. Physiol. 1984;35(1):155–189.
- Yu J, Niu L, Yu J, et al. The involvement of ethylene in calcium-induced adventitious root formation in cucumber under salt stress. IJMS. 2019;20(5):1047.
- Muller M, Munne-Bosch S. Ethylene response factors: a key regulatory hub in hormone and stress signaling. Plant Physiol. 2015;169(1):32–41.
- Alonso JM, Hirayama T, Roman G, et al. EIN2, a bifunctional transducer of ethylene and stress responses in arabidopsis. Science. 1999;284(5423):2148–2152.
- Freitas VS, Miranda R, Costa JH, et al. Ethylene triggers salt tolerance in maize genotypes by modulating polyamine catabolism enzymes associated with H2O2 production. Environ Exp Bot. 2018;145:75–86.
- Xu L, Xiang G, Sun Q, et al. Melatonin enhances salt tolerance by promoting MYB108A-mediated ethylene biosynthesis in grapevines. Hortic Res. 2019;6:114.
- Tao JJ, Chen HW, Ma B, et al. The role of ethylene in plants under salinity stress. Front Plant Sci. 2015;6:1059.
- Lin Z, Zhong S, Grierson D. Recent advances in ethylene research. J Exp Bot. 2009;60(12):3311–3336.
- Orozco-Mosqueda MDC, Glick BR, Santoyo G. ACC deaminase in plant growth-promoting bacteria (PGPB): an efficient mechanism to counter salt stress in crops. Microbiol Res. 2020;235:126439.
- Robison MM, Griffith M, Pauls KP, et al. Dual role for ethylene in susceptibility of tomato to Verticillium wilt. J Phytopathol. 2001;149(7-8):385–388.
- Hackett RM, Ho CW, Lin ZF, et al. Antisense inhibition of the Nr gene restores normal ripening to the tomato never-ripe mutant, consistent with the ethylene receptor-inhibition model. Plant Physiol. 2000;124(3):1079–1086.
- Stearns JC, Glick BR. Transgenic plants with altered ethylene biosynthesis or perception. Biotechnol Adv. 2003;21(3):193–210.
- Orozco-Mosqueda MD, Duan J, Dibernardo M, et al. The production of ACC deaminase and trehalose by the plant growth promoting bacterium Pseudomonas sp. UW4 synergistically protect tomato plants against salt stress. Front Microbiol. 2019;10:1392.
- Misra S, Chauhan PS. ACC deaminase-producing rhizosphere competent bacillus spp. mitigate salt stress and promote Zea mays growth by modulating ethylene metabolism. 3 Biotech. 2020;10(3):119.
- Tak HI, Ahmad F, Babalola OO. Advances in the application of plant growth-promoting rhizobacteria in phytoremediation of heavy metals. Rev Environ Contam Toxicol. 2013;223:33–52.
- Belimov AA, Safronova VI, Sergeyeva TA, et al. Characterization of plant growth promoting rhizobacteria isolated from polluted soils and containing 1-aminocyclopropane-1-carboxylate deaminase. Can J Microbiol. 2001;47(7):642–652.
- Sergeeva E, Shah S, Glick BR. Growth of transgenic canola (brassica napus cv. Westar) expressing a bacterial 1-aminocyclopropane-1-carboxylate (ACC) deaminase gene on high concentrations of salt. World J Microbiol Biotechnol. 2006;22(3):277–282.
- Liu CH, Siew WY, Hung YT, et al. 1-Aminocyclopropane-1-carboxylate (ACC) deaminase gene in Pseudomonas azotoformans is associated with the amelioration of salinity stress in tomato. J Agric Food Chem. 2021;69(3):913–921.
- Barnawal D, Pandey SS, Bharti N, et al. ACC deaminase-containing plant growth-promoting rhizobacteria protect papaver somniferum from downy mildew. J Appl Microbiol. 2017;122(5):1286–1298.
- Naing AH, Maung TT, Chang KK. The ACC deaminase-producing plant growth promoting bacteria (PGPB): influences of bacterial strains and ACC deaminase activities in plant tolerance to abiotic stress. Physiol Plant. 2021;173(4):1992–2012.
- Ahangarzadeh S, Daneshvar MH, Rajabi-Memari H, et al. Cloning, transformation and expression of human interferon alpha2b gene in tobacco plant (Nicotiana tabacum cv. xanthi). Jundishapur J Nat Pharm Prod. 2012;7(3):111–116.
- Zhou SG, Cheng X, Li FF, et al. Overexpression of SlOFP20 in tomato affects plant growth, chlorophyll accumulation, and leaf senescence. Front Plant Sci. 2019;10:1510.
- Beauchamp C, Fridovich I. Superoxide dismutase: improved assays and an assay applicable to acrylamide gels. Anal. Biochem. 1971;44(1):276–287.
- He MT, Tian ZY, Liu QQ, et al. Trichoderma asperellum promotes cadmium accumulation within maize seedlings. Biotechnol Biotechnol Equip. 2021;35(1):1546–1559.
- Wu ZZ, Yang JY, Zhang YX, et al. Growth responses, accumulation, translocation and distribution of vanadium in tobacco and its potential in phytoremediation. Ecotoxicol Environ Saf. 2021;207:111297.
- Kukla M, Płociniczak T, Piotrowska-Seget Z. Diversity of endophytic bacteria in Lolium perenne and their potential to degrade petroleum hydrocarbons and promote plant growth. Chemosphere. 2014;117:40–46.
- Hosseinifard M, Stefaniak S, Ghorbani Javid M, et al. Contribution of exogenous proline to abiotic stresses tolerance in plants: a review. IJMS. 2022;23(9):5186.
- Cao B, Shu L, Li A. Functional characterization of LkERF-B2 for improved salt tolerance ability in Arabidopsis thaliana. 3 Biotech. 2019;9(7):263.
- Nadarajah KK. ROS homeostasis in abiotic stress tolerance in plants. IJMS. 2020;21(15):5208.
- Hossain MA, Bhattacharjee S, Armin SM, et al. Hydrogen peroxide priming modulates abiotic oxidative stress tolerance: insights from ROS detoxification and scavenging. Front Plant Sci. 2015;6:420.
- Huang H, Ullah F, Zhou DX, et al. Mechanisms of ROS regulation of plant development and stress responses. Front Plant Sci. 2019;10:800.
- Gechev TS, Van Breusegem F, Stone JM, et al. Reactive oxygen species as signals that modulate plant stress responses and programmed cell death. Bioessays. 2006;28(11):1091–1101.
- Mittler R. ROS are good. Trends Plant Sci. 2017;22(1):11–19.
- Parvin K, Hasanuzzaman M, Bhuyan M, et al. Comparative physiological and biochemical changes in tomato (Solanum lycopersicum L.) under salt stress and recovery: role of antioxidant defense and glyoxalase systems. Antioxidants. 2019;8(9):350.
- Du YT, Zhao MJ, Wang CT, et al. Identification and characterization of GmMYB118 responses to drought and salt stress. BMC Plant Biol. 2018;18(1):320.
- Li J, Xu H, Liu W, et al. Ethylene inhibits root elongation during alkaline stress through AUXIN1 and associated changes in auxin accumulation. Plant Physiol. 2015;168(4):1777–1791.
- Ribeiro RP, Costa LC, Medina EF, et al. Ethylene coordinates seed germination behavior in response to low soil pH in stylosanthes humilis. Plant Soil. 2018;425(1–2):87–100.
- Bleecker AB, Kende H. Ethylene: a gaseous signal molecule in plants. Annu Rev Cell Dev Biol. 2000;16:1–18.
- Khan AL, Halo BA, Elyassi A, et al. Indole acetic acid and acc deaminase from endophytic bacteria improves the growth of solanum lycopersicum. Electron. J. Biotechnol. 2016;21:58–64.
- Singh RP, Shelke GM, Anil K, et al. Biochemistry and genetics of ACC deaminase: a weapon to "stress ethylene" produced in plants. Front Microbiol. 2015;6:937.
- Ali S, Khan MA, Kim WC. Pseudomonas veronii KJ mitigates flood stress-associated damage in Sesamum indicum L. Appl Biol Chem. 2018;61(5):575–585.
- Fernandez-Llamosas H, Ibero J, Thijs S, et al. Enhancing the rice seedlings growth promotion abilities of Azoarcus sp. CIB by heterologous expression of ACC deaminase to improve performance of plants exposed to cadmium stress. Microorganisms. 2020;8(9):1453.
- Peng J, Wu D, Liang Y, et al. Disruption of acdS gene reduces plant growth promotion activity and maize saline stress resistance by Rahnella aquatilis HX2. J Basic Microbiol. 2019;59(4):402–411.
- Naing AH, Jeong HY, Jung SK, et al. Overexpression of 1-Aminocyclopropane-1-Carboxylic acid deaminase (acdS) gene in Petunia hybrida improves tolerance to abiotic stresses. Front Plant Sci. 2021;12:737490.
- Kacprzyk J, Burke R, Schwarze J, et al. Plant programmed cell death meets auxin signalling. Febs J. 2022;289(7):1731–1745.